Abstract
Purpose: Computational feasibility of a new non-invasive microwave hyperthermia technique that employs dual deformable mirror is investigated using simplified computational tools and anatomically realistic breast models.
Materials and methods: The proposed technique employs two pairs of electromagnetic sources and continuously deformable mirrors to focus the electromagnetic radiation at the target site for hyperthermia. The mirror functions like a continuum of radiating elements that offer effective scan coverage inside the breast with efficient field focusing at the target location. The electric field focusing and temperature mapping in the two-dimensional numerical simulations are investigated using wave propagation and bio-heat transfer models respectively. The method of moments, a popular numerical simulation tool, is used to model the electric field maintained by the deformable mirrors for continuous wave excitation. The electromagnetic (EM) energy deposited by the mirrors is used in the steady state bio-heat transfer equation to quantify the temperature distribution inside two-dimensional anatomically realistic breast models.
Results: Feasibility of the proposed technique is evaluated using numerical breast models derived from magnetic resonance images of patients with variation in breast density, age and pathology.
Conclusions: The computational study indicates preferential EM energy deposition and temperature elevation inside tumor tissue with minimum collateral damage to the neighboring normal tissues. Simulation results obtained for the magnetic resonance (MR) breast data appear promising and indicate the merit in pursuing the investigation using 3D computational models.
Introduction
The most common cancers diagnosed amongst women in the United States include cancer of the lungs and breast. Breast cancer accounts for one in every three cancer cases diagnosed and is the second leading cause of cancer deaths amongst women Citation[1]. It is anticipated that one in every eight women will succumb to breast cancer during her life time Citation[2]. The incidence and mortality rates reported for breast carcinoma are of utmost public health concern in the United States and elsewhere in the world. Depending on cancer stage, age and health factors, surgery or surgery combined with radiation or chemotherapy is prescribed to treat the malignancy. Besides radiation and chemotherapy, EM hyperthermia technique is being actively pursued for effective treatment of breast carcinoma Citation[3–7]. The relatively high contrast between the electrical property of normal and cancerous tissues Citation[8–10] leads to preferential deposition of EM energy and selective thermal elevation within the tumor. In EM hyperthermia, tumor temperature elevation to 39°C–45°C for prolonged time duration has been reported to increase the susceptibility of malignant cells to ionizing radiation rendering it an effective adjuvant therapy technique for effective treatment of breast carcinoma Citation[11–14]. The array applicators proposed for local and regional heating utilizes multiple electromagnetic sources to control the phase and amplitude of the incident field for optimal power deposition Citation[14]. Tissue heating using multi-element applicators involves careful control of multiple parameters such as the number of elements, positioning and arrangement, and the phase and amplitude for optimal power deposition.
An EM hyperthermia technique employing two deformable mirrors is presented in this paper for the treatment of localized breast tumors. Unlike conventional multi-radiator systems, the proposed hyperthermia technique focuses the incident electric field at the tumor target using a pair of deformable mirrors. Feasibility of the mirror-based microwave hyperthermia technique is evaluated using two-dimensional wave propagation and bio-heat transfer models. Computational models developed for the dual mirror technique are evaluated using high resolution MRI breast images of women reported to have breast malignancy. Section two of the paper presents the functionality of the proposed technique for localized breast tumors. The selection of mirror deformations and bio-heat transfer models for EM field focusing are presented in section three. The hyperthermia simulation results on several MR breast images are presented in section four followed by the discussion and conclusion sections.
Deformable mirror hyperthermia technique
Deformable mirror
Deformable mirrors are widely used in adaptive optic systems for real time control of the incident optical wave front Citation[15–17]. In this paper we extend the concept of adaptive mirrors to the microwave regime to adaptively focus the EM waves inside the target tumor for selective power deposition. The proposed hyperthermia technique relies on the ability of deformable mirror to conform to a predetermined shape for adaptive field focusing during hyperthermia. A deformable mirror consists of a thin flexible material or an array of closely packed mirror segments with reflective coating resting above an array of actuator circuits Citation[14–16]. The deformation of the mirror surface is controlled by the force exerted by the actuators beneath the reflective mirror material. The deformation of a thin flexible mirror under the influence of the actuator load, F(x, y) is given byCitation[18], where f(x, y) and D are the deformation and flexural rigidity of the mirror respectively and F(x, y) is the force per unit length acting normal to the flexible material. For a given actuator load, Equation 1 with appropriate boundary conditions on mirror edges yields the deformation Citation[18]. Another possible design to achieve mirror deformation in 3D is to construct a 2D array of closely spaced hexagonal mirror segments. Position actuators beneath the individual segments could be controlled to achieve a piecewise approximation of the desired mirror deformation. At the operating frequency (500 MHz), diffraction from gaps between the mirror segments will have negligible impact on the beam pattern and the segmented mirror array could be used to steer and focus the EM field at the target site. Large mirror deflections required to focus the incident EM waves could be realized using mechanical actuators.
Dual mirror hyperthermia
A schematic illustration of the dual mirror hyperthermia technique is shown in Citation[19]. The proposed technique employs two pairs of directional EM sources and deformable mirrors inside a tank filled with coupling/coolant solution. During hyperthermia, the patient lies in prone position with the breast pendant inside the tank and the breast is simultaneously irradiated by two deformable mirrors positioned on either sides of the breast. The mirror shapes used for hyperthermia depend on the tumor spatial location reported in clinical breast diagnosis report. The desired mirror shapes for field focusing could be achieved by adaptively controlling the actuator load, F(x, y) in Equation 1. The estimated mirror shape functions like a continuum of radiating elements and focuses the incident EM radiation at the tumor target inside the breast. Breast illumination from both sides was observed to reduce undesired skin burns associated with the single mirror hyperthermia model Citation[20]. During hyperthermia, the liquid inside the tank could be maintained at a constant temperature via external circulation. The circulating coupling solution serves as a thermal sink for the skin and minimizes the incidence of secondary hot spots and skin burns. The high loss tangent of the couplant damps spurious scattering from mirror edges and the backscattered field from the breast from interacting with the mirror assembly. The liquid also offers low impedance mismatch for the EM wave incident on the breast.
Figure 1. (a) Schematic illustration of the dual mirror microwave technique for thermal therapy of localized breast cancer (not drawn to scale). (b) Arrangement of the mirror and breast coronal section in the computational model; a1 = a2 = 1.25λc, b1 = b2 = 3.75λc, c1 = c2 = D, d1 = d2 = 2λc, D = max{Xlen, Ylen}; s1, s2: sources (figure drawn approximately to scale; see for λc).
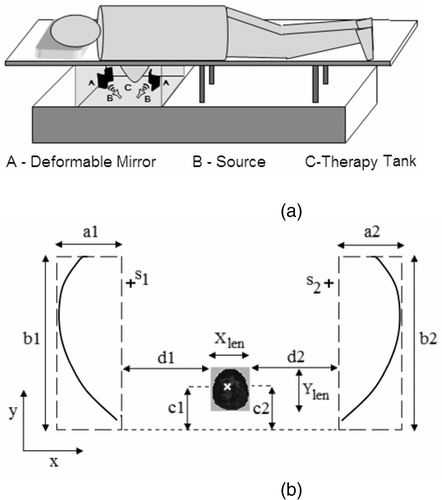
shows the two-dimensional geometry of the dual mirror microwave technique for hyperthermia used in the computational study. is not drawn to scale and shows the mirror dimension and location with respect to the breast and tumor tissues. The dimensions in were chosen based on the field pattern obtained for different mirror shapes and model geometry. The major mirror dimension of 3.75λc in effectively reflects and redirects the incident field towards the target with minimal creepy waves. Also, the mirror dimension in is several wavelengths larger than the physical dimension of the breast tissue (assuming benign tissue properties), thereby ensuring coverage over the range necessary to scan the entire breast.
Numerical models
Numerical feasibility study requires computational techniques to model electromagnetic wave propagation for energy deposition inside the breast and to quantify heat transfer within the breast tissue for hyperthermia. The computational models implemented for the feasibility study include (i) Mirror shape estimation for adaptive field focusing inside tumor, (ii) Electric field computation for EM energy deposition and (iii) Bio-heat transfer model for steady state temperature calculation inside the breast. Two-dimensional computational models were implemented to get preliminary insights into the feasibility of the proposed technique and to demonstrate the concept than provide a full solution.
Adaptive field focusing
The objective of this module is to determine the mirror shape that can focus the EM radiation at the target site. Large mirror dimensions of the order of (3λc–4λc) used in the simulations enabled to use geometrical optics approximation for mirror shape estimation. For computational simplicity, mirror deformation for field focusing was estimated using ray tracing technique. During mirror shape estimation, the breast and external medium were assigned the dielectric property of benign mammary tissue and the lossy couplant respectively to account for refraction in ray tracing.
Focusing strategy
illustrates the first strategy in mirror shape estimation for adaptive field focusing. In , let A and T denote the location of the source and tumor center
respectively. Assuming homogeneous tissue property inside the breast and coupling medium, the ray-tracing model was implemented to obtain a set of contiguous points
inside S to estimate
capable of focusing the incident field at
. The objective is to fit a curve,
inside the search space, S denoted by the bounded dotted lines such that rays from A are focused at T after reflection from the mirror and refraction at the tissue interface. This was accomplished by tracing the path traveled by the rays from T to A. At the tissue interface, the tangential and normal components computed at the intersection, B were used with the law of refraction to determine the outgoing transmitted ray. For each ray traced from T, the mirror deformation inside S re-directs the incident ray, OB towards A only if the angle of incidence equals angle of reflection, i.e.:
Condition (2) requires the unit normal vector at O, to bisect the angle subtended by
as shown in . The surface tangent, CD at O redirects the rays from A to the tissue interface at B. Following this procedure, the surface tangents were calculated for all points inside S as shown in .
Mirror shape estimation for constructive interference
The next step in adaptive field focusing is to estimate by fitting a curve through the surface tangents inside S. Shapes that yield constructive interference at
for field focusing were estimated using the following procedure.
Start with an initial coordinate, (xi, yi) inside S near the source
Find the angle between surface tangent vector,
and its neighboring tangential vectors denoted by
, subtending angle
with
Retain surface tangents,
for which,
where
is a positive real threshold
Find the closest surface tangent
, such that vectors
and
are not collinear
If such a surface tangent vector,
exists then
Increment i = i + 1 and assign (xi, yi) as (xi+1, yi+1), a contiguous point to (xi, yi)
Repeat steps (1–5) for all
The surface tangents identified using steps 1 to 5 yield that maintain constructive interference of the incident field at
. Of the multitude possible shapes in , a mirror shape with major dimension approximately 3λc−4λc was chosen for field focusing. During mirror shape estimation, the region inside the breast model was assumed to be homogeneous. Corrections to the mirror shape to account for heterogeneity inside the breast tissue were not considered in the current preliminary study and will be treated in the subsequent investigation.
shows the mirror shapes obtained using steps 1 to 5 for the tumor location in . The location of the search space for the mirrors is based on the model geometry illustrated in . shows the histogram of the path lengths computed for the rays traced from to
for mirror 1 in . The histogram in indicates that the mirror shape estimated following Equation 2 and steps 1 to 5 yields constructive interference at the target location. The mirror shapes in and those estimated for the MR breast data in the simulations yielded concave shapes. The actuator load distribution that yields the estimated mirror deformation,
for individual mirrors could be estimated by minimizing the error,
EM energy deposition
EM wave interaction with the metal-coated deformable mirrors, EM sources and lossy dielectric breast were modeled using equivalent currents Citation[21]. shows the 2D representation of the proposed technique and the computational model. In , EM aperture sources are approximated by line sources, {I1, I2} enclosed within curved conducting wedges defined by the contours respectively to provide directional field pattern, and the deformable mirrors are modeled as thin conducting strips with contours
. The impressed line currents,
induces surface currents,
on
, and
on
respectively, and volume current, Jd inside the dielectric breast model. For TMz polarization, the z-directed impressed and equivalent currents maintain the total electric field,
inside the computational domain Citation[21]. In Equation 4, β is the wave number of the couplant with dielectric constant, εc and
is the heterogeneous dielectric permittivity inside the breast model. Equation 4 with the boundary condition,
yields the total field anywhere inside computational domain. The EM energy deposited inside the breast is then computed using the equation,
where σ is the tissue electrical conductivity.
Thermal model
The EM energy given by Equation 6 was used in Penne's bio-heat transfer equation (BHTE) to quantify the steady state temperature distribution inside the breast Citation[22]. In the presence of external power deposition, metabolic heat generation was neglected and the simplified steady state BHTE given by,was solved using the finite element method Citation[23]. In Equation 7,
denotes the tissue thermal conductivity; ωb denotes the blood perfusion rate; cb refers to the specific heat of blood; Tb is the arterial blood temperature, assumed to be constant; Qr is the power deposited due to spatial tissue heating and ‘T(x, y)’ is the steady state temperature distribution inside the tissue. Spatial heating inside the breast model is controlled by the tissue specific absorption rate,
where ρ is the tissue density and Erms is the root mean square value of the total field, Ez(x, y). The electromagnetic energy, Qr in Equation 8 forms the heat source for the steady state BHTE Equation 7. Equations 4–5 were used to compute the tissue SAR in Equation 8 which was then used to solve Equation 7 with the boundary condition,
to obtain the steady state temperature inside the breast. The boundary condition in Equation 9 maintains the temperature in the tank below the therapeutic temperature and acts as a thermal sink for skin and breast. In the computational model, SAR and temperature distributions inside the breast were controlled by the impressed line current densities, {I1, I2}. Tissue SAR necessary to achieve the desired therapeutic temperature was determined by gradually increasing the impressed current densities and computing thermal metrics inside the skin, benign and lesion tissues.
Simulations results
This section presents the numerical simulations carried out for the dual mirror microwave technique using 2D anatomically realistic breast models obtained from the MR breast images of symptomatic women. Anonymous MR breast data of symptomatic women used in this study were obtained from the Department of Radiology at Michigan State University.
Frequency selection
Frequency selection for hyperthermia was based on simulation studies that were conducted over 100–900 MHz using the electrical property of normal and malignant mammary tissues reported in Joines et al. Citation[10]. Higher power absorption in cancerous breast tissues reported in Citation[10] was used to narrow the frequency range to 200–800 MHz. For frequencies less than 500 MHz, mirror dimension used in the theoretical study based on the design illustrated in becomes physically large for the treatment of the human breast. Besides mirror dimension, EM field focusing at these frequencies are relatively large compared to the tumor size. On the other hand, frequencies above 700 MHz yielded focused field pattern at the target inside the breast with relatively higher SAR and temperature on the skin due to the relatively poor skin depth. shows the normalized field magnitude maintained by single and dual mirror set-ups at 500 and 700 MHz respectively inside the coupling solution in the absence of the breast tissue. Mirror dimension, EM field penetration inside tissue, tissue SAR and thermal hot spots inside the breast model were used to select 500 MHz as the operating frequency. In the interest of brevity, EM field and thermal simulations conducted for frequency selection were not included.
MR breast data – tissue permittivity map
T2 weighted MR image sequences reported to have lesions were used to evaluate the performance of the dual mirror microwave hyperthermia technique for localized breast cancer. The two-dimensional MR breast images were segmented into skin, tumor and normal (fat and glandular) tissues as shown in , and tissue electrical property reported in Citation[10], Citation[24] were used to obtain an equivalent permittivity distribution for the high resolution MR breast images. In the microwave regime, fibro-glandular tissues are more hydrous than fat and have higher dielectric permittivity. The dielectric property of the heterogeneous breast tissue was obtained using the mapping function,
Figure 6. Tissue permittivity map for the MR breast image. Boundary extraction for (a) skin and (b) tumor; (c) relative permittivity and (d) electrical conductivity (S/m) obtained using Equation 10 and Citation[10], Citation[24].
![Figure 6. Tissue permittivity map for the MR breast image. Boundary extraction for (a) skin and (b) tumor; (c) relative permittivity and (d) electrical conductivity (S/m) obtained using Equation 10 and Citation[10], Citation[24].](/cms/asset/94300477-d3d5-464c-a229-b8a7b42ad9f8/ihyt_a_272665_f0006_b.gif)
In Equation 10, ; Iμ and σI refer to the mean and standard deviation of the MR intensity image, I; η is a positive real number used to control the contrast between normal and tumor tissues depending on the amount of glandular tissue present in the MR breast image. For the breast models used in the simulations, η was varied depending on the radiological density of the MR image. The relative permittivity, ε′ and electrical conductivity, σ of the breast tissue at 500 MHz used for tissue permittivity mapping were taken from Citation[10] and Citation[24]. and show the relative permittivity and electrical conductivity map obtained for the MR breast image in and .
Hyperthermia simulations
Several MR breast images with lesions were used in the simulations. A subset of the MR breast images used for performance evaluation is listed in . includes patient data belonging to different age groups with variation in breast heterogeneity. For each patient, coronal section of the MR image with tumor was used in the simulation. Spatial information of the lesions reported in the MR breast images listed in was used to determine the mirror deformations. The mirror shapes estimated using the field focusing strategy were used in the electric field and temperature calculations for the anatomically realistic breast models. Thermal metrics computed for each breast model were used to determine the current intensities, {I1, I2} for the hyperthermia simulation.
Table I. Patient information for MR derived breast models.
to shows the coronal MR breast images used in the simulations. The high intensity signals indicated by the black contour correspond to the lesions listed in . lists the tissue properties used in the simulations. to shows the tissue SAR inside MR derived breast models for which , Tbenign,skin < 41° C and T0 = 30°C. The SAR distributions in to indicate preferential EM energy deposition at the lesion site. The corresponding steady state temperature distributions are shown in to . The steady thermal maps indicate temperature rise inside the lesion without damaging the surrounding normal tissues and skin.
Figure 7. Coronal MR breast images used in the hyperthermia simulations (a) patient data I, (b) patient data II, (c) patient data III, (d) patient data IV (black contours indicate tumor sites).
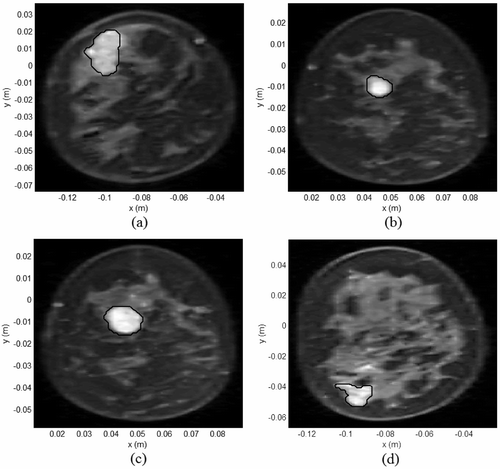
Figure 8. Tissue SAR (W/kg/m) inside MR derived breast models for which and
(a) Model I, (b) Model II, (c) Model III, (d) Model IV.
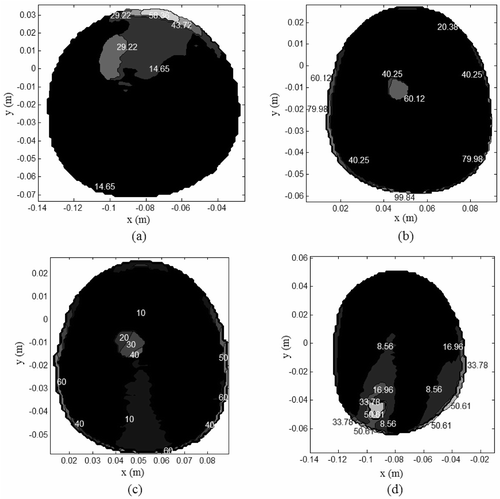
Table II. Electrical and thermal properties of breast tissues Citation[10], Citation[24–26] (ε = ε′–jε″, couplant permittivity, εc = 43.76-j22.82, cb = 4000 (J/kg/°C), Tb = 37°C, f = 500 MHz, λc = 0.0907m).
Discussion
Thermal metrics
Simulations results in indicate a single primary hot spot at the target tumor with the remaining breast tissue at a relatively lower temperature. Thus, collateral tissue damage was confined only to the benign tissues neighboring the lesion. As transient analysis was not studied in the thermal simulations, thermal dosage inside the breast models was computed using the following metrics and T90 calculations. Cumulative tumor tissue damage (TD) caused by the dual mirror microwave technique is quantified by,
Figure 9. Steady state temperature for the tissue SAR in (T0 = 30°C), (a) Model I, (b) Model II, (c) Model III, (d) Model IV.
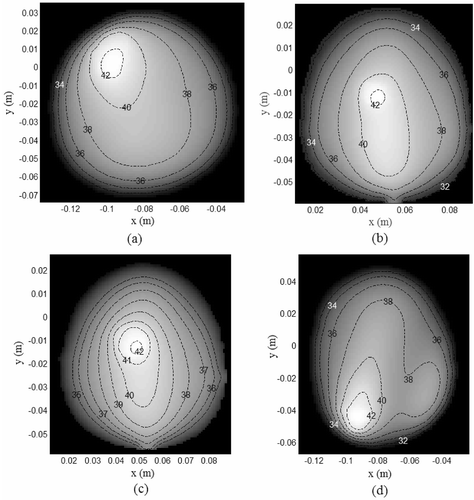
In Equation 11(a), Thyp refers to the therapeutic temperature, At is the total area of the tumor tissue and A1 is the area inside the tumor tissue with . Collateral tissue damage (CTD) to the neighboring benign tissues was computed for the MR breast data using,
where A2 is the tissue area inside the breast that correspond to healthy tissue including skin where
and An is the total area of the normal breast tissue. Equations 11(a) and (b) quantify tumor under dosage and normal tissue damage respectively. Tissue damage given by (11) and the T90 metrics calculated for the simulations with
are summarized in . Comparison of the thermal metrics in with the tumor dimension in indicate normal tissue damage to be the least for the smallest tumor (model II). As the tumor size increases, increase in the tumor dosage results in relatively higher CTD for larger tumors than that for smaller tumors.
Table III. Tumor and normal tissue damage for the hyperthermia simulations.
Variation in tissue permittivity
Numerical simulations were carried out to evaluate the ability of the estimated mirror shapes to focus the incident EM waves at the target inside a breast model with poor electrical contrast between normal and tumor tissues. Dielectric breast model III with 60% decrease in εtumor shown in and was analysed with the criteria and Tbenign,skin < 41° C for T0 = 30°C. shows the steady state temperature distribution calculated for the tissue permittivity in and . The steady-state thermal map in indicates a primary hot spot at the tumor site with minimal collateral damage to the surrounding glandular tissues. Decreasing εtumor lowers the electrical contrast between the normal and malignant tissues, which essentially reduces selective EM power deposition inside the target tumor. Simulation results in demonstrate the ability of the mirror shapes to focus inside the breast tissue with poor tumor tissue contrast.
Figure 10. Hyperthermia simulation for model III with 60% decrease in εtumor. (a) Dielectric constant; (b) electrical conductivity at 500 MHz using Equation 10 and Citation[10], Citation[24]; (c) steady state thermal contours.
![Figure 10. Hyperthermia simulation for model III with 60% decrease in εtumor. (a) Dielectric constant; (b) electrical conductivity at 500 MHz using Equation 10 and Citation[10], Citation[24]; (c) steady state thermal contours.](/cms/asset/ece649b4-22a3-4db0-b754-54af33238c2d/ihyt_a_272665_f0010_b.gif)
Another simulation was conducted to evaluate the field focusing capability of the deformable mirrors inside a healthy MR breast model. and shows the dielectric constant and electrical conductivity map for the healthy MR breast image in . Mirror shapes were estimated to focus the EM field at (−0.093, −0.0475, 0) m inside the breast. shows the tissue SAR computed for normal breast tissue property. The peak SAR value at the target location inside the healthy breast model in indicates the ability of the estimated mirror shapes to yield preferential EM energy deposition at the target. Simulation results in , indicate the field focusing ability and robustness of the proposed technique to variation in target tissue permittivity. The simulation results obtained for different breast data appear promising and indicate the merit in evaluating the feasibility of the dual deformable mirror microwave technique using realistic 3D models.
Figure 11. EM energy deposited inside the benign breast tissue for field focus at (−0.093, − 0.0475). (a) Tissue dielectric constant, (b) electrical conductivity, (c) coronal MR breast image, (d) Tissue SAR within the breast model (W/kg/m).
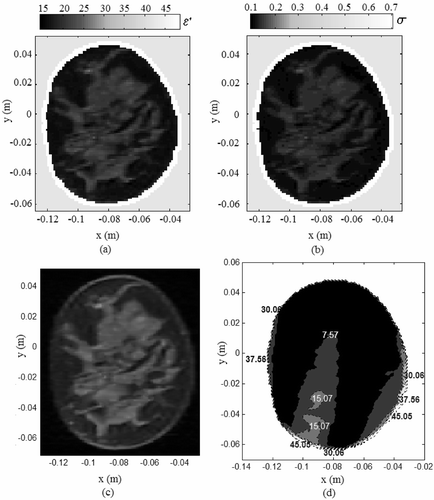
Optimistic results obtained in the 2D feasibility study on anatomically realistic breast models argue for the need to implement a full 3D model for a more realistic performance evaluation. In the 3D model, mirror shapes estimated using geometrical physics could serve as an initial estimate to refine the mirror shapes for field focusing inside a tumor volume by incorporating diffraction effects and using a 3D microwave source. In 3D, a deformable mirror could be realized using an array of mirror segments wherein the position and orientation of the individual segments could be mechanically controlled to achieve a piecewise approximation of the desired shape. In the proposed technique, the mirror shape and source placement could be adaptively adjusted to deposit EM energy anywhere inside the breast. An alternative approach for adaptive field focusing inside the breast tissue could be potentially achieved using a pair of rigid elliptical reflectors. In the case of rigid elliptical reflectors, field focusing inside the breast is limited, as the source alone could be moved. A full coverage of the breast for field focusing could be obtained by moving the source and the rigid reflector mirror far away from the breast. However, this might require the use of a very large applicator for the hyperthermia treatment. Unlike rigid elliptical reflectors, the ability of the deformable mirror to change its shape enables one to scan the breast and focus the high intensity EM radiation inside the target tissue without the need to move the patient or the source.
Conclusion
Computational feasibility of a dual mirror microwave technique for hyperthermia was investigated using MRI-derived 2D breast models. In the computer simulations, tissue SAR and steady-state temperature were observed to be the highest inside tumor, and the tissue SAR in the skin was higher than inside the normal breast tissue due to the relatively higher electrical conductivity of skin tissue. Though SAR deposition inside the skin was higher than the normal tissue, the steady state temperature inside skin was maintained at a relatively lower temperature by the external couplant solution (T0 = 30°C). Thermal metrics listed in indicate selective tumor tissue heating sparing the skin and surrounding normal tissues. The simulation results demonstrate the potential of the dual mirror hyperthermia technique to selectively deposit EM energy inside the target tissue with variation in tissue permittivity. Preliminary computational study of the proposed technique on MR breast images of symptomatic women with moderate, scattered and dense fibro-glandular tissues is encouraging and requires further investigation.
Acknowledgement
The MRI data was provided by Dr Gerald Aben in the Radiology Department at Michigan State University through Michigan State University IRB #06–376. We gratefully acknowledge the support and assistance provided by Dr Aben in this regard. We are also thankful to Ms Lori Hoisington who helped us interpret and access the MRI data.
References
- American Cancer Society. Breast cancer facts and figures 2005–2006. Cancer Society Inc., Atlanta 2006
- Jatoi I. Manual of breast diseases. Lippincott Williams & Wilkins;, Philadelphia 2001
- Vernon CC, Hand JW, Field SB, Machin D, Whaley JB, et al. Radiotherapy with or without hyperthermia in the treatment of superficial localized breast cancer: Results from five randomized controlled trials. International Collaborative Hyperthermia Group. Int J Radiat Oncol Biol Phys 1996; 35: 731–744
- Fenn AJ, Wolf GL, Fogle RM. An adaptive microwave phased array for targeted heating of deep tumors in intact breast: Animal study results. Int J Hyperthermia 1999; 15: 45–61
- Hehr T, Lamprecht U, Glocker S, Classen J, Paulsen F, Budach W, Bamberg M. Thermoradiotherapy for locally recurrent breast cancer with skin involvement. Int J Hyperthermia 2001; 17: 291–301
- Van Der Zee J, Koper PCM, Jansen RFM, De Winter KAJ, Van Rhoon GC. Re-irradiation and hyperthermia for recurrent breast cancer in the orbital region: A case report. Int J Hyperthermia 2004; 20: 1–6
- Fujimoto S, Kobayashi K, Takahashi M, Nemoto K, Yamamoto I, et al. Clinical pilot studies on pre-operative hyperthermic tumour ablation for advanced breast carcinoma using an 8MHz radiofrequency heating device. Int J Hyperthermia 2003; 19: 13–22
- Chaudhary SS, Mishra RK, Swarup A, Thomas JM. Dielectric properties of normal and malignant human breast tissues at radiowave and microwave frequencies. Indian J Biochem BioPhys 1984; 21: 76–79
- Surowiec AJ, Stuchly SS, Barr JR, Swarup A. Dielectric properties of breast carcinoma and the surrounding tissues. IEEE Trans Biomed Eng 1988; 35: 257–263
- Joines WT, Zhang Y, Li C, Jirtle RL. The measured electrical properties of normal and malignant human tissues from 50 to 900 MHz. Med Phys 1994; 21: 547–550
- Wust P, Hildebrandt B, Sreenivasa G, Rau B, Gellermann J, Riess H, Felix R, Schlag PM. Hyperthermia in combined treatment of cancer. Lancet Oncol 2002; 3: 487–497
- Dewhirst MW, Vujaskovic1 Z, Jones E, Thrall D. Re-setting the biologic rationale for thermal therapy. Int J Hyperthermia 2005; 21: 779–790
- Jones EL, Oleson JR, Prosnitz LR, Samulski TV, Vujaskovic Z, et al. Randomized trial of hyperthermia and radiation for superficial tumors. J Clin Oncol 2005; 23: 3079–3085
- Stauffer PR, Evolving technology for thermal therapy of cancer. Int J Hyperthermia 2005; 21: 731–744
- Freeman RH, Pearson JE. Deformable mirrors for all season and reasons. Applied Optics 1982; 21: 580–588
- Hardy JW. Adaptive optics – A progress review. Proc SPIE 1991; 1542: 2–17
- Tyson RK. Adaptive optics engineering handbook. Marcel Dekker Inc, New York 2000
- Mansfield EH. The bending and stretching of plates. 2nd. Cambridge University Press, Cambridge 1989
- Arunachalam K, Udpa SS, Udpa L. An RF therapy system for breast cancer treatment using deformable mirror. The 33rd Annual Rev Progress QNDE, Portland, OR 2006
- Arunachalam K, Udpa SS, Udpa L. Microwave breast cancer hyperthermia using deformable mirror. The 2006 IEEE AP-S Int Symp Ant Propagat USNC/URSI Nat Radio Sci Meeting;, New Mexico 2006
- Peterson AF, Ray SL, Mittra R. Computational methods for eelectromagnetics. The IEEE/OUP series on electromagnetic wave theory. Oxford University Press, Oxford 1997
- Wissler EH. “Pennes' 1948 paper revisited”,. J Appl Physiol 1998; 85: 36–42
- Jin JM. The finite element method in electromagnetic. 2nd. John Wiley & Sons, NJ 2002
- Gabriely S, Lau RW, Gabriel C. The dielectric properties of biological tissues: III Parametric models for the dielectric spectrum of tissues. Phys Med Biol 1996; 41: 2271–2293
- Van De Kamer JB, Van Wieringen N, De Leeuw AAC, Lagendijk JJW. The significance of accurate dielectric tissue data for hyperthermia treatment planning. Int J Hyperthermia 2001; 17: 123–142
- Bernardi P, Cavagnaro M, Pisa S, Piuzzi E. Specific absorption rate and temperature elevation in a subject exposed in the far-field of radio-frequency sources operating in the 10–900-MHz range. IEEE Trans Biomed Eng 2003; 50: 294–304