Abstract
Bursts of focused ultrasound energy a thousand times more intense than diagnostic ultrasound have become a non-invasive option for treating cancer, from breast to prostate or uterine fibroid, during the last decade. Despite this progress, many issues still need to be addressed. First, the distortions caused by defocusing obstacles, such as the skull or ribs, on the ultrasonic therapeutic beam are still being investigated. Multi-element transducer technology must be used in order to achieve such transcranial or transcostal adaptive focusing. Second, the problem of motion artifacts, a key component in the treatment of abdominal lesions, has been shown significantly to influence the efficacy and treatment time. Though many methods have been proposed for the detection of organ motion, little work has been done to develop a comprehensive solution including motion tracking and feedback correction in real time. This paper is a review of the work achieved by authors in transcranial high-intensity focused ultrasound (HIFU), transcostal HIFU and motion compensated HIFU. For these three issues, the optimal solution can be reached using the same technology of multi-element transducers devices able to work both in transmit and receive modes.
Introduction
During the past decade, high-intensity focused ultrasound (HIFU) has been used in a wide range of therapeutic applications such as prostate, liver and breast cancer. Conventional HIFU systems evaluated in clinical conditions these days rely on the typical use of a spherical, single-element transducer, which focuses the ultrasonic beam with a mm-size heating spot. The heating spot can then be scanned mechanically in order to cure the whole tumor volume. The efficacy and accuracy of such systems is decreased when trying to treat a tumor located behind a bone barrier such as skull bone in the treatment of brain tumors Citation[1], Citation[2] or ribs in the treatment of liver.
However, the treatment of brain tumors using HIFU beams should be possible. The effects of HIFU in human and animal brains have been extensively investigated Citation[3–9], and these studies have shown the feasibility of ultrasound brain therapy and surgery with the skull bone removed. Nevertheless, non-invasive transcranial therapy remains very limited owing to the strong phase aberrations and absorption caused by the skull, as was first shown by Fry and Barger Citation[10]. To compensate for this distortion, Phillips et al. Citation[11] introduced the idea of phased array corrections to ultrasound diagnostic imaging through the skull. In 1996, Thomas and Fink proposed using a time reversal mirror in therapeutic ultrasound Citation[12]. Prior to treatment and taking advantage of the biopsy, which confirms that the tumor is malignant, a hydrophone is positioned near the tumor to record the signals for each element of the therapeutic array. Once the diagnosis has been confirmed and the hydrophone removed, one has to emit the time-reversed signals with amplitude compensation in order to correct for both phase and amplitude aberrations caused by the skull. Thomas and Fink validated this technique experimentally at low amplitude levels, showing a significant improvement of up to 10 dB in focus quality. In 1998, Hynynen et al. applied a similar phase conjugation technique (equivalent to the time reversal process for phase aberration correction of monochromatic signals) with a high-intensity ultrasound system Citation[13].
Recently, the possibility to assessing the acoustic properties of the skull from MRI and CT images raised new hopes for non-invasive brain therapy. Thus, the reliable and minimally invasive procedure described i Citation[12], Citation[13] could be replaced by a totally non-invasive method that uses MRI Citation[14] or CT images Citation[15–17] to predict the aberrations that will be caused by the skull. Hynynen et al. proposed using MRI images Citation[16] for extracting the skull profile, without information on the internal heterogeneities. Then, using a three-layer numerical model composed of water/skull/brain, they pointed out the necessity of taking into account the complex heterogeneities of the skull bone to improve the model. As CT images can give the internal structure of the skull, it is definitely more suited for modeling the ultrasonic properties of the skull Citation[15], Citation[17]. In order to take into account all the information of the CT images, Pernot et al. Citation[15] proposed using numerical simulations based on three-dimensional finite differences of the complete wave equation. By taking into account the internal bone structure (heterogeneities in density, sound speed and ultrasonic absorption coefficient), this finite differences simulation models accurately all the defocusing effects highlighted by White et al. Citation[17]. Thus, it offers a way to correct these effects non-invasively. The ability of this numerical code to predict the experimental wave propagation was fully validated in experiments. The ultrasonic signals resulting from the propagation through human skull bones immersed in a water tank were recorded by echographic arrays and compared with the numerical code results. The defocusing effect of skull bones was perfectly mimicked by the numerical simulation. Thus, the feasibility of an ultrasonic time reversal focusing technique based on the prior acquisition and guidance of CT scans was confirmed in vitro.
In order to steer the beam electronically and heat the whole target spot by spot without moving the therapeutic array, Tanter et al., in 1998, proposed combining the time reversal process with a numerical back-propagation and steering algorithm, and demonstrated experimentally its feasibility Citation[18]. Recently, Clement and Hynynen Citation[19] used a similar steering technique at high power to achieve in vitro necrosis at the geometrical center of the array with a beam steered from an implantable hydrophone located 1 cm away. However, as explained in their article, the beamwidths of the elements were too tight to obtain a good beam focusing outside of the geometrical center and the array only permitted the restoration of the focus with enough power at the geometrical center. They concluded that a significant improvement in the steering range would occur by using much smaller elements. The present study demonstrates in vivo the ability of ultrasound multi-channels systems to deliver a sufficient acoustic energy through the skull bone for a remote and controlled necrosis of brain tumors. The necrosis is achieved with a high spatial accuracy and important steering capabilities.
In order to build an array that can correct for such skull bone aberrations, a number of design considerations such as the number of elements, the aperture and the frequency must be taken into account. These points have been previously discussed by Clement et al. Citation[20–22]. Based on different considerations on the beam-steering capabilities, the Paris team put its efforts to design a new prototype that used the great versatility of single-element technology. In close collaboration with Imasonic (Besançon, France), they have developed a new technology of high-power elements with small diameter that can be distributed on any surface. Such a technology enables complete control of the shape and the size of the array active surface. Moreover, the large bandwidth of this single-element technology enables it to work between 0.7 and 1.1 MHz. All the single elements are embedded in homemade moulds resulting in an easily upgradeable system. The number of elements results from several tradeoffs: the acoustic energy must be distributed over a large area of the skull in order to maximize the so-called antenna gain (ratio between the active surface of the emitting array and the cross section surface of the beam in the focal plane) and consequently avoid undesired skull heating. Nevertheless the size of the array elements should be small compared with the coherence length of the skull bone in order to achieve a good correction of the skull aberrations. Moreover, the steering capabilities of the array and the grating lobes are deeply related to the size and inter-element distance of the transducers. Finally the cost of the system increases with the number of transducers and has to be taken into consideration.
However, an important disadvantage of large phased arrays is the unwanted presence of grating lobes, and the need to reduce the grating lobes has been common to all therapeutic arrays reported to d Citation[23], Citation[24]. Goss et al. Citation[25], Gavrilov and Hand Citation[26], have shown theoretically that the use of elements randomly distributed on a segment of a spherical surface may improve the focusing capabilities of a phased array compared with more conventional distributions (hexagonal and annular distributions): the near-field beam amplitude and the sidelobes level are lowered. The improvement of the focusing quality becomes particularly important when the focus is steered electronically in the vicinity of the geometrical center as shown by simulations in Citation[35].
Beyond this transcranial application, the similar problem of HIFU transcostal focusing can benefit from the research achieved in the field of non-invasive brain HIFU. It is well known that the treatment of liver cancer is made a challenging task as the target is partially shadowed by the rib cage Citation[27–29]. On one hand, the rib cage acts as an aberrator that might affect the focusing Citation[29]. On the other hand, as a result of the high value of the absorption coefficient of the bone, the overheating on the ribs may be quite significant and must be avoided Citation[27], Citation[29].
The treatment of a large tumor then requires mechanical or electronic scanning in order to focus over the whole region of interest, interleaving the sonications with cooling periods to avoid near-field heating. However, accurate targeting of human abdominal tumors is difficult to maintain during such treatments that can last several hours Citation[30]. The treatment conformability and efficacy can be greatly decreased by human motions, such as breathing Citation[31]. Recently, Allen et al. also reported some cases of mistargeting in HIFU treatment of liver metastases Citation[32].
Motion-tracking techniques have been widely investigated in medical applications such as radiotherapy or 3D imaging (MRI and CT). Respiratory gating is a conventional technique for addressing the problem of breathing motions in radiation therapy Citation[33]. External sensors (spirometer, strain gauge or infrared laser sensors on the patient's skin) are monitored during the treatment, and the therapeutic beam is switched off whenever the target is outside a predefined window. Internal metallic markers have been recently used in respiration gated radiotherapy Citation[34] to determine more accurately the 3D position of the tumor in real-time. But most of the commercial systems are currently limited to the measurement of the displacement amplitude and act as alarms preventing a critical motion; in other words, no feedback is introduced to correct in real time the therapeutic or imaging system.
In 2003, Pernot et al. proposed the first alternative ultrasound-based method for tracking and correcting in real time the 3D motion of tissues during HIFU therapy Citation[35]. This technique is based on tracking the temporal shifts in the backscattered RF signals (i.e. speckle) due to the displacement of the tissues. Speckle tracking techniques were widely investigated in diagnosis ultrasound Citation[36], Citation[37] and were successfully applied to various 2D motion estimation problems Citation[38], Citation[39]. In optics, a similar tracking method based on tracking laser speckle has been developed for estimating the motion of a surface Citation[40] and it is now used in ‘pad-less optical mouse’, a common input device for PCs. To our knowledge, the technique proposed and validated by Pernot et al. is to date the only alternative for getting rid of motion artifacts in an optimal way in terms of acoustic energy deposition and treatment duration.
Non-invasive transcranial focusing for the HIFU treatment of brain tumors
The Paris group (Laboratoire Ondes et Acoustique) developed in the last decade a 300-element HIFU array for skull distortion corrections and 3D electronic beam steering. This device is able to reach very high acoustic power delivery. For a detailed description of this unique system, the reader can refer to Citation[35]. shows the brain HIFU system relying on a quasi-random distribution of 8-mm diameter mono-elements connected with its water-cooling and coupling system. In vitro experiments of human transcranial focusing were achieved with this system. The corresponding beam directivity pattern in the focal plane (140 mm focal distance) is presented in and . It clearly emphasizes the necessity to correct for skull bone distortions. The beam profile is much thinner and secondary lobes have been totally destroyed, but the most important result lies in the comparison between the acoustic energy deposition at focus with and without time reversal correction. While using the same acoustic energy emitted on the HIFU array, the energy deposition obtained at focus by the time reversal process is more than 20 times higher than the one obtained without any aberration correction.
Figure 1. (a) A 300-element HIFU array with a water-cooling and coupling system and the 300 electronic channels driving the HIFU brain array. A curved array (128 elements–3 MHz) is embedded in the vicinity of the HIFU array in order to provide a low-quality echographic image of the skull surface and brain for a rough positioning of the complete system with respect to the head. (b) Quasi Random distribution of the 300 elements into a spherical mould. (c, d) 2D scan of the transcranial focal spot: Distribution of the acoustic energy deposition in the focal plane of the array (z = 140 mm depth) without (c) and with (d) time reversal correction of the skull distortions.
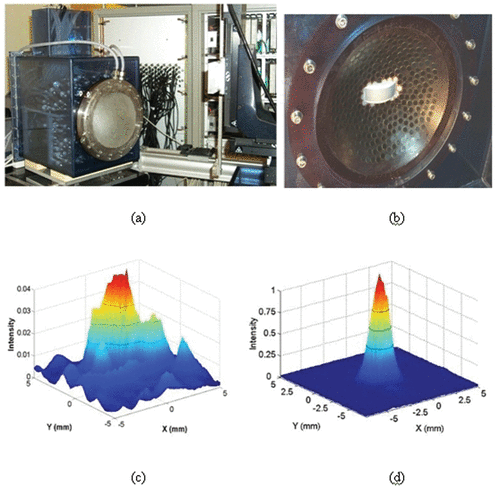
Using this high-power, fully programmable HIFU system based on 300 independent channels (220 transmit channels and 80 transmit / receive channels), the Paris group achieved, in 2005, the first experiments of transcranial HIFU thermal necrosis in a set of 20 sheep Citation[41]. The adaptive focusing technique used in the first animal's trials consisted in introducing a very small hydrophone near the targeted location during a pre-treatment biopsy. An impulse signal was then emitted element by element and recorded on the hydrophone. The hydrophone was removed at the end of the biopsy. After this calibration step, a simple time reversal (or phase conjugation operation for monochromatic signals) of the recorded signals allows to refocus the acoustic energy at the initial location of the hydrophone. Ten sheep were woken up after the treatment and no particular side effects were observed. These sheep were sacrificed in three groups respectively, 1, 2 and 3 weeks after the treatment. The histological examination confirmed that thermal necrosis had been induced in the targeted area and that no skin and skull burns occurred.
Following these pioneer experiments (based on a minimally invasive technique), a new animal investigation testing a fully non-invasive approach Citation[42] was proposed and validated in 2005 on monkeys (Macaca fascicularis). This non-invasive approach Citation[42] consisted in simulating the first step of the time reversal experiment using a priori information about the 3D architecture of the skull bone. First, a 3D CT scan of the monkey was performed, as seen in . The grayscale images obtained in Hounsfield units were converted into local acoustical parameters (speed of sound, density and ultrasonic absorption) as explained i Citation[15], Citation[17]. Then, the 3D propagation of an ultrasonic wave initiated from the targeted location bone was simulated with a 3D finite differences code (see ). After numerical propagation through the 3D skull bone model signals were recorded at the location of virtual HIFU array elements.
Figure 2. The different steps of the brain HIFU therapy guided by Ctscans: (a) the monkey is imaged into a CT scanner. The head is fixed on a house-made stereotactic frame. (b) Treatment software allows for the selection of the targeted spot. From these data, numerical simulation of the 3D transcranial ultrasonic propagation enables the estimation and correction of skull aberrations for the HIFU treatment. (c) One week after the CT scan exam, the monkey's head is again fixed into the house-made stereotactic frame. (d) The HIFU array is coupled to the monkey's head via the stereotactic frame, thus ensuring the concordance of CT scan reference frames and the HIFU experimental reference frame. The HIFU treatment is then performed using the calculated emission signals.
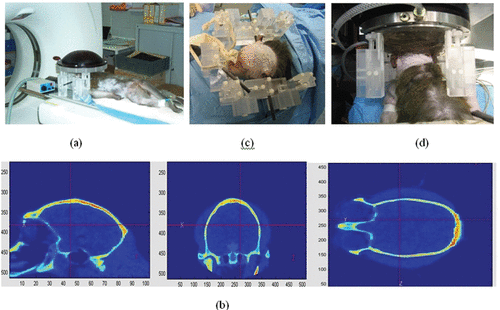
Figure 3. (a) Principle of the transcranial time reversal focusing technique guided by CT scans. (b) 3D simulation of the wave propagation (finite differences): wave field distribution at different time steps (at t = 0 µs, t = 8 µs, t = 16 µs, t = 24 µs, t = 32 µs, t = 40 µs, t = 48 µs, t = 56 µs, t = 64 µs) in a selected plane. (c) The monkey was sacrificed 1 week after treatment: the 7T MR images of the fixed brain clearly show the thermal necrosis at the targeted location using a steady state free precession (SSFP) sequence. The histological examination clearly shows thermal acidophilic necrosis in the area treated with time reversal correction and normal pattern (neurons, oligodendrocytes, capillaries) in the area treated with a non-corrected HIFU beam.
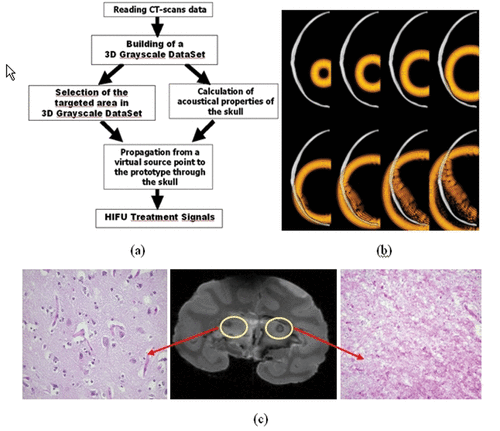
One week after the CT scan, the monkey is again fixed into the stereotactic frame (). The HIFU array is then coupled to the monkey's head via a water-cooling and coupling system (). The treatment is then performed using phase aberrations deduced from the 3D simulations. Monochromatic signals are emitted by each element of the array using as an emission delay the time-reversed version of the estimated phase aberrations. Thirty sonifications of the therapy beam are achieved using all array elements. Each shot duration was 10 s and the cooling time between each sonification was fixed to 15 s. Two treatments were performed with the same parameters. First, a treatment sequence was performed in the right hemisphere of the brain using a corrected therapy beam. A second treatment sequence was performed in the contralateral part of the brain with an uncorrected HIFU beam. The treatment duration was identical for both treatments. After the HIFU experiment, the monkey was woken up and a basic neurological score was given showing that no side effects occurred. The monkey's consciousness was normal, its appetite was good and the motor functions were not affected. For a more detailed description of this work, the reader can refer to Citation[42].
One week after the treatment, the monkey was sacrificed. No burns were observed on the skin, on the outer and inner tables of the skull but some petechia on the outer surface of the brain had been induced. shows a 7T MR image of the fixed brain. It clearly shows the thermal necrosis at the targeted location using a steady state free precession (SSFP) sequence. The histological examination also confirmed thermal acidophilic necrosis in the area treated with the time reversal correction. A normal pattern (neurons, oligodendrocytes, capillaries, i.e. no damage) was observed in the area treated with a non-corrected HIFU beam.
These promising results represent an important step towards human brain ultrasonic therapy. A detailed description of these in vivo animal trials can be found i Citation[41], Citation[42]. Beyond the thermal ablation application, the validation of this adaptive focusing technique guided by CT scans will be applicable at lower levels of acoustic energy deposition for the ultrasonic targeting of cerebral gene therapy and sonothrombolysis. Based on these works, ultrasonic adaptive focusing techniques were also proposed for high-resolution brain imaging Citation[43].
Transcostal focusing for liver HIFU treatments
The problem of transcostal focusing for liver ultrasonic therapy is also a great challenge for therapeutic ultrasound. Ribs introduce a severe amplitude and phase aberration on the focusing wavefront Citation[29]. It results in the creation of secondary sidelobes in the focal area but, perhaps even more importantly, a non-negligible part of the emitted HIFU beam will be significantly absorbed by the ribs and consequently will not contribute to the acoustic heat deposition at the desired focal location. Again, time reversal focusing represents an appropriate way to correct for these amplitude and phase aberrations. Tanter et al. showed that time reversal acts as a spatial and temporal matched filter Citation[44–46]. In other words, for a given acoustic energy emitted by the array, it optimizes the acoustic energy deposition at the focus. This property holds even if the medium is absorbing. Time reversal was successfully applied to many application fields Citation[47] including medical applications Citation[48]. For liver treatments, time reversal focusing can be achieved using an implanted hydrophone. Aubry et al. achieved in vitro experiments in 2005 to evaluate the feasibility and efficacy of such an aberration correction technique Citation[49]. The quasi-random sparse array described in the previous section was placed in front of a freshly excised and degassed sheep rib cage. The rib cage is partially shadowing the beam. Fresh and degassed liver samples are placed behind the rib cage. The HIFU array, the rib cage and the liver samples are immersed in a watertank containing degassed and deionized water. shows the spatial distribution of the pressure amplitude obtained in the focal plane after propagation through the rib cage. The conventional focusing is achieved by assuming the speed of sound to be homogeneous in the medium. Without any correction, the pressure field in the focal plane was affected by both inhomogeneous attenuation and phase distortion and three main effects were observed: a mean 2-mm shift of the main lobe, a mean 1.25-mm spreading in the half width of the main lobe and up to a 20-dB increase in the secondary lobe level. shows the spatial distribution of the pressure field obtained in the focal plane using the time reversal process: the signals linking each element of the array to the implanted hydrophone are time reversed and sent back in the medium through the ribs. The spatial distribution of the acoustic pressure field is better and sidelobes have been significantly decreased. Reproducible transcostal necrosis was achieved in 20 fresh and degassed liver samples. shows one necrosis obtained with a single 5-s duration insonification using time reversal respectively for a 1000-W · cm−2 and 1600 W · cm−2 acoustic power delivery at focus Citation[50]. No histology exam was performed. Liver specimen was cut into thin slices transversally to the ultrasonic beam. The thermal necrosis was confirmed by the change of local optical properties of the cut liver slices corresponding to the focal area.
Figure 4. Spatial directivity pattern of the transcostal ultrasonic beam in the focal plane using (a) conventional focusing and (b) time reversal focusing. (c) Necrosis obtained using time reversal focusing through the rib cage in liver samples for a 5-s duration at 1000 W · cm−2 and for a 5-s duration at 1600 W · cm−2. (d) Temperature elevation at several locations on the rib cage with conventional and time reversal focusing. The temperature elevation is estimated using implanted thermocouples (Iron-constantan, 40 µm diameter, PhysiTemp Corp.).
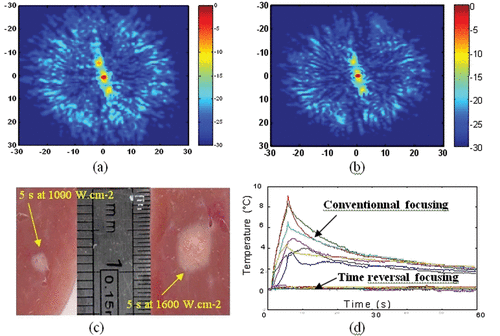
However, the most important result obtained in reference Citation[50] consists of the temperature elevations on the rib cage surface. Indeed, the focusing quality is not the only concern when focusing though the rib cage: one also has to pay attention to the pressure level on the ribs that may cause a temperature elevation due to the high absorption of the bones. While a conventional focusing technique (with each element firing the same acoustic energy) induces temperature elevations at several locations on the rib cage ranging from 4 to 8°C (for a 20°C elevation at the focus), the time reversal approach minimizes the heat deposition on the ribs by sending less energy on the transducers just in front of the ribs. For a 20°C elevation at focus in the liver, the mean temperature elevation with time reversal is less than 0.3°C (with a 0.1 standard deviation and a 0.4°C maximal value). For the same 20°C elevation at the focus, conventional focusing induces a 6°C mean temperature elevation (with a 2°C standard deviation and a 9.1°C maximal value).
As time reversal is a matched filter, it maximizes the pressure amplitude at the focus and naturally sonicates between the ribs, minimizing the temperature elevation on the rib surface (a mean 0.3°C compared with more than 5°C for the other techniques presented). Nevertheless, such a technique is invasive as it requires an acoustic source (or receiver) at the focus.
Now, an important question is could we achieve the ribs correction fully non-invasively? The answer is yes, but to achieve this goal, we need to use Transmit / Receive HIFU channels similar to the ones developed by the Paris group. This concept of therapeutic arrays able to work in both the transmit and receive modes, therefore called dual mode arrays, is now studied by several research groups and was introduced by Ebbini et al. Citation[51]. The idea is to use the HIFU array first as an echographic device imaging the ribs with a conventional grayscale B-mode imaging technique achieved with the HIFU probe. Then, from this conventional echographic image, we can easily choose the transducer elements that have to be turned off for the HIFU treatment. Another appropriate solution could be remotely to create this acoustic source by creating and collapsing a cavitation bubble in the targeted area as explained in Citation[52]. In a first step, the HIFU array emits a short impulse. The resulting focused wavefront creates a bubble cloud in the focal area. If the acoustic pressure field at focus is high enough (>10 MPa), bubbles collapse and create a shock wave that propagates from the focal area towards the HIFU array: the bubble collapses and acts as an acoustic source for the time reversal process. Applying the time reversal process to the resulting wavefield recorded on the HIFU array allows the aberrations induced by the ribcage to be corrected and the focusing quality of the next insonification to be improved.
Three-dimensional motion tracking and feedback correction for HIFU surgery
Another major problem that occurs when dealing with the HIFU treatment of abdominal organs (liver, kidney) concerns the correction of respiratory motion. Pernot et al. recently proposed a full ultrasound-based technique that solves this problem Citation[53]. The proposed approach greatly emphasizes the potential of HIFU systems that are able to work not only in the transmit mode, but also as receivers. Such Transmit/Receive multi-channel systems allow the real time tracking and feedback correction of the therapeutic beam during respiratory motion. A proof of principle of this capability was given in Citation[53] and first in vitro experiments were conducted with the 300-element system presented in the previous section. Recently, in vivo experiments were conducted on pigs that clearly demonstrate the feasibility of a HIFU beam tracking in real time the respiratory motion and remaining locked on the desired target Citation[54]. The basic principle of the correction technique is summarized in and . At time t0, four subapertures of the array are successively emitting a short ultrasonic impulse focused at a so-called tracking location 1 cm in front of the focal spot and recording the backscattered echoes (speckle noise). This sequence of four ultrasonic shots is repeated at time t0 + Δt (Δt ∼ 100 ms) and standard 1D cross-correlations of successive echoes permit estimation of the displacement along the beam axis of each subaperture. As the subapertures’ beam angles only depend on the geometry of the probe and the tracking location, the three components of the displacement vector between time t0 and t0 + Δt can be deduced from a simple and robust triangulation. As soon as the 3D displacement is calculated, the time delays applied on each of the 300 array elements are corrected in order to move the therapeutic focal point according to the calculated value. These operations are achieved very quickly (less than 5 ms). The therapy beam is then fired during nearly 95 ms and then stopped in order to achieve a new set of tracking sequences. The complete process consists of interleaving therapeutic and tracking sequences. The HIFU duty cycle can reach values higher than 95% ensuring an almost continuous therapeutic insonification while remaining locked on the targeted location. For a detailed description of the process, the reader can refer to Citation[53].
Figure 5. Basic principle of the real time motion correction. (a) Four subapertures successively send short ultrasonic impulses focusing at the tracking location. (b) Standard 1D cross-correlations between successive sets of acquisitions permit to recover the 3D displacement vector. (c) Displacement trajectory of a liver sample mounted on three-axis linear motors. Necrosis obtained in the moving liver sample (d) without and (e) with real time motion correction.
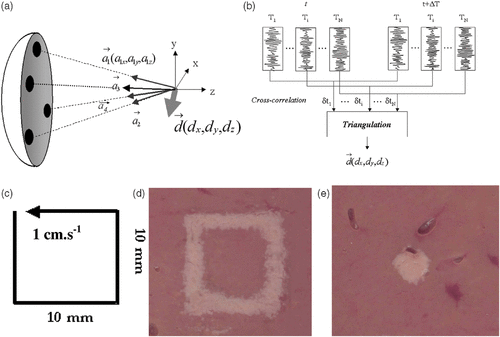
Pernot et al. conducted laboratory experiments in 15 fresh and degassed pork liver samples mounted on three-axis linear motors imposing any kind of desired 3D displacements trajectory. Experiments were achieved while imposing a square trajectory in the focal plane of the array at 140 mm depth with a 1-cm · s−1 speed as seen in . Necrosis was induced in the moving liver sample using a 2700-W · cm−2 insonification during 15 s without correcting the therapeutic beam. In the non-corrected experiment, the beam is not locked on the target and the necrosis clearly follows the sample trajectory (). When the tracking and feedback correction of the therapy beam is turned on, the necrosis remains locked on the targeted point (). As the acoustic energy deposition is much more efficient (the beam focuses always at the same location in the liver sample), much less time is needed to reach necrosis. The therapeutic beam in the corrected experiment consists of a 2700-W · cm—2 energy deposition at focus during only 5 s. This point illustrates a very important feature of real-time motion tracking and correction of the HIFU beam. As the acoustic energy deposition is much more efficient, this modality should allow a dramatic reduction in the time needed to treat a given tissue volume. As explained in Citation[53], treatment times could be decreased by roughly three times while using motion correction processing.
Finally, (52) describes in vivo experimentations that were conducted in pigs. 3D motion tracking was achieved in real time using the HIFU array and interleaving tracking sequences (5 ms) and HIFU therapy sequences (50 ms with a 2000-W/cm2 acoustic deposition at focus). Preliminary results show that in vivo 3D motion tracking is feasible as shown on where the three components of the respiratory motion can be seen. At time t = 35 s, the anesthetized pig had a sudden movement that was caught by the tracking HIFU system. After such a movement the motion of the liver can still be measured.
Figure 6. (a) In vivo investigation: the anesthetized pig is placed in front of the water cooling and coupling balloon of the 300-element system. (b) Real-time recording of the 3D respiratory motion using the HIFU array (red: superior-inferior motion, blue: lateral motion, green: anterior posterior motion).
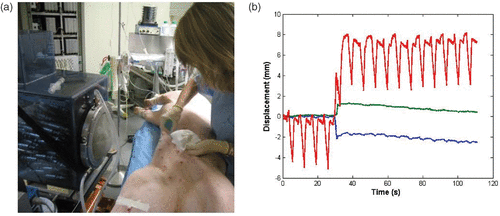
Conclusion
Although significant progresses have been made in focusing through bone interfaces like human skull or rib cage, the clinical validation of these techniques remains to be done. The high technological level needed to correct these kinds of aberrations encouraged the development of HIFU arrays driven by multi-channels transmit electronics. The extension of HIFU arrays capabilities both to transmit and receive modalities should also find interesting applications. It could solve the problem of transcostal focusing and the major issue of respiratory motion tracking and correction. Feasibility studies have already emphasized this potential.
References
- White DN, Clark JM, Chesebrough JN, White MN, Campbell JK. Effect of skull in degrading the display of echoencephalographic B and C scans. J Acoust Soc Am 1968; 44: 1339–1345
- White DN, Clark JM, White DA, Campbell JK, Bahuleyan K, Kraus AS, Brinker RA. The deformation of ultrasonic field in passage across the living and cadaver head. Med Biol Eng 1969; 7: 607–618
- Fry WJ, Mosberg WH, et al. Production of focal destructive lesions in the central nervous system with ultrasound. J Neurosurg 1954; 11: 471–478
- Fry WJ, Barnard JW, Fry FJ, Krumins RF, Brennan JF. Ultrasonic lesions in the mammalian central nervous system. Science 1955; 122: 517–518
- Basauri L, Lele PP. A simple method for production of trackless focal lesions with focused ultrasound: Statistical evaluation of the effects of irradiation on the central nervous system of the cat. J Physiol 1962; 160: 513–514
- Dunn F, Fry F. Ultrasonic threshold dosages for the mammalian central nervous system. IEEE Trans Biomed Eng 1971; 18: 253–256
- Robinson TC, Lele PP. An analysis of lesion development in the brain an in plastics by high-intensity focused ultrasound at low-Megahertz frequencies. J Acoust Soc Am 1972; 51: 1333–1351
- Fry FJ, Sanghvi NT, Morris RF, Smithson S, Atkinson L, Dines K, Franklin T, Hastings J. A focused ultrasound system for tissue volume abalation in deep seated brain sites. Proc IEEE Ultrason Symp. 1986
- Vykhodtseva NI, Hynynen K, Damianou C. Pulse duration and peak intensity during focused ultrasound surgery: Theoretical and experimental effects in rabbit brain in vivo. Ultrasound Med Biol 1994; 20: 987–1000
- Fry FJ, Barger JE. Acoustical properties of the human skull. J Acoust Soc Am 1978; 63: 1576–1590
- Phillips PD, Smith S, Von Ramm O, Thurstone F. Sampled aperture techniques applied to B-mode echoencephalography. Acoust Hologr 1975; 6: 103–120
- Thomas J-L, Fink M. Ultrasonic beam focusing through tissue inhomogeneities with a time reversal mirror: Application to transskull therapy. IEEE Trans Ultrason Ferroelectr Freq Control 1996; 43: 1122–1129
- Hynynen K, Jolesz FA. Demonstration of potential noninvasive ultrasound brain therapy through an intact skull. Ultrasound Med Biol 1998; 24: 275–283
- Sun J, Hynynen K. Focusing of therapeutic ultrasound through a human skull: A numerical study. J Acoust Soc Am 1998; 51: 1333–1351
- Pernot M, Aubry J-F, Tanter M, Thomas J-L, Fink M. Experimental validation of finite differences simulations of the ultrasonic wave propagation through the skull. Proc IEEE Ultrason Symp 2001; 2: 1547–1550
- Clement GT, Hynynen K. A non-invasive method for focusing ultrasound through the skull. Phys Med Biol 2002; 47: 1219–1236
- Aubry J-F, Tanter M, Pernot M, Thomas J-L, Fink M. Experimental demonstration of non invasive transskull adaptive focusing based on prior CT scans. J Acoust Soc Am 2003; 113: 85–93
- Tanter M, Thomas JL, Fink M. Focusing and steering through absorbing and aberrating layers: Application to ultrasonic propagation through the skull. J Acoust Soc Am 1998; 103: 2403–2410
- Clement GT, Hynynen K. Micro-receiver guided transcranial beam steering. IEEE Trans Ultrason Ferroelectr Freq Control 2002; 49: 447–453
- Clement GT, White JP, Hynynen K. Investigation of a large area phased array for focused ultrasound surgery through the skull. Phys Med Biol 2002; 45: 1071–1083
- Clement GT, Hynynen K. Criteria for the design and characterization of large-area arrays for transkull ultrasound surgery and therapy. Proc IEEE Ultrason Symp 2000; 12: 1243–1246
- Clement GT, Sun J, Giesecke T, Hynynen K. A hemisphere array for non-invasive brain therapy and surgery. Phys Med Biol 2000; 45: 3707–3719
- Dupenloup F, Chapelon JY, Cathignol D, Sapozhnikov OA. Reduction of the grating lobes of annular arrays used in focused ultrasound surgery. IEEE Trans Ultrason Ferroelectr Freq Control 1996; 43: 991–998
- Lockwood GR, Foster FS. Optimizing the radiation pattern of sparse periodic two-dimensional arrays. IEEE Trans Ultrason Ferroelectr Freq Control 1996; 43: 15–19
- Goss JA, Frizzell LA, Kouzmanoff JT, Barich JM, Yang JM. Sparse random ultrasound phased array for focal surgery. IEEE Trans Ultrason Ferroelectr Freq Control 1996; 43: 1111–1121
- Gavrilov L, Hand J. A theoretical assessment of the relative performance of spherical phased arrays for ultrasound surgery. IEEE Trans Ultrason Ferroelectr Freq Control 2000; 47: 125–138
- Botros YY, Ebbini ES, Volakis JL. Two step hybrid virtual array-ray (VAR) technique for focusing through the ribs. IEEE Trans Ultrason Ferroelectr Freq Control 1998; 45: 989–999
- Botros Y, Volakis JL, Van Barren P, Ebbini ES. A hybrid computational model for ultrasound phased array heating in presence of strongly scattering obstacles. IEEE Trans Biomed Eng 1997; 44: 1039–1050
- Kennedy JE, Clarke RL, Ter Haar GR. The effects of absorbers such as ribs in the HIFU beam-path on the focal profile. Proc. 2nd International Symposium on Therapeutic Ultrasound 2003; 195–92: 185–192
- Fan X, Hynynen K. Ultrasound surgery using multiple sonications—Treatment time considerations. Ultrasound Med Biol 1996; 22: 471–482
- Wang H, Ebbini ES, O’Donnell M, Cain CA. Phase aberration correction and motion compensation for ultrasonic hyperthermia phased arrays: Experimental results. IEEE Trans Ultrason Ferroelectr Freq Control 1994; 41: 34–43
- Allen M, Rivens I, Visioli A, Ter Haar G. Focused ultrasound surgery (FUS): A non-invasive technique for the thermal ablation of liver metastases. Seattle, 2002. Proc ISTU
- Kubo H, Hill B. Respiration gated radiotherapy treatment: A technical study. Phys Med Biol 1996; 41: 83–91
- Shirato H, Shimizu S, Kunieda T, et al. Physical aspects of a real-time tumor-tracking system for gated radiotherapy. Int J Radiat Oncol Biol Phys 2000; 48: 1187–1195
- Pernot M, Aubry JF, Tanter M, Thomas J-L, Fink M. High power transcranial beam steering for ultrasonic brain therapy. Phys Med Biol 2003; 48: 2577–2589
- Bonnefous O, Pesque P. Time domain formulation of pulse-Doppler ultrasound and blood velocity estimation by cross correlation. Ultrason Imaging 1986; 8: 73–85
- Hein IA, O’Brien WD. Current time-domain methods for assessing tissue motion by analysis from reflected ultrasound echoes: A review. IEEE Trans Ultrason Ferroelectr Freq Control 1993; 40: 84–102
- Capineri L, Scabia M, Masotti L. A Doppler system for dynamic vector velocity maps. Ultrasound Med Biol 2002; 28: 237–248
- Tanter M, Bercoff J, Sandrin L, Fink M. Ultrafast compound imaging for 2-D motion vector estimation: apllication to transient elastography. IEEE Trans Ultrason Ferroelectr Freq Control 2002; 49: 1363–1374
- Houghton A, Rees G, Ivey P. A method for processing laser speckle images to extract high-resolution motion. Meas Sci Technol 1997; 8: 611–617
- Aubry JF, Pernot M, Tanter M, Boch A-L, Kujas M, Seilhean D, Fink M. In vivo transcranial brain surgery with an ultrasonic time reversal mirror. 2006, J Neurosurg to appear in May Issue
- Marquet F, Aubry JF, Boch AL, Kujas M, Tanter M, Fink M. Non-invasive transcranial brain therapy guided by CT scans: An in vivo monkey study. Proceedings of the International Society for Therapeutic Ultrasound, Oxford, 2006
- Tanter M, Thomas JL, Fink M. Time reversal and the inverse filter. J Acoust Soc Am 2000; 108: 223–234
- Tanter M, Aubry JF, Gerber J, Thomas JL, Fink M. Optimal focusing by spatio-temporal inverse filter: Part I. Basic principles. J Acoust Soc Am 2001; 101: 37–47
- Aubry JF, Tanter M, Gerber J, Thomas JL, Fink M. Optimal focusing by spatio-temporal inverse filter: Part II. Experiments. J Acoust Soc Am 2001; 101: 48–58
- Vignon F, Aubry JF, Tanter M, Margoum A, Fink M. Adaptive focusing for transcranial ultrasound imaging using dual arrays. J Acoust Soc Am 2006; 120: 2237–2745
- Fink M, Cassereau D, Derode A, Prada C, Roux P, Tanter M, Thomas JL, Wu F. Time reversed acoustics. Rep Prog Phys 2000; 63: 1933–1995
- Fink M, Montaldo G, Tanter M. Time reversal acoustics in biomedical engineering. Annu Rev Biomed Eng 2003; 5: 465–497
- Pernot M, Aubry JF, Tanter M, Fink M. Ultrasonically induced necrosis through the rib cage based on adaptive focusing: Ex vivo experiments. Proc IEEE Ultrason Symp 2003; 1: 833–836
- Pernot M, Aubry JF, Tanter M, Fink M. Ultrasonically induced necrosis through the rib cage based on adaptive focusing: Ex-vivo experiments. Proc IEEE Ultrason Symp, Hawaii, October, 2003
- Yao H, Ebbini ES. Refocusing dual-mode ultrasound arrays in the presence of strongly scattering obstacles. Proc IEEE Ultrason Symp 2004; 1: 239–241
- Pernot M, Montaldo G, Tanter M, Fink M. ‘Ultrasonic stars’ for time reversal focusing using induced cavitation bubbles. Appl Phys Lett 2006; 88: 034102
- Pernot M, Tanter M, Fink M. 3D real-time motion correction in high-intensity focused ultrasound therapy. Ultrasound Med Ther 2004; 30: 1239–1249
- Marquet F, Pernot M, Aubry JF, Montaldo G, Tanter M, Fink M. In-vivo non-invasive motion tracking and correction in high intensity focused ultrasound therapy. IEEE EMBS Proc, New York, September, 2006