Abstract
Thermal ablation is increasingly being utilised in the treatment of primary and metastatic liver tumours, both as curative therapy and as a bridge to transplantation. Recent advances in high-powered microwave ablation systems have allowed physicians to realise the theoretical heating advantages of microwave energy compared to other ablation modalities. As a result there is a growing body of literature detailing the effects of microwave energy on tissue heating, as well as its effect on clinical outcomes. This article will discuss the relevant physics, review current clinical outcomes and then describe the current techniques used to optimise patient care when using microwave ablation systems.
Introduction
Primary liver cancer is the sixth most common malignancy worldwide with approximately 782,000 new cases and 745,000 deaths annually [Citation1]. Hepatocellular carcinoma (HCC) accounts for 85% of all primary liver malignancies. In addition, the liver is a common site for metastatic disease, particularly from gastrointestinal primaries such as colorectal cancer. Current treatment guidelines recommend surgical resection or transplantation as the gold standard for treating patients with very early stage or early stage HCC [Citation2,Citation3]. Resection is also considered standard of care for select patients with liver metastases. However, the majority of patients who are diagnosed with liver malignancies are not eligible for resection or transplantation due to inadequate functional liver function, multifocal or advanced disease, prohibitive tumour location or the presence of medical co-morbidities [Citation4]. As a result, thermal ablation is becoming increasingly utilised in the treatment of primary and metastatic liver tumours, both as destination therapy and as a bridge to transplantation.
The goal of thermal ablation is to heat malignant tissues to temperatures that can induce immediate coagulative necrosis (typically over 60 °C). A complete treatment encompasses the target tumour plus a 5–10-mm margin (analogous to a surgical margin) while sparing healthy parenchyma and vulnerable non-target structures [Citation5]. However, effective treatment can be difficult to achieve in the liver where high tissue perfusion and large blood vessels can act as ‘heat-sinks’ near the ablation zone [Citation6]. Such heat sinks can lead to sub-lethal temperatures and sparing of malignant cells, thereby increasing the likelihood of local tumour progression (LTP) [Citation7]. Optimal treatment is therefore a function of appropriate device placement, sufficient energy delivery, and verification of ablative margins.
During radiofrequency ablation (RFA), the most common thermal ablation modality worldwide, electrical current is passed through the tumour and adjacent tissues to generate heat [Citation8]. As tissue temperature rises beyond cytotoxic levels, desiccation precipitates a high electrical impedance that disrupts the electrical circuit [Citation9]. Passive thermal conduction facilitates heat distribution into the peripheral ablation zone. While RFA has demonstrated good results against liver tumours up to 3 cm, the combination of inefficient heating physics in conventional RFA systems and highly vascularised tissue has made effective treatment of liver tumours over 3 cm challenging [Citation10].
Microwave ablation (MWA) does not suffer from the same limitations. Microwave systems utilise an alternating electromagnetic field at 915 MHz or 2.45 GHz that is capable of propagating readily through a variety of biological material including charred and desiccated tissue [Citation11]. Heat is generated as the alternating field interacts with tissue water and ions, leading to larger ablation zones than RFA in well-perfused organs [Citation12]. Despite these theoretical advantages, treatment of large liver tumours was not possible with early generation MWA devices due to issues with antenna shaft heating that precluded higher power delivery or extended treatments [Citation13]. More recently developed microwave systems are characterised by more efficient antennas and shaft cooling with either water or gas that enables greater power delivery [Citation14–16].
This manuscript reviews the relevant physics related to MWA and effects on tissue heating. We then review the current clinical outcomes from existing clinical MWA trials. Lastly, we address how physicians leverage the most recent MW advances and technologies to improve clinical outcomes.
Physics of microwave heating
During MWA, a coaxial antenna is used to deliver high frequency (915 MHz or 2.45 GHz) electromagnetic fields into the target tumour. The rapidly alternating electric field causes water and other polar molecules to rotate in an attempt to realign with the electric field. This realignment process generates kinetic energy in the tissue, raising temperatures well over 100 °C and causing tissue near the antenna to desiccate and char [Citation11]. Electromagnetic fields from MW energy are capable of continuous transmission through this desiccated and charred tissue. As a result, MWA systems can create larger ablation zones than RF systems, even in the presence of large and fast-flowing blood vessels [Citation12,Citation17].
The rapid and efficient heating of MWA systems causes local tissue changes that have not been previously appreciated with other ablation modalities. Such changes include water vaporisation, tissue contraction and large-scale desiccation. These changes can be observed on CT and ultrasound imaging. An understanding of these changes is important when evaluating MWAs, both immediately after the procedure and on follow-up imaging.
Water vaporisation
The ability to generate temperatures well above 100 °C causes water in the tissue to undergo a rapid phase change into water vapour [Citation17,Citation18]. Water vapour disperses by pressure gradients from the centre of the ablation zone, where the heat generation is highest, to the periphery of the ablation zone, either in the liver parenchyma or nearby vasculature. During this time, bubbles in the ablation zone create backscatter that can be appreciated on B-mode ultrasound imaging [Citation19]. The distribution of hyperechoic bubbles closely approximates that of the developing ablation zone [Citation5]. Therefore, the creation and subsequent growth of MWAs should be monitored in real-time using ultrasound imaging (at least on the side of the ultrasound transducer). Water vapour can also be observed on CT during or immediately after the MWA. On CT the water vapour appears as a low attenuation area against the background liver parenchyma. Optimising imaging tools to monitor the creation and movement of water vapour during MWA is currently an active area of research [Citation20–22].
Tissue contraction
One metric of technical success during thermal ablation is to determine whether or not the volume of ablated tissue exceeds that of the target tumour plus the desired ablative margin [Citation5]. Ablation device vendors often supply data sheets detailing the anticipated size of an ablation zone given a specific time and power setting. Physicians use these data sheets to treat a target tumour of a certain size with the settings suggested by vendors. However, the majority of these data sheets are compiled from data collected in normal ex vivo animal tissue, which lacks both perfusion and tumours. As a result, these data sheets may not be particularly illustrative for human tumours [Citation23].
One explanation for this lack of concordance between ex vivo models and results in human tumours is the large amount of tissue contraction observed during MWA. Tissue contraction is more pronounced during MWA compared to other thermal ablation modalities, which produce comparatively lower tissue temperatures and less water vapour [Citation9,Citation17]. Ablation zone measurements performed on post-ablation imaging or specimen dissection that do not account for tissue contraction may underestimate the true volume of tissue destruction by up to 50% [Citation14,Citation18,Citation24]. This finding likely extends to ablation vendors’ power–time tables, where the ablation sizes are also likely underestimated. Understanding the potential for error using these tables can prevent overtreatment of tumours located near critical non-target structures.
Current literature on treating primary and secondary liver cancer
Adoption of MWA systems has previously been limited by technical problems associated with suboptimal power handling, large antenna diameter, antenna shaft heating and unpredictable heating patterns. Despite these limitations, the results of clinical MWA studies have historically compared favourably with those of RFA. In a recent meta-analysis, RFA and MWA had similar 1–5-year overall survival, disease-free survival, local recurrence rate, and adverse events. However, MWA demonstrated a superior 6-year overall survival [Citation25]. As MWA technology has advanced and our understanding of MW energy delivery has improved, MWA systems have become increasingly utilised in interventional oncology practices.
Primary liver cancer
The largest clinical experience using MWA to treat HCC comes from China, in part due to early adoption of MWA systems available only in Asia. That multi-centre study of 1007 patients with primary liver cancer showed a technical success of 97.1% (1276/1363) and a local tumour progression rate of 5.9% (78/1363) with a mean follow-up time of 17.3 months (range 3–68.9 months). The 1-, 3- and 5-year overall survival rates were 91.2%, 72.5% and 59.8%, respectively [Citation26]. In the USA, a large multicentre trial involving 455 patients with both primary and secondary liver cancers demonstrated a primary technique effectiveness rate of 97.0% (839/865) and overall local tumour progression rate of 6.0%. In that study, MWA was used to treat 139 HCCs and the LTP rate was 10.1% for HCC specifically. Tumour size greater than 3 cm was a significant predictor for decreased recurrence-free survival [Citation27].
According to the Barcelona Clinic liver cancer (BCLC) guidelines, a single HCC less than 5-cm in diameter or three HCCs that are each less than 3-cm in diameter should be treated with curative intent using an ablative technique as long as there are no extrahepatic metastases or portal vein invasion. For HCCs that meet these criteria, primary technique effectiveness is high (93–99%) and LTP rates are low (5.2–10.2%) [Citation26,Citation28,Citation29]. A summary of overall survival rates and local tumour progression from multiple studies can be found in .
Table 1. Summary of current literature on treatment of liver malignancies using microwave ablation systems.
The recent introduction of high-powered MWA devices and multiple-antenna strategies have allowed physicians to treat tumours larger than 3 cm or those that fall outside of the BCLC early stage criteria () [Citation30]. For example, a recent single-centre study of 75 patients treated with a high-powered, gas-cooled multiple-antenna MWA system showed primary technique effectiveness of 93.7% in tumours 4 cm or smaller, and 75% in tumours greater than 4 cm, with only minor complications [Citation31]. Reported local tumour progression rates are higher for tumours larger than 3 cm while overall survival rates have been comparable between the two groups [Citation32,Citation33].
Figure 1. An 84-year-old woman with a history of cirrhosis and hepatitis C. (A) Contrast-enhanced computed tomography (CECT) image demonstrates an arterial-enhanced lesion in segment IV measuring 4.0 cm (arrow). (B) Lesion was treated with the placement of a single cooled microwave antenna at 40 W for 15 min. (C) A 24-h post-procedural CECT demonstrates the presence of a thick hypervascular peri-ablation halo (triangles). This finding can be seen from the hyperemic reaction that regularly develops around an ablation zone. (D) Four-year follow-up of an arterial-phase (left) and portal-venous phase (right) CECT demonstrates contraction of the ablation zone (arrow) with no evidence of enhancement.
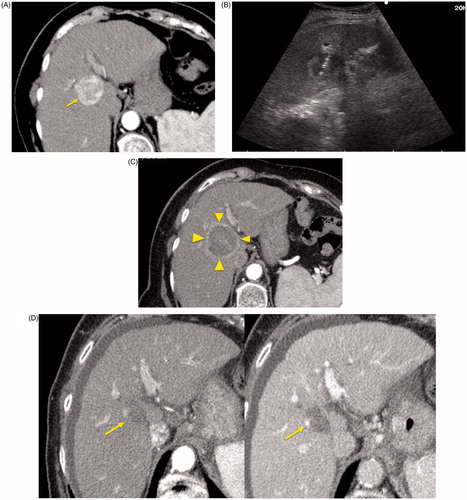
Liver metastases
MWA of liver metastases is reserved for patients who are not candidates for surgical resection or have failed other therapies. A consensus guideline was developed recently to address the indications for MWA in these cases [Citation3]. The liver metastasis should be less than 3 cm in diameter but may reach up to 5 cm depending on anatomical location (). Clinical studies focusing on MWA of metastatic liver disease are limited and usually grouped with primary liver cancer data [Citation34]. Clinical outcome studies for metastatic liver disease after ablation have been similar to those of primary disease, with local tumour progression rates ranging from 9.6–14.5% [Citation35–37]. Overall survival rates are difficult to compare with surgical results as patients referred for ablation are generally not surgical candidates and often have significant medical co-morbidities.
Figure 2. A 67-year-old woman with liver metastasis from colorectal cancer. (A) Pre-ablation MRI (left) of liver lesion (arrow) and CEUS (right) confirming presence of 13 × 14 mm liver lesion (arrow). (B) The lesion was treated with a single water-cooled antenna at 50 W for 6 min (left). Post-ablation image demonstrating hyper-echoic region measuring 34 × 37 mm, representing the ablation zone (right). The goal is to create ablation margins >1 cm beyond tumour boundary. (C) Post-ablation B-mode ultrasound (left) and CEUS (right) showing the ablation zone (anechoic) with the presence of the hypervascular peri-lesional halo. (D) CECT (left) and CEUS (right) demonstrating ablation zones that encompass the entire lesion measuring 32 × 42 mm.
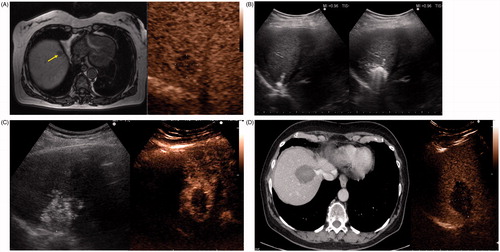
Combination therapies
Transcatheter arterial chemoembolisation (TACE) has been the preferred treatment option for patients with intermediate stage HCC. Unfortunately, recurrence rates associated with TACE monotherapy for intermediate stage HCC remain higher than both resection and ablation [Citation38,Citation39]. There is an increasing interest in combining TACE with ablation to reduce the risk of residual or recurrent tumour at the treatment site. While the precise indications are presently unclear, the authors have considered combined embolisation and ablation approaches for patients with larger tumours (>3–4 cm), infiltrative tumours or tumours that are poorly visualised on CT and ultrasound. Hepatic blood flow can be reduced with TACE to increase the rate of heating and MWA size. Furthermore, the chemotherapeutic agent mixed with lipiodol is hypothesised to increase the sensitivity of tumour cells to elevated temperatures, enhancing the efficacy of the ablation [Citation40]. Recent studies have demonstrated that patients with large, unresectable HCC experienced significantly longer survival with combination therapy using both MW and TACE when compared with patients who had undergone either TACE or MW alone [Citation41,Citation42]. There are limited data comparing different combined embolisation and ablation strategies. One recent study showed similar safety and efficacy when comparing TACE-RF and TACE-MW for the treatment of HCC [Citation43].
Complications
MWA has a safety profile similar to that of RFA in the liver [Citation35,Citation44,Citation45]. Two large retrospective MWA studies from Italy and China showed complication rates of 2.6% and 2.9% respectively [Citation46,Citation47]. Major complications have included bile duct injury, haemorrhage, liver abscess, colon perforation, skin burns and tumour seeding. Low-grade fever and post-procedural malaise are common syndromes immediately after ablation [Citation48]. The advent of shaft cooling mechanisms has substantially reduced the risk of inadvertent anatomical burns along the probe track. In one study, complication rates dropped from 3.9% to 1.6% after the introduction of water-cooled antennas [Citation46]. Improvements in shaft cooling, either with water or gas, will continue to minimise complication rates [Citation15].
Bowel perforation is a rare but potentially catastrophic complication that can result from unintended thermal damage to bowel in proximity to the target tumour. Retrospective studies with MWA report an incidence ranging from 0.1–0.7% [Citation46,Citation47]. Risk factors for bowel perforation from thermal damage include previous abdominal surgery or chronic cholecystitis leading to fibrotic adhesions between the bowel and liver. Increasing experience with MWA and utilising either hydrodissection or laparoscopic approach can virtually eliminate these complications.
Vascular injuries also remain a concern with the high heating rates associated with MWA [Citation49,Citation50]. Physicians need to consider the risk between aggressive treatment to achieve an adequate ablative margin and reduce rates of local tumour progression while minimising the risk for hepatic infarcts or intra-hepatic haematomas. The majority of vascular damage results in asymptomatic thrombosis in smaller hepatic vasculature [Citation31,Citation47]. Vessel patency during MWA is likely related to a combination of blood vessel flow, vessel size and the amount of energy deposited into the tissue [Citation6]. In vivo studies have shown that vessels smaller than 3 mm in diameter encompassed by the ablation zone are likely to thrombose during MWA [Citation51]. Additional pre-clinical studies suggest that hepatic arteries, which serve as the main blood supply to HCC, are less likely to thrombose due to their high flow states [Citation52].
Current techniques for treating liver cancer using microwave ablation systems
Image guidance
Percutaneous MWAs can be performed using ultrasound and/or CT for imaging guidance. The two modalities are complementary and often used together to assess technical success and treatment efficacy during follow-up. Ultrasound has the advantage of real-time guidance for targeting, applicator placement and monitoring the development of the ablation zone (). This can be especially helpful given that applicator placement is not confined to the axial plane. Ultrasound contrast agents can also be used to increase the conspicuity of the tumours during applicator placement and to assess for residual tumour immediately after ablation [Citation53,Citation54]. Contrast-enhanced ultrasound imaging allows for vascular enhancement patterns to be evaluated in real-time. The higher temporal resolution compared to other imaging modalities increases sensitivity to post-ablation patency of nearby tumour vasculature, indicative of residual viable tumour. The safety profile of ultrasound contrast agents has also been well documented, making it a safer imaging modality for patients with impaired renal function. However, there are limitations with ultrasound, particularly with regard to its dependence on user experience and penetration depth in overweight patients.
Figure 3. A 79-year-old man with a history of cirrhosis and hepatitis C and prior treatment with surgery and radiofrequency ablation for HCC. (A) CECT (left) and CEUS (right) images demonstrating an arterially enhanced liver lesion (yellow arrows). (B) Ultrasound image showing single gas cooled-antenna (arrowhead) being guided into the HCC (left) and after being treated at 50 W for 5 min (right). This ultrasound image shows progression of treatment via rapid generation of gas inside the liver tissue (arrow). (C) On post-procedural B-mode (left) and CEUS (right) (24 h post-ablation), the image shows presence of a central hypo-echoic area corresponding to the lesion treated, surrounded by a hyper-echoic area which corresponds to the inflammatory region of the ablation zone. (D) A 24-h post-ablation arterial phase CECT (left) and portal-venous phase CECT (right) showing the ablation zone encompassing the lesion (arrows).
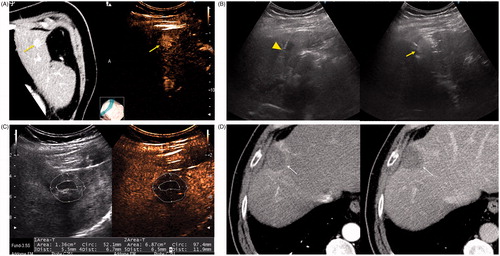
Real-time fusion imaging can also be used, particularly if tumours are not visible by US or contrast-enhanced ultrasound [Citation55]. Fusion imaging involves overlaying or displaying side-by-side real-time US images onto a previously acquired CT or MRI during the ablation procedure [Citation56]. Changes in US transducer positioning or imaging are reflected on the CT or MRI, giving physicians a more accurate view of the heating zone in relation to the nearby anatomy. Liver tumours smaller than 3 cm in diameter and difficult to identify with US alone may be targeted more easily via fusion imaging and reduce the chances of incomplete ablations [Citation57,Citation58].
After the ablation procedure an immediate post-procedural biphasic CT for HCC and hypervascular metastasis, or a single-phase CT for CRC metastasis should be performed to assess for complications and residual tumour or incomplete ablation coverage. This exam can function as a baseline study for follow-up imaging studies when evaluating for local tumour progression, either with contrast-enhanced CT or MRI [Citation59].
Hydrodissection
High-powered MWA systems can create ablation zones exceeding 4 cm with internal temperature exceeding 150 °C, especially systems with multiple-antenna capability [Citation15,Citation31,Citation60]. These high temperatures and large sizes can inadvertently damage non-target structures, especially in peripheral hepatic tumours that often lay adjacent to the bowel, abdominal wall or diaphragm. Thermal damage to these structures can cause significant post-procedural pain, while bowel injuries can lead to life-threatening perforation and sepsis [Citation47].
The most common method to minimise the risk of these complications is to utilise physical displacement strategies. This involves injecting fluid such as 5% dextrose or 0.9% saline into an adjacent potential space, pushing the ablation zone away from the vulnerable structure [Citation61,Citation62]. A drawback of using these low-viscosity fluids is a propensity to diffuse away from the injection site towards a dependent location before the ablation procedure is complete. In such cases one option of maintaining that barrier is to continuously infuse fluid during the entirety of the case. However, this can lead to fluid overload and abdominal distension requiring paracentesis or follow-up surveillance.
Recently several authors have been investigating other displacement substances such as hyaluronic acid or poloxamer gel that have a longer dwell time at or near the injection site. Hyaluronic acid, packaged as a highly viscous gel, has been used to separate bowel from the liver surface during ablation to prevent more superficial liver ablations [Citation63]. Thermo-reversible poloxamer gels designed to increase in viscosity at high temperatures have shown promising results in pre-clinical studies [Citation64]. Poloxamer gel can be doped with contrast agents to appear more visible during imaging for antenna guidance and ablation monitoring [Citation65,Citation66]. Improving the gel’s pharmacokinetics and stability presents an opportunity for investigation and its adoption will minimise the risk for burns to non-target anatomy.
Laparoscopic approach
The majority of existing clinical literature describing laparoscopic ablations have utilised RFA as opposed to MWA technology [Citation67–69]. However, the principles of thermal ablations in laparoscopy remain the same. Laparoscopic approaches can be utilised to identify safe antenna insertion paths to avoid critical structures near the liver such as the bowel or diaphragm [Citation67,Citation70]. Furthermore, laparoscopy can provide access for intraoperative ultrasound imaging, which improves lesion detection and more accurate antenna positioning. Early laparoscopic studies using RFA on small, early-stage HCCs has been associated with similar survival rates compared to surgical resection, albeit with higher rates of local tumour progression [Citation69]. Comparison studies between laparoscopic and percutaneous ablations have shown that a laparoscopic approach is associated with decreased rates of LTP in subcapsular tumours by allowing for more aggressive treatment without risk of burning adjacent organs, diaphragms and abdominal wall [Citation10]. As a result, select institutions have recommended laparoscopic ablations as the first-line treatment for patients with subcapsular liver tumours [Citation68].
Energy delivery optimisation
The primary reason for local tumour progression is the failure to create an ablation zone that completely covers the tumour and a margin [Citation71]. In many cases, multiple overlapping ablations may be required to create the necessary ablation zone size. Overlapping ablations can be created in different ways: (1) multiple insertions of a single antenna to create overlapping ablation zones in a sequential manner or (2) multiple antennas ablating simultaneously to create a confluent ablation zone. Sequential antenna applications performed by repositioning a single antenna can be technically challenging. Prior ablations create charring, bleeding, tissue contraction and gas bubbles, which can make visualisation of the remaining tumour and margins difficult. Simultaneous antenna activation using multiple antennas has been demonstrated to create larger, rounder and more confluent ablation zones in a fraction of the time due to the higher heating rate compared to sequentially activated antennas [Citation60]. This effect was seen even when controlled for total energy delivery over the ablation period.
Recent investigations have also focused on the method of energy delivery. Pulsing a higher peak-power through a single antenna can help overcome heat-sinks and create larger ablation zones than delivering the same energy using a lower, continuous power [Citation72,Citation73]. A more recent in vivo study found that peak power delivery, rather than ablation time or duty cycle, was the chief determinant in overcoming blood perfusion, highlighting the importance of utilising high-powered MWA systems [Citation74]. Conversely, treatment using low powers for long periods of time may be considered sub-optimal for creating sufficient necrosis in highly perfused tissue such as the liver. The pulsing concept can also be extended into multiple-antenna applications when multiple generators are not available [Citation75].
Protecting non-target structures using antenna orientation
The size and shape of an ablation zone and its position relative to the antenna depend not only on the input power and ablation time, but also on the design of the antenna. Ablation zones grow radially outward from the antenna. The distance that an ablation zone extends beyond the tip of the antenna will vary for different systems and antenna designs; however, ablation zones generally extend only a few millimetres beyond the tip of the antenna. During antenna placement, orienting the antenna to point towards a non-target structure in close proximity to the tumour can help minimise the risk of unintended thermal damage to that structure.
Antenna design
Early generation MWA systems were limited by inefficient antennas that created elongated ablation zones with a ‘comet tail’ shape [Citation16]. More recent antenna designs are now available to create more focal, shorter ablation zones that are centred more towards the tip of the antenna [Citation14,Citation76–78]. Such antennas are particularly helpful when treating small or peripheral tumours. In addition, tissue-specific antennas tuned to the dielectric properties of different soft tissues have been developed to improve the efficiency of power delivery [Citation11,Citation79,Citation80]. With the current array of antennas and systems available, the historical deficiencies of low power delivery and elongated ablations have been nearly eliminated. A more in-depth discussion on the historical development of modern microwave antenna design is also discussed in this issue.
Conclusion
MWA systems generate heat at a faster rate and create larger ablation zones compared to radiofrequency ablation systems. Over the last 15 years, gradual technological improvements and an improved understanding of MW energy delivery have led to better control of the ablation zone and improved outcomes in treating early stage HCC and CRC metastases. The recent introduction of high-powered MWA systems has begun to draw interest as a viable treatment option against tumours larger than 3 cm in diameter. Patient safety and outcome data from multi-institutional ablation studies have also begun to be published, and novel strategies to improve patient safety as well as reduce local tumour progression rates are appearing in the clinical literature.
Disclosure statement
P.F.L., C.L.B. and F.T.L. are consultants of NeuWave Medical Inc. No financial support was provided for this review by any corporate interest. The authors alone are responsible for the content and writing of the paper.
References
- El-Serag HB. Hepatocellular carcinoma. N Engl J Med 2011;365:1118–27.
- Pons F, Varela M, Llovet JM. Staging systems in hepatocellular carcinoma. HPB (Oxford) 2005;7:35–41.
- Gillams A, Goldberg N, Ahmed M, Bale R, Breen D, Callstrom M, et al. Thermal ablation of colorectal liver metastases: a position paper by an international panel of ablation experts, the Interventional Oncology Sans Frontières meeting 2013. Eur Radiol 2015;25:3438–54.
- Bruix J, Sherman M, American Association for the Study of Liver Diseases. Management of hepatocellular carcinoma: an update. Hepatology 2011;53:1020–2.
- Ahmed M, Solbiati L, Brace CL, Breen DJ, Callstrom MR, Charboneau JW, et al. Image-guided tumor ablation: standardization of terminology and reporting criteria – a 10-year update. Radiology 2014;273:241–60.
- Chiang J, Hynes K, Brace CL. Flow-dependent vascular heat transfer during microwave thermal ablation. Conf Proc IEEE Eng Med Biol Soc 2012;2012:5582–5.
- Huang H-W. Influence of blood vessel on the thermal lesion formation during radiofrequency ablation for liver tumors. Med Phys 2013;40:073303.
- Haemmerich D. Hepatic radiofrequency ablation – an overview from an engineering perspective. Conf Proc IEEE Eng Med Biol Soc 2004;7:5433–6.
- Brace CL. Radiofrequency and microwave ablation of the liver, lung, kidney, and bone: what are the differences? Curr Probl Diagn Radiol 2009;38:135–43.
- Mulier S, Ruers T, Jamart J, Michel L, Marchal G, Ni Y. Radiofrequency ablation versus resection for resectable colorectal liver metastases: time for a randomized trial? An update. Dig Surg 2008;25:445–60.
- Brace CL. Microwave tissue ablation: biophysics, technology, and applications. Crit Rev Biomed Eng 2010;38:65–78.
- Dodd GD III, Dodd NA, Lanctot AC, Glueck DA. Effect of variation of portal venous blood flow on radiofrequency and microwave ablations in a blood-perfused bovine liver model. Radiology 2013;267:129–36.
- Ahmed M, Brace CL, Lee FT, Goldberg SN. Principles of and advances in percutaneous ablation. Radiology 2011;258:351–69.
- Lubner MG, Ziemlewicz TJ, Hinshaw JL, Lee FT, Sampson LA, Brace CL. Creation of short microwave ablation zones: in vivo characterization of single and paired modified triaxial antennas. J Vasc Interv Radiol 2014;25:1633–40.
- Lubner MG, Brace CL, Hinshaw JL, Lee FT Jr. Microwave tumor ablation: mechanism of action, clinical results, and devices. J Vasc Interv Radiol 2010;21(Suppl8):S192–S203.
- Bertram JM, Yang D, Converse MC, Webster JG, Mahvi DM. A review of coaxial-based interstitial antennas for hepatic microwave ablation. Crit Rev Biomed Eng 2006;34:187–213.
- Andreano A, Huang Y, Meloni MF, Lee FT, Brace C. Microwaves create larger ablations than radiofrequency when controlled for power in ex vivo tissue. Med Phys 2010;37:2967–73.
- Brace CL, Laeseke PF, Sampson LA, Frey TM, van der Weide DW, Lee FT. Microwave ablation with multiple simultaneously powered small-gauge triaxial antennas: results from an in vivo swine liver model. Radiology 2007;244:151–6.
- Wilson SR, Burns PN. Microbubble-enhanced US in body imaging: what role? Radiology 2010;257:24–39.
- Chiang J, Birla S, Bedoya M, Jones D, Subbiah J, Brace CL. Modeling and validation of microwave ablations with internal vaporization. IEEE Trans Biomed Eng 2015;62:657–63.
- Ai H, Wu S, Gao H, Zhao L, Yang C, Zeng Y. Temperature distribution analysis of tissue water vaporization during microwave ablation: experiments and simulations. Int J Hyperthermia 2012;28:674–85.
- Lopresto V, Pinto R, Cavagnaro M. Experimental characterisation of the thermal lesion induced by microwave ablation. Int J Hyperthermia 2014;30:110–18.
- Winokur RS, Du JY, Pua BB, Talenfeld AD, Sista AK, Schiffman MA, et al. Characterization of in vivo ablation zones following percutaneous microwave ablation of the liver with two commercially available devices: are manufacturer published reference values useful? J Vasc Interv Radiol 2014;25:1939–46.e1.
- Liu D, Brace CL. CT imaging during microwave ablation: analysis of spatial and temporal tissue contraction. Med Phys 2014;41:113303.
- Huo YR, Eslick GD. Microwave ablation compared to radiofrequency ablation for hepatic lesions: a meta-analysis. J Vasc Interv Radiol 2015;26:1139–46.e2.
- Liang P, Yu J, Yu X, Wang X, Wei Q, Yu S, et al. Percutaneous cooled-tip microwave ablation under ultrasound guidance for primary liver cancer: a multicentre analysis of 1363 treatment-naive lesions in 1007 patients in China. Gut 2012;61:1100–1.
- Groeschl RT, Pilgrim CHC, Hanna EM, Simo KA, Swan RZ, Sindram D, et al. Microwave ablation for hepatic malignancies: a multiinstitutional analysis. Ann Surg 2014;259:1195–200.
- Swan RZ, Sindram D, Martinie JB, Iannitti DA. Operative microwave ablation for hepatocellular carcinoma: complications, recurrence, and long-term outcomes. J Gastrointest Surg 2013;17: 719–29.
- Shi J, Sun Q, Wang Y, Jing X, Ding J, Yuan Q, et al. Comparison of microwave ablation and surgical resection for treatment of hepatocellular carcinomas conforming to Milan criteria. J Gastroenterol Hepatol 2014;29:1500–7.
- Jung YK, Jung CH, Seo YS, Kim JH, Kim TH, Yoo YJ, et al. BCLC stage B is a better designation for single large hepatocellular carcinoma than BCLC stage A. J Gastroenterol Hepatol 2016;31:467–74.
- Ziemlewicz TJ, Hinshaw JL, Lubner MG, Brace CL, Alexander ML, Agarwal P, et al. Percutaneous microwave ablation of hepatocellular carcinoma with a gas-cooled system: initial clinical results with 107 tumors. J Vasc Interv Radiol 2015;26:62–8.
- Zhang NN, Lu W, Cheng XJ, Liu JY, Zhou YH, Li F. High-powered microwave ablation of larger hepatocellular carcinoma: evaluation of recurrence rate and factors related to recurrence. Clin Radiol 2015;70:1237–43.
- Sun A-X, Cheng Z-L, Wu P-P, Sheng Y-H, Qu X-J, Lu W, et al. Clinical outcome of medium-sized hepatocellular carcinoma treated with microwave ablation. World J Gastroenterol 2015;21:2997–3004.
- Galimberti S, Dietrich C, Lazzaroni S, S Nahum G, Abate A, Sironi S, et al. Microwave ablation of hepatic tumors with a third generation system: locoregional efficacy in a prospective cohort study with intermediate term follow-up. Z Gastroenterol 2016;54:1–7.
- Liu Y, Li S, Wan X, Li Y, Li B, Zhang Y, et al. Efficacy and safety of thermal ablation in patients with liver metastases. Eur J Gastroenterol Hepatol 2013;25:442–6.
- Liang P, Dong B, Yu X, Yang Y, Yu D, Su L, et al. Prognostic factors for percutaneous microwave coagulation therapy of hepatic metastases. Am J Roentgenol 2003;181:1319–25.
- Lorentzen T, Skjoldbye BO, Nolsoe CP. Microwave ablation of liver metastases guided by contrast-enhanced ultrasound: experience with 125 metastases in 39 patients. Ultraschall Med 2011;32:492–6.
- Yang H-J, Lee J-H, Lee DH, Yu SJ, Kim YJ, Yoon J-H, et al. Small single-nodule hepatocellular carcinoma: comparison of transarterial chemoembolization, radiofrequency ablation, and hepatic resection by using inverse probability weighting. Radiology 2014;271:909–18.
- Hsu K-F, Chu C-H, Chan D-C, Yu J-C, Shih M-L, Hsieh H-F, et al. Superselective transarterial chemoembolization vs hepatic resection for resectable early-stage hepatocellular carcinoma in patients with Child-Pugh class A liver function. Eur J Radiol 2012;81:466–71.
- Higgins MCSS, Soulen MC. Combining locoregional therapies in the treatment of hepatocellular carcinoma. Semin Interv Radiol 2013;30:74–81.
- Xu L-F, Sun H-L, Chen Y-T, Ni J-Y, Chen D, Luo J-H, et al. Large primary hepatocellular carcinoma: transarterial chemoembolization monotherapy versus combined transarterial chemoembolization-percutaneous microwave coagulation therapy. J Gastroenterol Hepatol 2013;28:456–63.
- Liu C, Liang P, Liu F, Wang Y, Li X, Han Z, et al. MWA combined with TACE as a combined therapy for unresectable large-sized hepotocellular carcinoma. Int J Hyperthermia 2011;27:654–62.
- Ginsburg M, Zivin SP, Wroblewski K, Doshi T, Vasnani RJ, Van Ha TG. Comparison of combination therapies in the management of hepatocellular carcinoma: transarterial chemoembolization with radiofrequency ablation versus microwave ablation. J Vasc Interv Radiol 2015;26:330–41.
- Correa-Gallego C, Fong Y, Gonen M, D’Angelica MI, Allen PJ, DeMatteo RP, et al. A retrospective comparison of microwave ablation vs. radiofrequency ablation for colorectal cancer hepatic metastases. Ann Surg Oncol 2014;21:4278–83.
- Kong W-T, Zhang W-W, Qiu Y-D, Zhou T, Qiu J-L, Zhang W, et al. Major complications after radiofrequency ablation for liver tumors: analysis of 255 patients. World J Gastroenterol 2009;15:2651–6.
- Liang P, Wang Y, Yu X, Dong B. Malignant liver tumors: treatment with percutaneous microwave ablation – complications among cohort of 1136 patients. Radiology 2009;251:933–40.
- Livraghi T, Meloni F, Solbiati L, Zanus G, Collaborative Italian group using AMICA system. Complications of microwave ablation for liver tumors: results of a multicenter study. Cardiovasc Intervent Radiol 2012;35:868–74.
- Andreano A, Galimberti S, Franza E, Knavel EM, Sironi S, Lee FT, et al. Percutaneous microwave ablation of hepatic tumors: prospective evaluation of postablation syndrome and postprocedural pain. J Vasc Interv Radiol 2014;25:97–105.e1–2.
- Meloni MF, Andreano A, Bovo G, Chiarpotto B, Amabile C, Gelsomino S, et al. Acute portal venous injury after microwave ablation in an in vivo porcine model: a rare possible complication. J Vasc Interv Radiol 2011;22:947–51.
- Meloni MF, Andreano A, Lava M, Lazzaroni S, Okolicsanyi S, Sironi S. Segmental portal vein thrombosis after microwave ablation of liver tumors: Report of two cases. Eur J Radiol Extra 2010;76:e95–8.
- Yu NC, Raman SS, Kim YJ, Lassman C, Chang X, Lu DSK. Microwave liver ablation: influence of hepatic vein size on heat-sink effect in a porcine model. J Vasc Interv Radiol 2008;19:1087–92.
- Chiang J, Willey BJ, Del Rio AM, Hinshaw JL, Lee FT, Brace CL. Predictors of thrombosis in hepatic vasculature during microwave tumor ablation of an in vivo porcine model. J Vasc Interv Radiol 2014;25:1965–71.
- Meloni MF, Smolock A, Cantisani V, Bezzi M, D’Ambrosio F, Proiti M, et al. Contrast enhanced ultrasound in the evaluation and percutaneous treatment of hepatic and renal tumors. Eur J Radiol 2015;84:1666–74.
- Meloni MF, Andreano A, Franza E, Passamonti M, Lazzaroni S. Contrast enhanced ultrasound: should it play a role in immediate evaluation of liver tumors following thermal ablation? Eur J Radiol 2012;81:e897–902.
- Mauri G, De Beni S, Forzoni L, D’Onofrio S, Kolev V, Laganà MM, et al. Virtual navigator automatic registration technology in abdominal application. Conf Proc IEEE Eng Med Biol Soc 2014;2014:5570–4.
- Lee MW. Fusion imaging of real-time ultrasonography with CT or MRI for hepatic intervention. Ultrason Seoul Korea 2014;33:227–39.
- Lee MW, Kim YJ, Park HS, Yu NC, Jung SI, Ko SY, et al. Targeted sonography for small hepatocellular carcinoma discovered by CT or MRI: factors affecting sonographic detection. Am J Roentgenol 2010;194:W396–400.
- Minami Y, Chung H, Kudo M, Kitai S, Takahashi S, Inoue T, et al. Radiofrequency ablation of hepatocellular carcinoma: value of virtual CT sonography with magnetic navigation. Am J Roentgenol 2008;190:W335–41.
- Vilgrain V, Esvan M, Ronot M, Caumont-Prim A, Aubé C, Chatellier G. A meta-analysis of diffusion-weighted and gadoxetic acid-enhanced MR imaging for the detection of liver metastases. Eur Radiol 2016. PMID: 26883327.
- Harari CM, Magagna M, Bedoya M, Lee FT, Lubner MG, Hinshaw JL, et al. Microwave ablation: comparison of simultaneous and sequential activation of multiple antennas in liver model systems. Radiology 2016;278:95–103.
- Zhang M, Liang P, Cheng Z-G, Yu X-L, Han Z-Y, Yu J. Efficacy and safety of artificial ascites in assisting percutaneous microwave ablation of hepatic tumours adjacent to the gastrointestinal tract. Int J Hyperthermia 2014;30:134–41.
- Kitchin D, Lubner M, Ziemlewicz T, Hinshaw JL, Alexander M, Brace CL, et al. Microwave ablation of malignant hepatic tumours: intraperitoneal fluid instillation prevents collateral damage and allows more aggressive case selection. Int J Hyperthermia 2014;30:299–305.
- Hasegawa T, Takaki H, Miyagi H, Nakatsuka A, Uraki J, Yamanaka T, et al. Hyaluronic acid gel injection to prevent thermal injury of adjacent gastrointestinal tract during percutaneous liver radiofrequency ablation. Cardiovasc Intervent Radiol 2013;36:1144–6.
- Moreland AJ, Lubner MG, Ziemlewicz TJ, Kitchin DR, Hinshaw JL, Johnson AD, et al. Evaluation of a thermoprotective gel for hydrodissection during percutaneous microwave ablation: in vivo results. Cardiovasc Intervent Radiol 2015;38:722–30.
- Johnson A, Brace C. Heat transfer within hydrodissection fluids: an analysis of thermal conduction and convection using liquid and gel materials. Int J Hyperthermia 2015;31:551–9.
- Johnson A, Sprangers A, Cassidy P, Heyrman S, Hinshaw JL, Lubner M, et al. Design and validation of a thermoreversible material for percutaneous tissue hydrodissection. J Biomed Mater Res B Appl Biomater 2013;101:1400–9.
- Ballem N, Berber E, Pitt T, Siperstein A. Laparoscopic radiofrequency ablation of unresectable hepatocellular carcinoma: long-term follow-up. HPB (Oxford) 2008;10:315–20.
- de la Serna S, Vilana R, Sánchez-Cabús S, Calatayud D, Ferrer J, Molina V, et al. Results of laparoscopic radiofrequency ablation for HCC. Could the location of the tumour influence a complete response to treatment? A single European centre experience. HPB (Oxford) 2015;17:387–93.
- Santambrogio R, Opocher E, Zuin M, Selmi C, Bertolini E, Costa M, et al. Surgical resection versus laparoscopic radiofrequency ablation in patients with hepatocellular carcinoma and Child–Pugh class A liver cirrhosis. Ann Surg Oncol 2009;16:3289–98.
- Chung MH, Wood TF, Tsioulias GJ, Rose DM, Bilchik AJ. Laparoscopic radiofrequency ablation of unresectable hepatic malignancies. Surg Endosc 2001;15:1020–6.
- Nakazawa T, Kokubu S, Shibuya A, Ono K, Watanabe M, Hidaka H, et al. Radiofrequency ablation of hepatocellular carcinoma: correlation between local tumor progression after ablation and ablative margin. Am J Roentgenol 2007;188:480–8.
- Andreano A, Huang Y, Meloni MF, Lee FT, Brace C. Microwaves create larger ablations than radiofrequency when controlled for power in ex vivo tissue. Med Phys 2010;37:2967.
- Sommer CM, Bryant M, Kortes N, Stampfl U, Bellemann N, Mokry T, et al. Microwave ablation in porcine livers applying 5-minute protocols: influence of deployed energy on extent and shape of coagulation. J Vasc Interv Radiol 2012;23:1692–9.
- Bedoya M, del Rio AM, Chiang J, Brace CL. Microwave ablation energy delivery: influence of power pulsing on ablation results in an ex vivo and in vivo liver model. Med Phys 2014;41:123301.
- Biffi Gentili G, Ignesti C. Dual applicator thermal ablation at 2.45 GHz: a numerical comparison and experiments on synchronous versus asynchronous and switched-mode feeding. Int J Hyperthermia 2015;31:528–37.
- Longo I, Gentili GB, Cerretelli M, Tosoratti N. A coaxial antenna with miniaturized choke for minimally invasive interstitial heating. IEEE Trans Biomed Eng 2003;50:82–8.
- Ierardi AM, Mangano A, Floridi C, Dionigi G, Biondi A, Duka E, et al. A new system of microwave ablation at 2450 MHz: preliminary experience. Updates Surg 2015;67:39–45.
- Lloyd DM, Lau KN, Welsh F, Lee K-F, Sherlock DJ, Choti MA, et al. International multicentre prospective study on microwave ablation of liver tumours: preliminary results. HPB (Oxford) 2011;13:579–85.
- Durick NA, Laeseke PF, Broderick LS, Lee FT, Sampson LA, Frey TM, et al. Microwave ablation with triaxial antennas tuned for lung: results in an in vivo porcine model. Radiology 2008;247:80–7.
- Chiang J, Hynes KA, Bedoya M, Brace CL. A dual-slot microwave antenna for more spherical ablation zones: ex vivo and in vivo validation. Radiology 2013;268:382–9.