Abstract
Purpose: To study the efficiency of a dual slot antenna with a floating metallic sleeve on the ablation of different ex vivo bovine tissues.
Materials and methods: COMSOL Multiphysics® version 4.4 (Stockholm, Sweden), which is based on finite element methods (FEM), was used to design and simulate monopole and dual slot with sleeve antennas. Power, specific absorption rate (SAR), temperature and necrosis distributions in the selected tissues were determined using these antennas. Monopole and dual slot with sleeve antennas were designed, simulated, constructed and applied in this study based on a semi-rigid coaxial cable. Ex vivo experiments were performed on liver, lung, muscle and heart of bovine obtained from a public animal slaughter house. The microwave energy was delivered using a 2.45 GHz solid-state microwave generator at 40 W for 3, 5 and 10 min. Aspect ratio, ablation length and ablation diameter were also determined on ablated tissues and compared with simulated results. Student’s t-test was used to compare the statistically significant difference between the performance of the two antennas.
Results: The dual slot antenna with sleeve produces localised microwave energy better than the monopole antenna in all ablated tissues using simulation and experimental validation methods. There were significant differences in ablation diameter and aspect ratio between the sleeve antenna and monopole antenna. Additionally, there were no significant differences between the simulation and experimental results.
Conclusions: This study demonstrated that the dual slot antenna with sleeve produced larger ablation zones and higher sphericity index in ex vivo bovine tissues with minimal backward heating when compared with the monopole antenna.
Introduction
Microwave ablation (MWA) is being reported as an emerging technology for the treatment of tumours of many organs [Citation1–5]. It is mostly applied in the treatment of unresectable tumours situated in the liver, lung, kidney, bone, etc. It is a minimally invasive procedure under an image guidance system, such as magnetic resonance imaging (MRI), computed tomography (CT) or ultrasound, for diagnosis, monitoring and treatment assessment [Citation6–8]. Hepatic MWA has been studied more completely in liver than other tissues, both in vivo and ex vivo [Citation1,Citation4,Citation6,Citation9]. This may be due to the large size of the organ, high effective conductivity, high relative permittivity and high water content of the tissue [Citation2,Citation5,Citation6]. Meanwhile, research is ongoing to determine the efficacy of MWA of other tissues such as bone, kidney, breast, lung, muscle, etc. Thermal therapy is a type of cancer treatment in which tissue is exposed to temperature above 50 °C to induce cell killing by coagulation and denaturing protein structure within the cells [Citation1,Citation2,Citation6,Citation10]. Many techniques are now in use to deliver heat into tissue, which include microwave, radiofrequency (RF), laser and high-intensity focussed ultrasound [Citation6,Citation7]. Each of these techniques is aimed at increasing heat to the tissue and raising the temperature to 50 °C and above in order to destroy the cells within the localised tumour. During MWA, the antenna is inserted into the target organ either percutaneously, laparoscopically or in open surgery under image guidance. Several antennas have been proposed with the aim to locally deposit electromagnetic energy into the tissue for effective tumour treatment. These include the monopole antenna, single slot antenna and tri-axial antenna which suffer backward heating along the antenna shaft [Citation2,Citation3,Citation11,Citation12]. Cap-choked antennas have been proposed to reduce this unwanted backward heating along the antenna shaft which works well at lower power levels [Citation13,Citation14]; but the backward heating problem ensues at higher power levels or during the extended ablation durations [Citation3]. Recently, a balun-free helical antenna was proposed to offer a promising solution to reduce heating back along the coaxial cable feedline but it only worked best at 1.9 GHz [Citation15]. Heating of the antenna shaft can result from: impedance mismatch between the antenna and the surrounding tissue, heat conduction from inside the antenna shaft, heat conduction from the hot ablation zone along the antenna shaft or backward heating from the radiating segment [Citation11,Citation14]. Antenna characteristics relevant to thermal ablation include both the radiation distribution pattern and the reflection coefficient. In general, the lowest return loss is desirable to maximise energy transfer from the antenna into the tissue [Citation2,Citation12,Citation13].
Mostly, 915 MHz and 2.45 GHz are the typical frequencies for MWA technologies. At these frequencies, water, protein and other polar molecules tend to oscillate out of phase with the applied fields, so some of the electromagnetic energy is absorbed and converted to heat [Citation4]. Cell death is a function of elevated temperature and ablation duration. Typically, treatment durations for clinical application of the MWA technique are in the order of 3–12 min [Citation10,Citation16]. Heat conduction also plays a vital role at higher microwave frequencies in achieving a comparably sized ablation zone [Citation17]. Temperatures above 60 °C are known to cause instantaneous cell death, while temperatures from 50 to 60 °C will induce coagulation. Biological tissues (e.g. liver) of high water content, high relative permittivity and high effective conductivity are good absorbers of electromagnetic energy, whereas low relative permittivity, low effective conductivity and low water content tissues (e.g. fat) are lossless materials. Unlike RF, microwave can propagate through all types of tissues and non-metallic materials, including water vapour and dehydrated, charred and desiccated tissues. These characteristics make MWA a better option for the treatment of tumours in different tissues especially breast, thyroid, brain and spleen [Citation1–6].
Theoretical modelling is generally used to solve partial differential equations (PDEs) which govern electromagnetic wave propagation and heat transfer in tissue to determine the resultant temperature change during an ablation procedure. Computer modelling plays a crucial role in designing suitable antennas for MWA because it serves as a quick, convenient and inexpensive tool for evaluation, to isolate and optimise promising devices for prototyping. It helps also to understand the interaction between various physical phenomena that occur during ablation. The propagation of microwave in tissue is governed by Maxwell’s equations while temperature profile distribution is obtained by solving Pennes’ bio-heat equations and both have been discussed extensively by many authors [Citation2,Citation5,Citation13,Citation18]. Finite element method (FEM), finite difference time domain (FDTD), moment of method (MoM) and finite integration technique (FIT) are the numerical techniques for solving Maxwell’s equations [Citation19]. These techniques form the unique ways of assessing the resulting spatial distributions of internal electric fields, currents and rate of energy deposition in biological material.
The temperature profile in tissue is determined by the interaction of microwave with tissue and heat transfer in tissue which eventually lead to tissue damage as a result of elevated temperature.
The survival fraction of cells in tissue exposed to elevated temperature is given by [Citation18]
(1)
where C(0) is the original concentration of undamaged cells prior to heating, C(t) is the concentration of undamaged cells after heating, Ω is a dimensionless damaged parameter, A (1/s) is a frequency factor, Ea (J/mol) is the activation energy required to transform tissue from normal to damaged state, R (J/mol·K) is the universal gas constant and T (K) is the absolute temperature of tissue. Percentage of dead tissue, P, can be determined by using [Citation2]
(2)
From EquationEquation (2)(2) , when Ω = 1, there is 63% probability of cell death and when Ω = 4.6, probability of cell death rises to 99%. The first case of Ω = 1 corresponds to when tissue coagulation first occurs and tissue perfusion ceases.
In this study, we compare the efficiency of monopole and dual slot with sleeve antennas for MWA using simulation and experimental procedures on different ex vivo bovine tissues.
Materials and methods
Modelling and simulation of antennas
A schematic diagram of the antennas used in the study is depicted in . The coaxial monopole antenna () was created with a 14 mm radiating segment as in Labonte et al. [Citation20] while the dual slot antenna with floating metallic sleeve () was designed and fabricated to match the dimensions of the optimal geometry proposed by Ibitoye et al. [Citation21]. Both antennas were based on a 50 Ω R405/U semi-rigid coaxial cable from Pasternack Enterprise Inc. (Los Angeles, CA).
Figure 1. Schematic diagram of (a) monopole antenna and (b) dual antenna with a floating metallic sleeve.
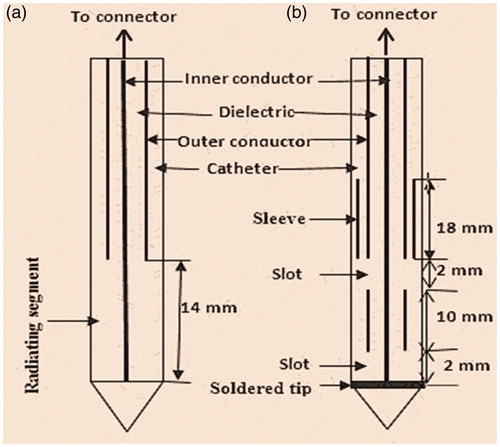
The coaxial cable has an inner conductor made of single strand silver-plated copper clad steel of 0.51 mm in diameter, dielectric made of polytetrafluoroethylene (PTFE) 1.68 mm in diameter and outer conductor made of copper 2.20 mm in diameter. The commercial software, COMSOL Multiphysics® version 4.4 (Stockholm, Sweden), which is based on FEM, was used in the design, simulation and computational studies of both Maxwell’s equations and Pennes’ bio-heat equations (RF and heat transfer modules) [Citation22]. The software enables us to specify antenna geometry as shown in . Biological tissues were also specified to include their pre-defined properties and those from the literature [Citation23–25]. The catheter, made of PTFE, acts as an interface between the antenna and the ablated tissue, and is mostly for hygienic and guidance purposes. The model takes advantage of the problem’s rotational symmetry, which allows modelling in 2D using cylindrical coordinates. When modelling in 2D, a fine mesh was selected to achieve excellent accuracy. The model uses a frequency-domain problem formulation with the complex-valued azimuthal component of the magnetic field as the unknown. The tissues were considered to have a cylindrical geometry and assumed to be homogeneous with 40 mm radius and 80 mm in height. The horizontal z-axis was oriented along the longitudinal axis of the antenna and the vertical r-axis oriented along the radial direction. An electromagnetic wave propagating in a coaxial cable is characterised by transverse electromagnetic (TEM) fields whereas in the liver tissue, an electromagnetic wave is characterised by transverse magnetic (TM) fields. In the electromagnetic wave propagation analysis, a scattering boundary condition is set on the surface, which means that the boundary does not disturb the electromagnetic field distribution. The microwave source is set at the upper end of the dielectric component of the coaxial cable using 40 W and 2.45 GHz for 10 min. This frequency is commonly used in MWA [Citation10] and it is the frequency of the microwave source available in our laboratory. The model assumes that the wall of the antenna is a perfect electric conductor (PEC), and the dielectric properties of tissues are also a function of temperature. While in the heat transfer analysis, temperature-dependent properties are considered for the thermal properties. No phase change occurs within the tissues, no energy exchange through the outer surface of liver tissue and no chemical reactions occur within the liver tissue. The asymmetric finite element model was discretised using an adaptive triangular element with a maximum element size of 3 mm and a Lagrange quadratic was used to approximate microwave power absorbed. The external surface of the tissues acted as boundary for the computational domain. Metallic components were assumed to be PECs and the coaxial dielectric was assumed to be lossless PTFE. Perfectly matched layers were assumed for all exterior boundaries. We used this simulation procedure to predict SAR distribution, temperature distribution and coagulative necrosis distribution in the tissue of monopole and dual slot with sleeve antennas. Also, we determined reflection coefficient, maximum SAR, the maximum temperature and total power deposition in the tissue. The lesion size and shape were determined using the 48 °C isothermal contour since this corresponds to 63% probability of cell damage. ImageJ version 1.47 (NIH, Bethesda, MA) was used to determine diameter longitudinal length and aspect ratio of the lesions generated by the antennas, both for simulated and prototype antennas.
Experimental procedures
Ex vivo measurements were performed on bovine liver, muscle, lung and heart with fresh tissues obtained from a local animal slaughter house. The antennas were constructed to match the prototype described in . The antenna was connected via connectors (Pasternack Enterprise Inc., Los Angeles, CA) to a semi-rigid coaxial cable attached to the 2.45 GHz solid-state microwave generator (Neyron-Cedex, France). Reflected and forward powers were monitored to ensure high power deposition into the tissues. The input power was set at 40 W for 3, 5 and 10 min for different tissues as used in the simulation procedures. The sleeve in the dual slot is made of 0.15 mm thick copper (Basic Copper, Carbondale, IL). Ablation was carried out in large bovine tissues with initial temperatures recorded by a highly sensitive 4-Channel Datalogging thermometer (SPER Scientific, Scottsdale, AZ). The use of 40 W as input power was based on the previous studies reported in the literature [Citation16,Citation21].
At the end of each ablation procedure, the sample was sliced longitudinally along the pathway created by the antenna to evaluate its maximum ablation length and diameter. Ablation diameter and length were measured using a ruler after leaving the ablated tissues for 3 h. Sliced tissues were also captured using a camera with high resolution for further analysis with ImageJ software which has the capability of improving contrast. Each measurement was repeated 4 times for determination of the mean. Aspect ratio was determined from the quotient of ablation diameter by ablation length. Student’s t-test in Statistical Package for Social Science (SPSS) version 20 (IBM, Armonk, NY) was used to analyse and compare the performance of the two antennas. A value of p ≤ .05 was considered statistically significant.
Results
Simulation results
The simulation results using monopole and dual slot with sleeve antennas on ex vivo liver, lung, muscle and heart of bovine are presented in . The highest power deposition was produced in the heart tissue (39.3 W) by the dual antenna with sleeve. Liver exhibits the lowest reflection coefficient while SAR was highest in the heart tissue of the dual slot antenna with sleeve. Ablation length was lowest (46.6 mm) in muscle with the dual antenna with sleeve compared to the monopole antenna. The ablation diameter was highest (43.5 mm) in liver tissue with the dual antenna with sleeve. Muscle produced the highest aspect ratio (0.93). The monopole antenna produced the lowest reflection coefficient (−6.81 dB) in the heart while the dual slot with sleeve antenna produced the lowest reflection coefficient (−24.2 dB) in liver.
Table 1. Simulated ablation results in liver, lung, muscle and heart.
Coagulation necrosis pattern distributions for simulated tissues are shown in . The value 1 in the colour bar legend of the figures corresponds to 63% probability of cell death EquationEquation (2)(2) and it also indicates when tissue coagulation first occurs and perfusion ceases [Citation2]. On the figures, the black lines indicate the 48 °C isothermal contour which corresponds to a region where 63% of coagulative necrosis occurs. These lines are used to determine the aspect ratio, diameter and longitudinal length of the lesions generated. Necrosis distributions of the simulated tissues are similar which may be as a result of close values of their relative permittivity, effective conductivity, density, thermal conductivity and water content as reported in the literature [Citation23–25].
Experimental results
Ninety-six ablations were carried out on different bovine tissues using 40 W for 3, 5 and 10 min with monopole and dual slot with sleeve antennas. On the pathologic inspection of the tissues, three zones of different degrees of ablation were noted. The inner zone appears pale, dark and brittle which indicate high degree of cell death and char; the middle zone appeared pink with coagulated indications and the outer appeared brighter at the boundary between the coagulated part and unaffected part. The ablation zone sizes and colours varied with type of tissue, the applied power, ablation duration and distance from the antenna surface. shows the ablated tissues of liver, lung, muscle and heart of ex vivo bovine using 40 W for 10 min with the dual antenna with sleeve and monopole antenna. present a graphical quantification of the ablation diameter, ablation length and aspect ratio as measured in the ablated ex vivo bovine tissue samples.
Discussion
This study presents the theoretical and experimental ex vivo ablation results of different bovine tissues using a dual slot antenna with a floating metallic sleeve and monopole antenna for MWA. The dual slot antenna with floating metallic sleeve demonstrated higher power deposition, low reflection coefficient, high peak SAR, larger ablation diameter, lower ablation length and higher aspect ratio compared to the monopole antenna (). The sleeve in the dual slot antenna helps to constrain the SAR distribution to the tip end of the antenna thereby reducing backward heating [Citation13,Citation14]. Impedance mismatch between the monopole antenna and the surrounding tissues might be responsible for the high reflection coefficient values in . and depict necrotic regions of tissues using simulation and experimental methods. Both results are indications that the dual slot antenna with sleeve localised microwave energy at the distal end better than the monopole antenna. The study also shows no significant difference in the ablation diameter, ablation length and aspect ratio between simulation and experimental results (). Significant difference was observed in the aspect ratios and ablation diameter between the two antennas ( and ). shows a significant difference in ablation length of the lung (p = .01). This might be as a result of its low density, low effective conductivity and low relative permittivity [Citation23–25]. Effective conductivity, relative permittivity, density, heat capacity, thermal capacity and heat transfer rate affect microwave energy distribution in ablated tissues as well as operating frequency. Tissue water content also plays a significant role in power dissipation during MWA [Citation1,Citation5,Citation17]. This study demonstrated that the dual antenna with sleeve is capable of constraining power locally into different tissues better than the monopole antenna. Low reflection coefficients and high power deposition exhibited by the dual antenna with sleeve will make it a better antenna for MWA of tumours that exhibit spherical morphology and are surgically unresectable. The monopole antenna exhibits backward heating along the antenna shaft and high return loss with low sphericity index [Citation2,Citation3,Citation11,Citation12]. The experimental results obtained in this study indicate that the extent of the microwave coagulation zone in liver, lung, muscle and heart tissues is similar to those reported by Zhou et al. [Citation26]. This study also reports the potential of using MWA in different tissues since microwave can propagate through all types of biological materials such as dehydrated, charred and desiccated tissues. According to this study, the sleeved antenna performed better than the monopole antenna in terms of large ablation diameter, low backward heating and high aspect ratio [Citation27]. Chiang et al. [Citation28] compared dual slot and monopole antennas; their results showed that the dual slot antenna produced more spherical ablation than the monopole antenna. Inclusion of a floating sleeve on the dual slot antenna has been reported to constrain microwave energy at the distal end of the antenna and produced ablation of high sphericity index than the dual slot without a sleeve [Citation21]. The findings of this study indicate that the dual slot antenna with sleeve will be suitable for most clinical applications.
Table 2. Comparison between simulation and experimental results using 40 W for 10 min. Values of p ≤ .05 were considered to be significantly different.
Conclusion
This study compared the efficiency of a dual slot antenna with a floating metallic sleeve and monopole antenna through simulation and experimental validation methods in different bovine tissues. There are significant differences in ablation diameter and ablation length between the monopole antenna and dual antenna with sleeve. Simulation results provide better understanding of SAR, temperature profile and coagulative necrosis distribution patterns by both antennas. Experimental results in several different tissues indicate that sleeved antennas localise microwave energy better than monopole antennas. Finally, dual slot antennas with sleeves have demonstrated the ability to produce well-defined lesions with more spherical morphology in a variety of different tissue types, and thus have the potential to make an impact in the treatment of tumours located in regions where adjacent organs need to be protected.
Disclosure statement
The authors declare no conflict of interest.
References
- Brace CL. (2009). Radiofrequency and microwave ablation of the liver, lung, kidney, and bone: what are the differences? Curr Probl Diagn Radiol 38:135–43.
- Prakash P. (2010). Theoretical modeling for hepatic microwave ablation. Open Biomed Eng J 4:135–38.
- Bertram JM, Yang D, Converse MC, et al. (2006). A review of coaxial-based interstitial antennas for hepatic microwave ablation. Crit Rev Biomed Eng 34:187–213.
- Simon CJ, Dupuy DE, Mayo-Smith WW. (2005). Microwave ablation: principles and applications. Radiographics 25:S69–83.
- Vorst VA, Rosen A, Kotsuka Y. (2006). RF/microwave interaction with biological tissues. Hoboken (NJ): IEEE.
- Habash RWY, Bansal R, Krewski D, et al. (2007). Thermal therapy, Part III: ablation techniques. Crit Rev Biomed Eng 35:37–121.
- Andreano A, Huang Y, Meloni MF, et al. (2010). Microwaves create larger ablations than radiofrequency when controlled for power in ex vivo tissue. Med Phys 37:2967–73.
- Li M, Yu X, Liang P, et al. (2015). Ultrasound-guided percutaneous microwave ablation for hepatic malignancy adjacent to the gallbladder. Int J Hyperthermia 31:579–87.
- Liu F-Y, Yu X-L, Liang P, et al. (2010). Comparison of percutaneous 915 MHz microwave ablation and 2450 MHz microwave ablation in large hepatocellular carcinoma. Int J Hyperthermia 26:448–55.
- Hall SK, Ooi EH, Payne SJ. (2015). Cell death, perfusion and electrical parameters are critical in models of hepatic radiofrequency ablation. Int J Hyperthermia 31:538–50.
- Brace CL. (2010). Microwave tissue ablation: biophysics, technology and applications. Crit Rev Biomed Eng 38:65–78.
- Lubner MG, Brace CL, Henshaw JL, et al. (2010). Microwave tumor ablation: mechanism of action, clinical results, and devices. J Vasc Interv Radiol 21:S192–203.
- Bertram JM, Yang D, Converse MC, et al. (2006). Antenna design for microwave hepatic ablation using an axisymmetric electromagnetic model. Biomed Eng Online 5:15. doi: 10.1186/1475-925X-5-15.
- Yang D, Bertram JM, Converse MC, et al. (2006). A floating sleeve antenna yields localized hepatic microwave ablation. IEEE Trans Biomed Eng 53:533–7.
- Luyen H, Hagness SC, Behdad N. (2015). A balun-free helical antenna for minimally invasive microwave ablation. IEEE Trans Antennas Propag 63:533–65.
- Hope WW, Schmelzer TM, Newcomb WL, et al. (2008). Guidelines for power and time variables for microwave ablation in a porcine liver. J Gastrointest Surg 12:463–7.
- Luyen H, Fuqiang G, Hagness SC, et al. (2014). Microwave ablation at 10.0 GHz achieves comparable ablation zones to 1.9 GHz in ex vivo bovine liver. IEEE Trans Biomed Eng 61:1702–10.
- Chang AI, Nguyen DU. (2004). Thermal modeling of lesion growth with radiofrequency ablation devices. Biomed Eng Online 3:27.
- Hand JW. (2008). Modelling the interaction of electromagnetic fields (10 MHz–10 GHz) with the human body: methods and applications. Phys Med Biol 53:27–86.
- Labonte S, Blais A, Legault S, et al. (1996). Monopole antennas for microwave catheter ablation. IEEE Trans Microwave Theory Tech 44:1832–40.
- Ibitoye AZ, Nwoye EO, Aweda MA, et al. Optimization of dual slot antenna using floating metallic sleeve for microwave ablation. (2015). Med Eng Phys 37:384–91.
- COMSOL Multiphysics users’ guide. Electromagnetic module and heat transfer module, Version 4.4; 2013 Nov.
- Andreuccetti D, Fossi R, Petrucci C. (1997). An internet resource for the calculation of the dielectric properties of body tissues in the frequency range 10 Hz–100 GHz IFAC-CNR, Florence, Italy. Available from: http://niremf.ifac.cnr.it/tissprop [last accessed 15 Aug 2014].
- Gabriel S. (1996). The dielectric properties of biological tissues: II. Measurements in the frequency range 10 Hz to 20 GHz. Phys Med Biol 41:2251–69.
- Hasgall PA, Di Gennaro F, Baumgartner C, et al. (2015). IT’IS Database for thermal and electromagnetic parameters of biological tissues, Version 2.6. Available from: www.itis.ethz.database [last accessed 13 Jan 2015].
- Zhou W, Liang M, Pan H, et al. (2013). Comparison of ablation zones among different tissues using 2450-MHz cooled-shaft microwave antenna: results in ex vivo porcine models. PLoS One 8:e71873.
- Ahmed M, Brace LC, Lee TF. Jr, et al. (2011). A dual-slot microwave antenna for more spherical ablation zones: ex vivo and in vivo validation. Radiology 258:351–69.
- Chiang J, Hynes KA, Bedoval M, et al. (2013). A dual-microwave antenna for more spherical ablation zones: ex vivo and in vivo validation. Radiology 268:382–9.