Abstract
Dynamic contrast-enhanced magnetic resonance imaging (DCE-MRI) is today the most popular method to assess perfusion in the body. Unfortunately, the technique is mostly used to determine semiquantitative parameters like time to peak enhancement. Perfusion quantification remains challenging using DCE-MRI. The method suffers from two major limitations. First, no standardized imaging technique is available. Secondly, no standard evaluation technique for perfusion quantification has been established which accurately describes the tracer kinetics of available contrast agents. Different imaging techniques using spoiled gradient echo sequences and the basics of the evaluation are presented. To assess perfusion changes due to hyperthermia using DCE-MRI one has to take into account the systematic variation of the arterial input function required for perfusion quantification. Hyperthermia modifies the contrast agent bolus in that a shorter and more highly concentrated bolus appears earlier in the tissue.
Introduction
Mild local hyperthermia is a promising treatment modality for various types of cancer but it is difficult to ensure reliably heating the entire tumour while reducing energy deposition in healthy tissue. Perfusion is crucial for hyperthermia treatment planning and has to be taken into account when using the bioheat equation with temperature and tissue parameters for calculating the temperature that will be reached Citation[1–4]. Moreover, perfusion is important because it dramatically changes during hyperthermia treatment. Note that the interaction between temperature and perfusion is highly nonlinear due to complex thermoregulatory effects in a patient body Citation[5], Citation[6]. Part of the beneficial effects of hyperthermia in multimodal tumour treatment are caused by changes in the microenvironment such as changes in perfusion, improvement of oxygenation, and increased chemo supply of chemotherapeutic agents Citation[6–8]. It is therefore important to have a tool for evaluation of perfusion in the context of hyperthermia treatment.
Several techniques are available today to perform perfusion imaging. Functional information on blood volume and perfusion is obtained from signal time curves of tissue whose shape is determined by these parameters and which can be extracted from images acquired dynamically after the intravenous administration of a short tracer bolus. The most important techniques providing perfusion values are 15O-water-PET (positron emission tomography), dynamic contrast-enhanced magnetic resonance imaging (DCE-MRI), dynamic susceptibility contrast-enhanced MRI (DSC-MRI), arterial spin labeling (ASL), and dynamic contrast-enhanced computed tomography (DCE-CT). Broadly speaking, perfusion measurement by all of these techniques is based on the indicator dilution theory Citation[9], Citation[10] for dynamically measuring the concentration of a chemically inert, exogenic tracer and the evaluation technique developed by Kety Citation[11], Citation[12]. The MRI techniques are the most popular because they do not use radioactivity to assess the tracer concentration. Unfortunately, the MRI techniques are also the most challenging for perfusion quantification.
Perfusion quantification
Different models and methods are used to analyse dynamic MRI data, depending on the acquisition technique used and the parameters to be determined: the simplest model is a one-compartment model of Kety which assumes that all of the contrast agent administered is in a single compartment Citation[11], Citation[12]. The single compartment might be the blood volume in the case of an intravascular tracer, or an arbitrary distribution volume in which the tracer instantaneously extravasates.
The quantification of perfusion is based on the indicator dilution theory and requires the arterial input function (AIF), Ca(t), for deconvolution of the blood concentration time curve in tissue capillaries, Cb(t), where perfusion can be described mathematically as a convolution (⊗):where F is the perfusion and R(t) is the capillary residue function, which describes the fraction of contrast agent remaining in the tissue at time t, following the injection of an ideal instantaneous bolus at time t = 0. When an intravascular tracer is administered, the vascular volume is determined quantitatively from the integral of the bolus signal Citation[13], Citation[14].
Unfortunately, only low molecular weight substances have been available until now but they extravasate the moment they reach the tissue except within the brain, where the blood-brain barrier prevents extravasation. A signal predominantly from the microvascular blood compartment is obtained after administration of a high-dose bolus of low molecular weight Gd-tracers. Moreover, extravasation is limited so that there is no instantaneous extravasation, resulting in a homogeneous tracer concentration throughout the distribution volume. The amount of extravasation is described by the extraction coefficient. When using low molecular weight substances, contrast agent extravasation and bolus signal cannot be determined separately but interfere with each other. Therefore, the blood bolus signal dominates in the first seconds in highly perfused tissues while the extravascular signal becomes dominant after a few seconds in tissues with low perfusion.
To simplify perfusion calculation two approximations are used in practice for the same tracer: earlier investigators typically neglected extravasation when assessing perfusion of brain Citation[13], Citation[14] or myocardium Citation[15]. When low-perfused tissues are studied, the flow-limited extravasation model is applied when using water for PET and low molecular weight Gd-tracers in MRI Citation[16]. In that case the intravascular blood is neglected and perfusion-limited extravasation is assumed. In the case of low molecular weight Gd-tracers the distribution volume is the interstitial volume, which is frequently denoted as leakage volume or extravascular extracellular space.
If one wishes to explicitly take into account contrast agent extravasation, the vascular compartment must be supplemented by a second compartment, the interstitial space. The two-compartment model describes contrast agent exchange between the blood and interstitium as a bidirectional process with identical velocities Citation[17] and different velocities for extravasation and reflux Citation[16].
Often, the vascular compartment is neglected, again resulting in a permeability one-compartment model with different velocities for extravasation, Ktrans, and reflux, kepve Citation[16], where ve is the extravascular extracellular distribution volume of CM. Two-compartment models are much better suited to simultaneously calculate perfusion and extravasation and avoid the errors associated with one-compartment models Citation[18–21]. They allow simultaneous quantitative determination of the vascular and interstitial volumes and their exchange parameters Citation[20], Citation[22–24].
The phenomenologic parameters for extravasation, Ktrans, and reflux, kepve, of low molecular weight tracers depend on perfusion and permeability Citation[16], Citation[17] but are not linearly correlated with perfusion and permeability. The methodical limitation of low molecular weight tracers for perfusion quantification can now be overcome by the use of intravascular tracers in combination with DCE-MRI. Intravascular tracers simplify perfusion quantification because the one-compartment model of Kety can be used for calculation.
A possible diagnostic application of DCE-MRI is tumour assessment. The growth of tumours beyond a certain size depends on the development of a vascular supply that meets the metabolic demand of the neoplastic tissue. Once a growing tumour reaches a critical mass, it induces growth of new blood vessels. Studies of human neoplasms have demonstrated that higher malignancy is associated with an increased vascularity Citation[25–27]. Gliomas, especially when of a high grade, are highly vascular. Evaluation of the microvascular structures of these tumours, including the proliferation of the endothelial cells that line the tumour capillaries, is part of most histological grading systems. The assessment of vascular proliferation might allow differentiation of tumour grades Citation[28].
DCE-MRI
Echo-planar imaging (EPI) is the standard imaging technique for dynamic susceptibility contrast (DSC) perfusion MRI and was the first perfusion imaging technique performed with MRI Citation[14]. The difference in susceptibility between the tissue and the paramagnetic material during bolus passage provides a high dynamic contrast because the susceptibility effect is spread over a larger volume than the blood volume from which it is originating. Practically, only the signal decrease is measured using a T2/T2*-weighted EPI sequence. Unfortunately EPI suffers from several technical and methodical limitations. The relatively long TEs (echo times) needed for optimal T2 contrast lead to geometric image distortions due to the low sampling band-width in the phase-encoding direction. Aside from global distortions, local areas of magnetic field susceptibility gradients, which are most egregious at air/tissue interfaces, are degraded by signal voids. Additionally, the intrinsic susceptibility contrast makes perfusion quantification difficult Citation[29] and reduces application of the imaging technique to the brain.
DSC-MRI yields good results, compared with PET, in the brain, especially when the blood-brain barrier is intact Citation[30]. In tissues with contrast agent extravasation, paramagnetic agents have opposing effects: the extravasated agent results in signal enhancement while the intravascular bolus causes a decrease in signal intensity. In this situation, neglecting the signal increase resulting from the T1-effect will increase the error in calculating blood flow and volume Citation[31], Citation[32]. This effect is especially deleterious in the presence of a highly malignant tumour, where the signal increase resulting from increased extravasation and the signal decrease due to higher vascularization cancel each other Citation[19], Citation[24], which limits the differentiation of highly malignant and malignant tumours.
DCE-MRI overcomes the intrinsic problems of the DSC-MRI technique by exploiting the T1 contrast to monitor tracer dynamics. Different MR sequences are used in DCE-MRI to assess the dynamic change of the T1 contrast but all techniques use a spoiled gradient echo sequence (vendor names: FLASH (fast low-angle shot), SPGR (spoiled gradient echo), T1-FFE (T1-weighted fast field echo)) for image read-out. Both prepared and unprepared spoiled gradient echo sequences are used for DCE-MRI.
Li et al. Citation[18] introduced a straightforward way to quantify the longitudinal relaxation rate, R1, and thus tracer concentration in a dynamic study. First, three or more 3D spoiled gradient echo images are acquired with different flip angles to quantify R1 and magnetization, M0, before introducing the tracer. Afterwards, tracer dynamics is imaged using the 3D spoiled gradient sequence with a flip angle of 30°–35°. The dynamic change in relaxation rate, ΔR1(t), is proportional to the tracer concentration and can be quantified by using a rather simple equation Citation[18].
A 3D spoiled gradient echo sequence for quantification of dynamically changing tracer concentration is rather easy to implement. Unfortunately, the signal-to-noise ratio of these sequences is substantially lower than that of prepared gradient echo sequences. On the other hand, 3D spoiled gradient echo sequences without preparation provide a larger dynamic contrast agent concentration range that can be exploited for perfusion imaging. Therefore, image acquisition with 3D spoiled gradient echo sequences requires administration of the usual dose of 0.1 mmol/kg of low molecular weight Gd-tracer to yield a good contrast-to-noise ratio Citation[18].
For dynamic acquisition with prepared gradient echo sequences, both saturation Citation[15] and inversion preparation Citation[24] are applied. The prepared gradient echo sequences provide a much higher sensitivity for Gd-tracers. Inversion preparation has the advantage that the inversion time can be adjusted to null the signal of unenhanced blood Citation[24]. In that case the complete signal change can be used by the inversion-prepared sequence which results in a larger dynamic contrast-to-noise range in comparison to the saturation-prepared sequence. Unfortunately, prepared sequences show saturation effects at much lower tracer concentrations than unprepared gradient echo sequences. To avoid saturation effects therefore, a much lower dosage is typically used to assess the contrast medium bolus, i.e., 0.025 mmol/kg Citation[15]. In that case a linear correlation between signal change and concentration can be assumed in first approximation Citation[33].
In order to improve the dynamic contrast-to-noise ratio by applying a higher tracer dosage, different solutions have been proposed to increase the sensibility of the prepared sequences. The MR signal intensity of an inversion-prepared gradient-echo sequence depends on the relaxation times, T1, T2, and T2*, and its complete description is very complex Citation[34], Citation[35]. Therefore, a simplified phenomenological function experimentally fitted with CM probes is a useful approach to convert signal to concentration and requires less calculation time Citation[24]. Another solution is to use special saturation recovery sequences, which can determine baseline T1 and magnetization by repeated acquisition with different imaging parameters Citation[36]. In principle contrast agent and imaging sequence can be chosen independently. Practically the dynamic contrast-to-noise ratio limits the choice. The distribution volume of intravascular tracers is restricted to the blood volume and therefore only the blood compartment contributes to the dynamic signal change. In highly perfused tissues such as the kidney, brain, or heart this might be no limitation but most tissues have a blood volume of only 1–2%. In consequence, using an intravascular tracer for perfusion quantification in such tissues requires a dynamically available contrast-to-noise ratio significantly larger than 100 : 1. That can typically only be achieved with prepared gradient echo sequences.
Application to hyperthermia
Perfusion quantification principally requires knowledge of the AIF (Ca(t)). Extraction of the AIF is often difficult in practice because a sufficiently large artery within the field of view has to be used. To avoid partial volume effects the diameter of the artery must be larger than the intrinsic resolution of the dynamic imaging technique applied. A sufficiently large artery is often not detected in the field of view. Different approaches are used to overcome this limitation. Van Osch et al. Citation[37] presented a method that uses the phase information of the dynamic scan to correct for the partial volume effect. Other investigators used a standardized AIF which does not take individual variation into account. Often investigators do not normalize the dynamic signal change to the AIF and measure only the tissue curve. Such tissue curves are only evaluated by descriptive parameters such as time to peak enhancement, slope, and maximal amplitude.
Failure to normalize perfusion estimation to an individual AIF is not acceptable for evaluation of perfusion changes due to hyperthermia. Note that long-term power deposition with several hundreds of watts is necessary for effective hyperthermia in the patient's body and induces strong thermoregulation effects Citation[5], Citation[6]. As the amount of blood is nearly constant during therapy, perfusion cooling is adjusted by an increase in cardiac output per minute, which is achieved by a distinct increase in heart rate Citation[38].
The impact of increased heart rate is demonstrated in showing two AIFs measured four days before and 20 minutes after hyperthermia in a peripheral artery of a 63-year-old patient with a sarcoma in the right upper leg. The measurements were performed in the hybrid system for regional hyperthermia (Siemens Symphony/BSD-2000-3D), the first without hyperthermia applicator and the second within the hyperthermia applicator. In both cases 20 ml of low molecular weight Gd-tracer (gadoteridol) was injected with the same flow rate of 4 ml/s as a bolus beginning with acquisition of the dynamic scan. The AIF is less delayed and less dispersed after hyperthermia. The modification of the AIF by hyperthermia has a large impact on perfusion determined in the tissue. demonstrates the change in relaxation rate demonstrated by the same scans in a reference muscle within the heated area. The slope of the tissue change in relaxation rate becomes much steeper. Usually, a steeper slope indicates higher perfusion when calculating perfusion using the one-compartment model normalized to the AIF. In our patient, perfusion calculated before hyperthermia using a low molecular weight tracer and the one-compartment model with delay correction was F = 26.8 ml/(100 cm3 min) and was higher than 20 min after hyperthermia with F = 20.0 ml/(100 cm3 min). If one uses the AIF of the post-hyperthermia acquisition for calculating perfusion before hyperthermia with an appropriate delay correction, one obtains a perfusion of F = 23.4 ml/(100 cm3 min). Of course, calculation using a one-compartment model when a low molecular weight tracer is administered does not provide the real perfusion value. Nevertheless, the increase in cardiac output seems to suggest that there is an increase in perfusion, which, however, is not confirmed by the calculation.
Figure 1. Two arterial input functions (A) and tissue functions (B) in units of the change in relaxation rate ΔR1 determined from a peripheral artery in a sarcoma patient 4 days before (green) and 20 min after the first hyperthermia treatment (red). The change in relaxation rate which was calcuated by substracting the baseline relaxation rate is proportional to the tracer concentration. Images were acquired with a 3D spoiled gradient echo sequence. Baseline images were acquired using flip angles of 5°, 10°, 20°, 30°, and 40°; TR = 3.64 ms, TE = 1.21 ms. The dynamic scan was acquired using the same sequence with a flip angle of 30° starting with the injection of 20 ml gadoteridol bolus over 5 s. (A) Following hyperthermia, the delay of the AIF is reduced by 9.3 s and the apparent transit time of the AIF from 12.52 s to 7.58 s. (B) The change in relaxation rate in tissue is proportional to the tracer concentration determined. Perfusion is calculated by using a one-compartment model, which provides a tendency and rough estimation of the true perfusion value. The two fit functions are indicated by dots.
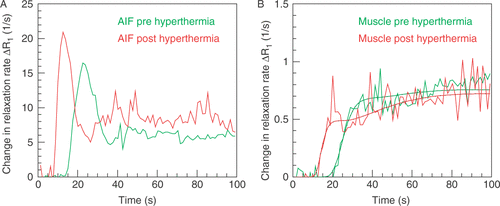
DCE-MRI might be useful to demonstrate perfusion changes in sarcoma following hyperthermia (see ). As stated earlier, it is expected that hyperthermia will induce an increase in perfusion especially in tumours. demonstrates the opposite effect. Before hyperthermia, the sarcoma consists of a large necrotic area in the tumour centre and highly perfused areas espcially in the tumour rim. After hyperthermia no perfusion is detectable in the tumour. The image suggests that a single hyperthermia treatment completely destroyed the tumour vasculature. The case nicely illustrates the immediate success of hyperthermia.
Figure 2. Same patient as in . The morphological images were acquired using a 3D gradient echo sequence with fat saturation, TE = 1.27 ms, TR = 4.66 ms. The perfusion images were calculated using singular value decomposition. Images obtained before hyperthermia are presented in the upper row and images after the first hyperthermia treatment in the lower row.
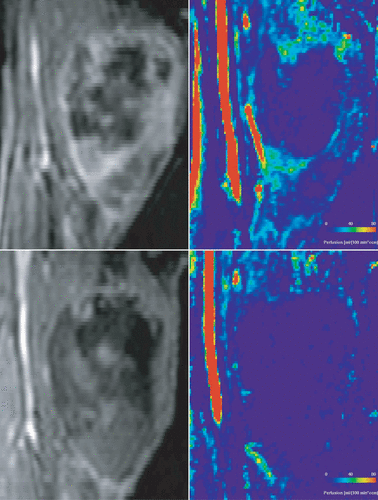
In conclusion, DCE-MRI allows assessment of hyperthermia-induced changes in perfusion while its practical implementation remains challenging. The techniqe will help us understand the role of hyperthermia in tumour treatment and has the potential to be used for monitoring hyperthermia treatment. Assuming that insufficiently perfused tissue after hyperthermia is nonviable, we can use perfusion imaging to assess the amount of viable tumour tissue as illustrated here by the case of the sarcoma patient presented here.
References
- Pennes HH. Analysis of tissue and arterial blood temperature in the resting forearm. J Applied Physiol 1948; 1: 93–122
- Langendijk JJW. The influence of blood flow in large vessels on the temperature distribution in hyperthermia. Phys Med Biol 1982; 27: 17–23
- Langendijk JJW, Schellekens M, Schipper J, van der Linden PV. A three-dimensional description of heating patterns in vascularized tissues during hyperthermic treatment. Phys Med Biol 1984; 29: 495–507
- Weinbaum S, Jiji LM, Lemons DE. Theory and experiment for the effect of vascular microstructure on surface tissue heat transfer–Part I: Anatomical foundation and model conceptualisation. ASME J Biomech Eng 1984; 106: 321–330
- Vaupel P, Kallinowski F, Kluge M. Pathophysiology of tumors in hyperthermia. Recent results in cancer research. Application of hyperthermia in the treatment of cancer, RD Issels, W Wilmanns. Springer-Verlag, BerlinHeidelberg 1988; 65–75
- Horsman MR. Tissue physiology and the response to heat. Int J Hyperthermia 2006; 22: 197–203
- Song CW, Park HJ, Lee CK, Griffin R. Implications of increased tumor blood flow and oxygenation caused by mild temperature hyperthermia in tumor treatment. Int J Hyperthermia 2005; 21: 761–767
- Dewhirst MW, Vujaskovic Z, Jones E, Thrall D. Re-setting the biologic rationale for thermal therapy. Int J Hyperthermia 2005; 21: 779–790
- Crone C. The permeability of capillaries in various organs as determined by use of the ‘Indikator Diffusion’ method. Acta Physiol Scand 1963; 58: 292–305
- Renkin E. Transport of potassium-42 from blood to tissue in isolated mammalian skeleted muscles. Am J Physiol 1959; 197: 1205–1210
- Kety SS. The theory and applications of the exchange of inert gas at the lungs and tissues. Pharmacol Rev 1951; 3: 1–41
- Kety SS. Theory of blood-tissue exchange and its application to measurement of blood flow. Meth Med Res 1960; 8: 223–227
- Rosen BR, Belliveau JW, Chien D. Perfusion imaging by nuclear magnetic resonance. Magn Res Q 1989; 5: 263–281
- Rosen BR, Belliveau JW, Vevea JM, Brady T. Perfusion imaging with NMR contrast agents. Magn Reson Med 1990; 14: 249–265
- Jerosch-Herold M, Wilke N, Stillman AE. Magnetic resonance quantification of the myocardial perfusion reserve with a Fermi function model for constrained deconvolution. Med Phys 1998; 25: 73–84
- Tofts PS, Brix G, Buckley DL, Evelhoch JL, Henderson E, Knopp MV, Larsson HBW, Lee T, Mayr NA, Parker GJM, Port RE, et al. Estimating kinetic parameters from dynamic contrast-enhanced T1-weighted MRI of a diffusable tracer: Standardized quantities and symbols. J Magn Res Imaging 1999; 10: 223–232
- Daldrup H, Shames DM, Wendland M, Okuhata Y, Link TM, Rosenau W, Lu Y, Brasch RC. Correlation of dynamic contrast-enhanced magnetic resonance imaging with histologic tumor grade: Comparison of macromolecular and small-molecular contrast media. Pediatr Radiol 1998; 28: 67–78
- Li KL, Zhu XP, Waterton J, Jackson A. Improved 3D quantitative mapping of blood volume and endothelial permeability in brain tumors. J Magn Res Imaging 2000; 12: 347–357
- Li KL, Jackson A. New hybrid technique for accurate and reproducible quantitation of dynamic contrast-enhanced MRI data. Magn Reson Med 2003; 50: 1286–1295
- Johnson G, Wetzel SG, Cha S, Babb J, Tofts PS. Measuring blood volume and vascular transfer constant from dynamic, -weighted contrast-enhanced MRI. Magn Reson Med 2004; 51: 961–968
- Haroon HA, Buckley DL, Patankar TA, Dow GR, Rutherford SA, Balériaux D, Jackson A. A comparison of Ktrans measurements obtained with conventional and first pass pharmacokinetic models in human gliomas. J Magn Res Imaging 2004; 19: 527–536
- Donahue KM, Weisskoff RM, Parmelee DJ, Callahan RJ, Wilkinson RA, Mandeville JB, Rosen BR. Dynamic Gd-DTPA enhanced MRI measurement of tissue cell volume fraction. Magn Res Med 1995; 34: 423–432
- Degani H, Gusis V, Weinstein D, Fields S, Strano S. Mapping pathophysiological features of breast tumors by MRI at high spatial resolution. Nature Medicine 1997; 3: 780–782
- Lüdemann L, Hamm B, Zimmer C. Pharmacokinetic analysis of glioma compartments with dynamic Gd-DTPA-enhanced MRI. Magn Res Imaging 2000; 18: 1201–1214
- Brem S, Cotran R, Folkman J. Tumor angiogenesis: A quantitative method for histologic grading. J Natl Cancer Inst 1972; 48: 347–356
- Weidner N, Semple JP, Welch WR, Folkman J. Tumor angiogenesis and metastasis: Correlation in invasive breast carcinoma. N Engl J Med 1991; 324: 1–8
- Macchiarini P, Fontanini G, Squartini F, Angeletti CA, Hardin MJ. Relation of neovascularisation to metastasis of non-small-cell lung cancer. Lancet 1992; 340: 145–146
- Lüdemann L, Grieger W, Wurm R, Wust P, Zimmer C. Glioma assessment using quantitative blood volume maps generated by T1-weighted dynamic contrast-enhanced magnetic resonance imaging: A receiver operating characteristic study. Acta Radiol 2006; 47: 303–310
- Troprès I, Grimault S, Vaeth A, Grillon E, Julien C, Payen JF, Lamalle L, Décorps M. Vessel size imaging. Magn Res Med 2001; 45: 397–408
- Kety SS, Axel L, Hoop B. Principles of conventional techniques. Diffusion and perfusion magnetic resonance imaging, D Le Bihan. Raven Press, New York 1995; 201–215
- Bruening R, Kwong KK, Vevea MJ, Hochberg FH, Cher L, Harsh IGR, Niemi PT, Weisskoff RM, Rosen BR. Echo-planar MR determination of relative cerebral blood volume in human brain tumors: T1 versus T2 weighting. AJNR Am J Neuroradiol 1996; 17: 831–840
- Wenz F, Rempp K, Brix G, Knopp MV, Gückel F, Heß T, van Kaick G. Age dependency of the regional cerebral blood volume (rCBV) measured with dynamic susceptibility contrast MR imaging (DCS). Magn Res Imaging 1996; 14: 157–162
- Hoffmann U, Brix G, Knopp MV, Heß T, Lorenz WJ. Pharmacokinetic mapping of the breast: A new method for dynamic MR mammography. Magn Res Med 1995; 33: 506–514
- Larsson HBW, Fritz-Hansen T, Rostrup E, Søndergaard L, Ring P, Henriksen O. Myocardial perfusion modeling using MRI. Magn Res Med 1996; 35: 716–726
- Vallée JP, Sostman HD, MacFallm JR, Wheeler T, Hedlund LW, Spritzer CE, Coleman RE. MRI quantitative myocardial perfusion with compartmental analysis: A rest and stress study. Magn Res Med 1997; 38: 981–989
- Brix G, Kiessling F, Lucht R, Darai S, Wasser K, Delorme S, Griebel J. Microcirculation and microvasculature in breast tumors: Pharmacokinetic analysis of dynamic MR image series. Magn Res Med 2004; 52: 420–429
- van Osch MJ, Vonken EJ, Bakker CJ, Viergever MA. Correcting partial volume artifacts of the arterial input function in quantitative cerebral perfusion MRI. Magn Res Med 2001; 45: 477–485
- De Leeuw AA, Van Vulpen M, Van De Kamer JB, Wárlám-Rodenhuis CC, Lagendijk JJ. Increasing the systemic temperature during regional hyperthermia: Effect of a cooling strategy on tumour temperatures and side-effects. Int J Hyperthermia 2003; 19: 655–663