Abstract
Purpose: This research was to reveal the thermal characteristics of a water-cooled microwave ablation antenna in phantom, and the influence of cooling water velocity on ablation pattern by numerical method. In addition, by comparing the numerical results with experimental results, the experimentally obtained SAR was proven to be correct.
Methods: The temperature distribution in ablations was simulated by the finite element method. In the FEM, the cooling effect in the region of the water-cooled antenna was introduced by applying the convective coefficient to the related boundaries, and the experimental determined SAR in phantom was applied as heat generation of microwave generator. To study the effect of water flow rate on ablation pattern, three different water velocities were chosen. In addition, the phantom thermal properties changes were considered in simulation when the heating temperature was above 80°C.
Results: The ablation pattern could be identified as a pear shape. The temperatures of monitoring points which were located near the antenna could rise more rapidly, and they were more likely to achieve steady heat transfer state within a short time compared with those far away from the antenna. The cooling water effectively decreased the temperature near the antenna, an under-temperature occurred in the cooling region, and the different cooling water flow rate did not affect significantly the ablation pattern.
Conclusions: The numerical results compared reasonably well with the experimental results in both heating pattern and temperature of individual monitoring point over the same heating duration. The SAR measured by our previous experiment was also confirmed by this numerical simulation. This method could be employed to study combination thermal field of multi-antennas in future work.
Introduction
Microwave (MW) ablation therapy for cancer has been widely applied in clinical therapy and its application in cancer treatment is one of the hottest subject matter Citation1. Japanese researcher Seki firstly conducted the ultrasound-guided treatment of small liver carcinoma with microwave ablation Citation2, and from then on many scholars researched this subject with satisfying results Citation3, Citation4. It aims at increasing temperature of tissues to above 54°C in order to destroy the tumor. Temperature prediction of the tumor and the surrounding tissue is important for clinical ablation. So the correct analysis of thermal field is essential to the treatment plan and the region-fitting therapy.
Two types of cooled antennas, air-cooled and water-cooled, have been used for the microwave ablation to create as large ablation patterns as possible Citation5. The water-cooled antenna has been developed relatively more fully compared with other technology, and is widely used in the clinical treatment. Firstly, cooling water could significantly reduce the temperature near the antenna, as confirmed by previous study Citation6. It avoids the carbonization near the antenna, because the coagulation region is limited by the carbonized tissues. Therefore, a water-cooled antenna makes it possible to create the maximum ablation pattern. Secondly, a water-cooled antenna could effectively reduce the adhesion between antenna and tissue. It reduces patient pain during treatment and risk of infection after the operation Citation5.
The numerical method is valuable for predicting temperature in tissue during the ablation. However, the temperature distribution due to the existence of cooling water in the antenna may be complicated, and numerical simulation of the thermal field of this specific water-cooled antenna is not sufficient on this relative subject.
The present study focused on the water-cooled antenna to quantify its thermal characters. The temperature distribution in the phantom heated by the microwave was simulated by the finite element method. The water cooling effect was included in the FEM by applying the convective coefficient. The temperature fields of different cooling water velocities were compared with experimental results.
Numerical simulation and methodology
Bioheat transfer model
The mechanism by which microwave ablation induces tissue injury is the conversion of microwave energy into heat. The Pennes’ bio-heat Citationequation 7 governs heat transfer during ablation therapy.where ρ is the density (kg/m3), c is the specific heat (J/kg°C), and k is the thermal conductivity (W/m°C). T is the temperature of tissue (°C). Qr is the heat generated by the microwave (W/m3) and Qm is heat generated by metabolism. ωb is blood perfusion (kg/m3 s), cb is the specific heat of blood (J/kg°C), Tb is the temperature of arterial blood (°C). In our present study, to compare with experimental results, the phantom was considered in the numerical simulation, therefore, ωb, Qm were zero.
A water-cooled antenna (designed by the Institute of Microwave Electronics, Nanjing, Qinghai, China) was chosen for our simulations. The microwave generator operated at a frequency of 2,450 MHz with a power output of 0–100 W. The antenna diameter was 1.9 mm. The antenna geometric model can be seen in .
A serial experimental study has been done on this antenna by our previous work Citation8, and the distribution of the specific absorption rate (SAR) has also been obtained. In the experiment, thirty-two thermocouples (TCs) were used to measure the transient temperature field from which the SAR distribution was determined (). The TCs were placed in the same plane with the antenna in the . Axis r was along the radial direction and axis z was oriented along the longitudinal axis of antenna. The SAR could be expressed as Equation 2 and shows the fitted coefficients.
Table I. Fitted coefficients of Equation 2.
Geometric model and boundary conditions
In this study, considering the symmetry of the SAR and the temperature field induced by the antenna, the geometric model was axis-symmetrical. The dimensions can be seen in . A 1.9 mm diameter antenna was immersed in the middle of the phantom. There was current of water within the antenna. The microwave generator was located at the coordinate origin (center of geometric model). Where z = 0 represented the position of antenna generator, z > 0 and z < 0 respectively represented the backward direction of the antenna with cooling water and forward direction without water. Four monitoring points were chosen to study the thermal distribution and to compare temperatures with the experimental data Citation8. The monitoring points were labeled 3, 4, 5, 12 respectively, in accordance with the experimental TCs, and the distances between two neighboring points were 4 mm (in r axis) and 5 mm (in z axis). The location of monitoring points respectively were 3 (4, −5), 4 (4, 0), 5 (4, 5) and 12 (8, 0).
Figure 3. Geometric modeling. A coaxial slot antenna was inserted in the phantom along the z-axis. The radius of the antenna was 0.95 mm. The dimension was directly labeled and every boundary condition was named as L1 ∼ L5.
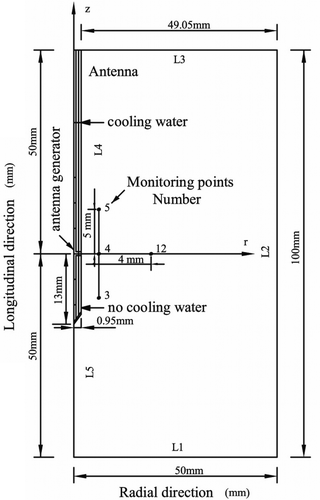
Constant temperature boundary conditions were used at boundaries (L1, L2, L3). The thermal boundary was maintained at 22°C as the ambient air temperature, based on the assumption that the boundaries were far enough not to be affected by the heating power. L5 was the symmetrical boundary condition, ∂T/∂r = 0. The microwave generator operated at 2,450 MHz with a power output of 60 W and the corresponding SAR distribution was used as volumetric heat generation rate. At L4, where the water-cooled antenna was located, the convective boundary condition was given. The convective coefficient h was calculated based on the Nusself number of the water flow rate Citation9. So the convective coefficient was given as 1,876 W/m2°C in accordance with the cooling water flow rate of 0.22 m/s. In addition, in order to investigate the ablation pattern variation with the water flow rate, velocities of 0.44 m/s and 0.66 m/s were also performed. And the convective coefficients respectively were 2,430 W/m2 °C and 2,741 W/m2 °C.where Nu = Nusself number, h = convective coefficient. λ = water thermal conductivity, 0.599 W/m°C; l = actual water cooling length, 150 mm; d was the antenna diameter, 1.9 mm; ν was the cooling water velocity; υ was the water viscosity, 1.006 × 10−6 m2/s; Pr was Prandtl number, 6.12. The Nu and h of the different velocities are given in .
Table II. Nu and h of the different velocities.
Material properties
A muscle-equivalent microwave phantom was used in this study. The thermal properties of the phantom were selected according to the study by Leonard and Jiang et al. Citation10, Citation11, Citation12. Various ingredients of this phantom are listed in .
Table III. Phantom ingredients (%).
Thermal parameters included the thermal conductivity, k = 0.535 W/m°C; the density, ρ = 1070 kg/m3; and the heat capacity, c = 3700 J/kg°C, under normal temperature.
In most cases, the thermal properties would change when the heating temperature was above 80°C Citation13, which would directly affect the heat conduction within the phantom. However, there were not enough references to find the thermal parameters of this material above 80°C. Generally speaking, it was difficult to measure the thermal properties because they were temporally and temperature dependent. Major contributions to the temperature dependence of the properties had been reported Citation14, Citation15, and the material properties changed rather complicated due to the nature of dehydration in the high temperature. Although there was a significant amount of information about the effect of temperature on the properties, the parameters were normally considered to be a constant in the existing modeling. To obtain an accurate numerical result, it was necessary to give a quantitative relationship between temperature and thermal parameters in simulation.
In this paper we introduced a simple relationship between thermal parameters and temperature into our numerical model to improve the accuracy of results. A simple assumption was proposed that material properties changed with temperature. For temperatures above 80°C, it was assumed that the thermal properties of the phantom were decided by polyethylene, which was the major component of this phantom. Then the polyethylene's parameters were defined in the simulation to replace the phantom's parameters when temperature was above 80°C. These parameters including specific heat and thermal conductivity came from the references Citation16–18. shows the assumed thermal properties with the temperature.
Table IV. Physical parameters of the phantom.
Methodology
The multi-physics simulation tool ANSYS9.0 was chosen in present work. It can provide all the elements necessary to build the model, to solve the problem and post-process the results. The geometric model was meshed with an axially symmetric element to construct the finite element model. For accurate results, the element meshing was refined near the generator. Meshing criterion independence was assessed by comparing the temperature distribution in the final results. After increasing the elements by 10%, the temperature changed by less than 1%. Then the meshing was believed reasonable. Finally, the finite element model consisted of 5100 (4-node plane 55) elements and 5253 nodes in total, which ensured the validation of the numerical results. By using the numerical method, temperature distribution was calculated under the same conditions as the experiment. To study the effect of cooling water velocity on ablation pattern and size, three different of water velocities were chosen in the experiment. The cooling water velocities (flow rate) were respectively adjusted to 0.22 m/s, 0.44 m/s and 0.66 m/s by a peristaltic pump in the experiment. Based on these experimental data, different heat transfer coefficients in accordance with the water velocities, were applied to simulate the cooling effects in our simulation. The calculation was a transient process and initial temperature was set to 22°C. For this study, it took about 60 steps to simulate a heating duration of 120 s. The initial and finial time steps were 2s.
Numerical results
The temperatures of the chosen monitoring points are shown in . It can be seen that the highest temperature appeared at the monitoring point 3 which was located in the front of the antenna, and its temperature rose more rapidly than those of others. The temperatures near the antenna (3, 4, 5) increased more quickly than those far away from the antenna (12).
showed the ablation pattern (54°C contours) at 60 W with cooling water velocity of 0.22 m/s at the time of 120 s. The map was generated by the ANSYS package, and the monitoring points (3, 4, 5, 12) were set as finite element node. It can be seen that coagulation necrosis appears more likely to be a pear shape. The temperature around the rear antenna with cooling water was decreased. Because there was no water cooling, the ablation pattern in the region where z < 0 was larger in diameter than that in the region where z > 0.
Numerical and experimental results comparison
In order to verify the accuracy of the numerical model, the ablation pattern and size was compared between numerical and experimental results. We also compared numerical results of the phantom temperature with experimental results under the same condition of cooling water velocity of 0.22 m/s. In addition, time-dependent temperature was compared with experimental results, and an R-sq was used to verify the accuracy of simulation.
Results of transient temperature
shows the temperature of monitoring points in the experiment and the numerical simulation at the flow velocity of cooling water for 0.22 m/s. It can be seen that the results of the simulation compared reasonably well to the experimental results in temperature profiles over the same time range.
These temperature curves of the monitoring points followed different laws along the radial direction and longitudinal direction. The temperature of monitoring point 3, which was located in the front of the antenna, increased fastest among the four monitoring points, and it reached the maximum temperature compared with other points due to no water cooling effect in the region (z < 0). Additionally, it can also be seen that at these points temperature (3, 4, 5) increased quickly at the beginning time, and they were more likely to reach a steady heat transfer state within a short time compared with point 12. Temperature of point 12 rose slowly and it took a longer time to reach steady state. showed the R-sq value of the numerical and experimental temperature. The statistical analysis showed that there was no significant change in temperature between numerical and experimental results.
Table V. R-sq between simulation and experiment.
Results of ablation pattern
shows the ablation patterns (54°C contours) at 60 W in the simulation, and the experiment with cooling water velocity of 0.22 m/s at different times. The experimental ablation pattern drawn using software Table curve according to temperatures measured at different sites. Firstly, it can be seen that the ablation pattern had a pear-like shape, which enlarged with heating time. Secondly, there was an obvious under-temperature region on the ablation pattern in the back of the antenna with cooling water.
The ablation pattern can be seen in . The r-axis ablation length was about 11.5 mm, z-axis ablation length was about 29 mm at the heating time of 120 s in simulation.
Table VI. Comparison of the coagulation region.
shows how water velocity influenced the thermal field in the numerical simulation. The cooling water velocities were chosen as 0.22 m/s, 0.44 m/s and 0.66 m/s from the experiment. The ablation pattern was also set to 54°C contours. It was shown that there were no significant changes for the ablation patterns with different cooling water velocities, but in the region of z > 0 there was a little change.
Discussion and conclusion
This study investigated the thermal distribution and ablation patterns of the water-cooled microwave ablation antenna. Water cooling effect was included in the employed FEM by using the convective coefficient. The temperate distribution and coagulation necrosis were calculated under the different cooling water velocities.
The numerical results agreed well with the experimental data in both ablation pattern and monitoring point's temperature. This method was found to be suitable for predicting temperature distribution during microwave ablation. The SAR measured by our early work was also confirmed by this simulation. Therefore, the SAR can be applied in this phantom model for this particular antenna. Furthermore, a combination temperature field of multi-antennas could be simulated by this method, and it would provide technical support for region-fitting therapy and surgical planning.
The limitation of the present work was the definition of phantom properties. As we know, it was an obvious fact that the phantom properties would change with temperature, including thermal conductivity, specific heat, and density. However, it was most difficult to choose appropriate thermal parameters because there were not enough references with available data for the phantom properties changes. The numerical results with constant thermal properties had a great error compared with the experimental results. So an assumption was proposed to use polyethylene parameters instead of phantom parameters when the temperature was above 80°C. Numerical results proved that this assumption was reasonable. If not, the numerical results could not match well with experimental results (see ).
Figure 9. Comparison of numerical results (original parameters and revised parameters) with experimental results at the flow velocity of 0.22 m/s (monitoring point 3)).
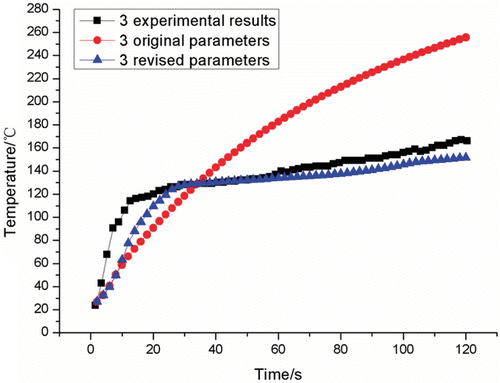
In our future work, knowledge of the phantom properties is also absolutely necessary and essential in the simulation. Multi-antenna thermal field simulation should be conducted in our later work.
Acknowledgements
This research is supported by National Science Foundation of China under grant numbers 10872013, 10772010 and 30470450.
Declaration of interest: The authors report no conflicts of interest. The authors alone are responsible for the content and writing of the paper.
References
- Liu AH, Sun KN, Li AM. Progress in mechanism and methods of tumor thermal therapy. Prog Mod Biomed 2006; 11: 105–108
- Seki T, Wakabayashi M, Nakagawa T, Itho T, Shiro T, Kunieda K, Sato M, Uchiyama S, Inoue K. Ultrasonically guided percutaneous microwave coagulation therapy for small hepatocellular carcinoma. Cancer 1994; 74: 817–825
- He W, Du HT, Feng BH, Zheng YC, Yu F. Experimental study and clinical application of US-guided microwave in the treatment of advanced liver cancer. Chinese J Med Imag Tech 2001; 17(7)607–608
- Jiang Y, Sheng ZP, Zhang JP, Zhong YD. Clinical application of ultrasound-guided microwave coagulation on treatment of liver carcinoma. Chinese J Ultrason 2002; 11(4)206–208
- Xiao QJ, Liang P, Wang ZL, Yu DJ, Yu XL. Study on thermal field of microwave ablation with internally gas-cool antenna. Biomed Eng Clin Med 2007; 11(1)5–8
- Zhang CM, Zhang J, Wang JX, Li J, Su J. Experimental study on water circulative-cooling microwave electrode's coagulating power on liver tissues. Chinese J Ultrasound Med 2006; 22(1)8–10
- Pennes HH. Analysis of tissue and arterial blood temperature in the resting human forearm. Appl Physiol 1948; 1(2)93–122
- Liu Y, Yang X, Nan Q, Xiao J, Sun MY, Hua W, Mao DS. Phantom experimental study on microwave ablation with a water-cooled antenna. Int J Hyperthermia 2007; 23(4)381–386
- Dai GS. Heat transfer, 2nd. High Education Press, Bei Jing 1999; 135
- Jiang HB, Hao J, Li AH, Sun MY, Hua W, Mao DS. Microwave phantom muscle tissue. Chinese J Biomed Eng 1992; 11(3)199–203
- Leonard JB, Foster KR, Athley TW, et al. Thermal properties of tissue equivalent phantom materials. IEEE Transactions on Biomedical Engineering 1984; 31: 533–536
- Bini MG. The polyacrylamide as a phantom material for electromagnetic hyperthermia studies. IEEE Transactions on Biomedical Engineering 1984; 31: 317–322
- Liang P, Dong BW, Yu XL, Yu DJ, P JS, Nan Q, Wang HJ, Cheng ZG, Su L. Study on thermal field during ultrasound-guided implanted microwave coagulation in phantom. Chinese J Ultrasonography 2002; 11(4)236–239
- Diller K, Valvano J, Pearce J. Bioheat transfer. CRC handbook of thermal engineering, F Kreith. CRC Press, Boca Raton 2000
- Duck F. Physical properties of tissue. Academic Press, London 1990; 9–42
- Chang YF, Li CQ, Wei WX. Measurement of specific heat of propellants by differential scanning calorimetry. J Beijing Inst Tech 1985; 3: 12–22
- Wunderlich B, Dole M. Specific heat of synthetic high polymers. VIII. Low pressure polyethylene. J Polymer Sci 1957; 24: 201–213
- Zhang J, Zheng CR, Feng JM. Survey of thermal conductivity property of polymer. Polymer Bull 2001; 6: 54–59