Abstract
This article summarises nearly all magnetic silica nanocomposites that have been synthesised for biomedical applications and evaluated under alternating magnetic field (AMF) from the point of view of heat generation. The use of these nanocomposites as a drug delivery system for remote control of drug release via applying AMF is described. Different parameters that affect the magnetic properties and, therefore, affect the amount of generated heat and the fact that sometimes these parameters are in conflict with each other are discussed. This review article presents insight into the synthesis of nanocomposites with optimal characteristics and use of them in optimal conditions to achieve the optimal magnetic properties for magnetic hyperthermia.
Introduction
Cancer is one of the main causes of deaths worldwide in 2012 [Citation1]. Several methods are used to treat different cancers. These methods can be classified into conventional and modern methods. Surgery, radiation therapy and chemotherapy are the main conventional methods but suffer from many limitations [Citation2]. Insufficiently, drug delivery to cancer cells besides adverse effects on the other tissues are examples of these limitations that cause the high-cancer mortality [Citation1]. Despite the recent technical advancements in these methods, the need for an efficient method for cancer therapy still remains [Citation2].
To date, much effort has been made to develop efficient methods that can enhance the therapeutic efficacy in cancer therapy [Citation3–7]. Hyperthermia has attracted much attention in the field of biomedical engineering. Hyperthermia is considered a "green therapy" for cancer treatment. Different studies have shown that high temperatures can damage tumour cells, usually with minimal damage to normal tissues. Treatment of tumour regions at temperatures between 41 and 47 °C allows tumour cell destruction while sparing the healthy ones. By destroying tumour cells and damaging proteins and structures within cells, hyperthermia can shrink tumours [Citation8,Citation9]. Hyperthermia is the preferred treatment for patients diagnosed with unresectable or complicated tumours or for patients who are looking for an alternative to costly and risky surgical operations [Citation10].
Heat also increases the efficacy of different chemotherapeutic drugs [Citation11]. Therefore, the combination of conventional chemotherapy with hyperthermia provides a promising strategy, which can have synergistic therapeutic effects on tumour cells and reduce the required effective doses of anticancer drugs [Citation12–14]. However, the key issue is that both conventional chemotherapy and hyperthermia should have the ability to deliver anticancer drugs and heat to the tumours simultaneously. Studies demonstrated the possibility for combination of chemotherapy and hyperthermia by the nanoparticle-based delivery systems [Citation15]. In particular, magnetic nanoparticles (MNPs) offer great potential as they can be loaded with anticancer drugs and heated under an external alternating magnetic field (AMF) after localisation in tumours [Citation12–14].
To achieve the treatment efficacy in hyperthermia for destroying cancer cells, maintaining sufficient temperature elevations for a period of time is necessary [Citation16–20]. Typically, if the heating time is longer than 1 or 2 h, the minimum temperature threshold to induce cytotoxic responses is 43 °C (6 °C above 37 °C). The required heating time for higher temperature elevations can be dramatically decreased due to the inverse logarithmic correlation between temperature elevation and heating time [Citation10]. One challenge in clinical application of hyperthermia is the lack of effective methods to localise heat only in diseased tissues. Numerous attempts have been reported to overcome this challenge. One example of efforts to localise the heat in the target region is magnetic hyperthermia. Magnetic hyperthermia has recently been highly regarded and is a promising method for its confirming heat within the tumour [Citation10]. Despite of the numerous benefits of combination therapy resulted from chemotherapy and hyperthermia, several studies showed that magnetic hyperthermia offers great potential as it can be used as single agent therapy for treatment of cancer [Citation10,Citation21–23].
One important challenge in magnetic hyperthermia is heat transfer to surrounding tissue that result in surrounding tissue damage. Accordingly, Salloum et al. have developed a computer algorithm that allows adjustments of injection parameters, such as injection rate and injection amount to elevate at least 90% of a tumour above certain threshold temperature (43 °C), while less than 10% of the surrounding tissue temperatures exceed this threshold [Citation24]. Magnetic hyperthermia was first investigated by Gilchrist et al. in 1957. In this kind of hyperthermia, magnetic materials are exposed to AMFs [Citation25]. The general procedure for magnetic hyperthermia involves the collection of MNPs via the local application of a magnetic field or surface functionalisation with targeting ligands. After localisation of MNPs in the desired location and then application of an AMFs, heat is generated and the temperature of the surrounding diseased tissue is increased [Citation26]. Heat generation in magnetic material may occur due to hysteresis loss, loss due to friction in viscous suspension, or Neel and Brownian relaxations [Citation27–29]. Preferably, the tissue is heated to approximately 42 °C for at least 30 min [Citation26]. The apparatus being used for generation of AMF for in vitro or in vivo studies is shown in . Some in vitro and in vivo studies revealed that magnetic hyperthermia has been successfully used in the treatment of some cancers, such as melanoma [Citation30], glioma [Citation31] and human colonic adenocarcinoma [Citation32].
Figure 1. Scheme of the apparatus for magnetic heating experiments for in vitro or in vivo applications: (1) Water-cooled exciting coil generating magnetic field; (2) Thermal insulating polystyrene foam; (3) Plastic tube for sample; (4) Fibre optic temperature probe; (5) Temperature register.
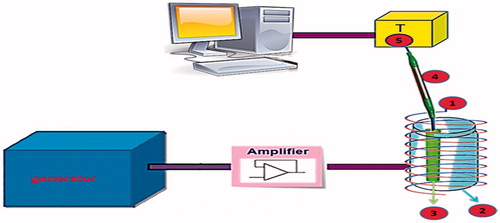
The intrinsic properties of MNPs, such as magnetisation, non-toxicity, biocompatibility and ability to functionalise the ligand shell [Citation33–37] make them a preferred non-invasive means for magnetic separation and detection of biological entities (e.g., cells, proteins, nucleic acids, enzymes, bacteria and viruses) [Citation35,Citation38], clinical diagnosis and therapy (including magnetic resonance imaging (MRI) and magnetic hyperthermia) [Citation38–42]. Ceramic-coated magnetic metal-oxide nanoparticles as MNPs have been of interest in the medical field for use in magnetic hyperthermia. Examples of different nanoparticles developed for magnetic hyperthermia are SiO2–Na2O–CaO–P2O5–FeO–Fe2O3 glass ceramic [Citation43], CaO–SiO2–P2O5–MgO–CaF2–MnO2–Fe2O3 glass ceramic [Citation44], FeSi microspheres [Citation45], La0.73Sr0.27MnO3–silica (LSMO) [Citation46], core–shell Co0.4Zn0.6Fe2O4+γ [Citation47], γ-Fe2O3@SiO2 [Citation48] and SiO2/Fe3O4 [Citation49]. The widest application of these nanoparticles is in cancer therapy as heat generators. In addition to the application of these nanoparticles in cancer therapy, they also can be used in other fields, such as microbiology. Firstly, microbes in liquid microbiological media were captured by silica-Fe3O4 nanoparticles. Then, the captured bacteria were aggregated using a magnetic bar. Finally, the generated heat through external application of an AC high-frequency magnetic field (HFMF) of 50–100 kHz (field strength =2.5 kAm−1, T = 80 °C, t = 10 min) completely destroyed these bacterial aggregations [Citation50]. Some of the various applications of magnetic silica nanocomposites are summarised in .
The most common parameter for measurement of the heating rate is specific absorption rate (SAR), also called specific loss power (SLP). A high SAR is a required factor for nanoparticles in magnetic hyperthermia. This factor is the amount of heat dissipated per unit mass of the magnetic material in an AMF:
(1)
where Cm is the specific heat capacity of the sample, msample and mmagnetic nanoparticle are the masses of the sample and magnetic nanoparticles, respectively, and ΔT is the temperature rise during the time interval (Δt) [Citation51].
The SAR value strongly depends on the nature and parameters of the magnetic nanosystem, such as particle size distribution, magnetic interaction of dipole, aggregation of particles, magnetisation of nanoparticle, and concentration of MNPs in suspension and medium of dispersion. Also, this parameter is influenced by AMF properties, such as strength and frequency of the magnetic field [Citation52–56]. The SAR value of nanosystem increases proportionally to the magnetic field strength and frequency as reported by Lartigue et al. for multicore iron oxide nanoparticles (IONPs) and Guardia et al. for iron oxide nanocubes [Citation57,Citation58]. According to the literature, medium frequencies are preferentially employed when larger objects are used for heat generation, whereas high frequencies are preferable for smaller objects [Citation59]. However, as these MNPs in naked form present high-specific surface area, unprotected nanoparticles can easily form aggregates when they are directly exposed to biological systems and react with oxygen in the air [Citation49].
The most popular magnetic silica nanocomposites have a core/shell structure. The shell is silica that coats a magnetic core. Different MNPs could be encapsulated into these magnetic nanocomposites. Some of these MNPs are summarised in . Several studies have used silica for embedding or encapsulating MNPs because of its unique properties, such as resistance to chemicals and high temperatures and ease of functionalization [Citation60]. Haematite (α-Fe2O3) is a ferromagnetic material with Ms = 0.4. The magnetic properties of haematite are much different from magnetite but some studies have analysed this nanoparticle for application in hyperthermia. Recently, Deka et al. performed the haemolytic test for various α-Fe2O3-SiO2 composites. All the investigated composites showed a haemolytic rate of less than 5%, which justifies their compatibility with blood, and all composites were found to be hemocompatible biomaterial [Citation61]. When MNPs are coated with silica, they achieve water stability at high concentrations as well as biocompatibility [Citation9]. This high stability in water is due to electrostatic repulsion of silanol groups present on the surface of silica [Citation62]. In addition, silica shells can minimise leakage of cargoes embedded in magnetic nanocomposites in the absence of the extrinsic stimulus [Citation63].
In this review, we focussed on magnetic silica nanocomposites synthesised for use in magnetic hyperthermia. Firstly, we discuss some general parameters that affect heating properties of magnetic nanoparticles. Then we focus on the already proven ability of magnetic nanosystems as both chemotherapy and hyperthermia agents. In the last part, this review was followed by a description of in vitro and in vivo studies of MNPs under an alternating magnetic field.
Effect of different parameters on magnetic properties of nanoparticles
The magnetic properties of MNPs are very strongly correlated to several parameters, such as their structure, method of synthesis, size and dispersion media. Zhang H-W et al. evaluated the effect of size on magnetic properties [Citation64]. MnFe2O4/SiO2 core/shell NPs with tuneable MnFe2O4 cores of 7, 12 and 18 nm have been synthesised. Magnetic heating measurements for aqueous solutions of MnFe2O4/SiO2 NPs (10-mg MnFe2O4/ml) under a RF field of 400 kHz showed that the 7-nm MnFe2O4/SiO2 NPs were unable to be heated even though the RF strength reached 320 Gs. On the other side, MnFe2O4/SiO2 NPs with the core sizes of 12 and 18 nm exhibited heat generation ability and MnFe2O4/SiO2 NPs with 18-nm magnetic core showed a much higher SAR value than those of the 12 nm cores. When the core sizes were larger than 18 nm, these NPs tended to aggregate in the solution due to the magnetic interaction [Citation64].
Silica in the structure of nanocomposites influences the magnetic properties of nanoparticles and much research has been done to the present to investigate the effect of silica on heat generation. In this regard, Villanueva et al. synthesised La0.56(SrCa)0.22MnO3 nanoparticles. They reported that magnetisation at 1 kOe was decreased by about 32% for the coated particles at room temperature. The total magnetisation value was decreased because of a large amount of diamagnetic silica. This reduction in magnetisation is expected to reduce the heating efficiency of the material as observed for IONPs. They also incubated HeLa tumour cells at 37 °C with La0.56(SrCa)0.22MnO3 under high-frequency AMF (f = 100 kHz, H = 15 mT, 30 min). Significant cell death by an apoptotic mechanism was observed, even though the bulk temperature increase was negligible and the temperature was always below 37.6 °C [Citation65]. Pollert and co-workers showed that extracellular heating of adult rat mesenchymal stem cells (rMSCs) through the application of 0.9 mg Mn ml−1 coated La1-xSrxMnO3 in combination with 45 min AMF resulted in the decrease of cellular viability by 80%. After heat treatment, the cell morphology was changed and the cells were swollen [Citation62]. In the next study, this group investigated the effect of silica on magnetic properties and they interestingly obtained different silica effect results. The heating experiments were carried out in an AC field of 100 kHz–1 MHz frequencies and 3.0–8.9 kAm−1 amplitudes on water dispersion of the samples, and it was demonstrated that the heating efficiency of the coated La1-xSrxMnO3 is generally higher than that of the bare magnetic core [Citation66], as shown in the previous study with γ-Fe2O3 (maghemite) nanoparticles [Citation67]. Considering the dominant role of hysteresis losses in the heating mechanism, this behaviour was related also to the decreased rotational motion of the silica-coated particles. It is also shown that the aggregation of the bare nanoparticles increases heating efficiency at least in a certain concentration range [Citation68]. This group also investigated magnetic heating of silica-coated Co-Zn ferrite particles. They pointed out that the correction of the SAR value may be important for very low concentrations of the magnetic particles in the suspension because of the imperfect thermal insulation calorimeter, and without knowledge of the behaviour of the calorimeter the data for SAR at some arbitrarily chosen temperature (e.g., 37 °C) may suffer from considerable error. On the other hand, the calorimetric equipment with imperfect insulation from the ambient is in a certain sense more closely resembling the in vivo situation, which possesses some additional features as, e.g., the flow of the medium (blood) carrying the particles or thermoregulation of the living system striving to maintain equilibrium [Citation47]. Tao et al. also evaluated the effect of 150 nm Fe3O4/SiO2 nanoparticles. Results showed that the SAR value is decreased for this nanoparticle compared to Fe3O4 nanoparticles when 50 mg ml−1 aqueous solution of Fe3O4 or magnetic mesoporous silica (MMS) nanoparticles were exposed to an AMF (f = 238 and 409 kHz and H = 150 Gauss). This phenomenon is due to presence of a lot of mesoporous silica matrices in MMS nanoparticles. It can be observed that the magnetic heating capacity of MMS nanoparticles at 409 kHz was much better than that at 238 kHz. Moreover, the SAR value of Fe3O4 and MMS nanoparticles increased with an increase of magnetic field strength [Citation69]. Similar results have been reported previously [Citation70–72]. For example, Gonzalez-Fernandez et al. showed that the silica coating significantly decreases the SAR value by hindering the heat flow, therefore, decreasing the heating efficiency. This indicates that the silica coating on the MNPs should be designed to be the minimum necessary to keep the nanocomposites stable in water. In addition, they studied the influence of the size and silica coating of IONPs on the heating rates. They used an AMF of 260 kHz frequency and 100-Oe amplitude to induce heat generation. The results showed strong effect of the size of Fe3O4 nanoparticles on the SAR, with maximum SAR achieved by the optimal size of 24 nm, and for nanoparticles below or above this optimal, the specific power absorption (SPA) decreased abruptly [Citation71]. This is in agreement with the expected behaviour for mechanisms based on Néel relaxation as first reported by Rosensweig [Citation73] and experimentally proved in many systems [Citation74,Citation75]. In this way, 25 mg ml−1 of IONPs and Si-IONPs in the agar phantom have been used by Kawashita et al. for heat generating experiments. The Si-IONPs samples showed higher SAR values, but lower ΔT/Δt ratio compared to the values of the IONPs. They attributed this observation to the low-magnetic IONPs content in the agar phantom. Also they reported that the heat generated by the microspheres can be improved by increasing the content of microspheres in the agar phantom [Citation76].
It has been proposed that the conditions of the initial synthesis of nanoparticle affect the subsequent heat generation. For example, the temperature of the initial synthesis of MNPs affects the magnetic properties of nanoparticles. In one study, it has been shown that the silica coated Fe3O4 nanoparticles prepared at 80 °C (Fe3O4/SiO2 80) generated the highest SAR value compared to the sample prepared at 100 °C (Fe3O4/SiO2 100) and uncoated Fe3O4. This reveals the importance of the initial temperature of synthesis on heat efficiency of nanoparticle. The increase in the SAR value of the coated sample in comparison with the naked sample is attributed to increase in Brownian motion after coating. On the other hand, the SAR value of Fe3O4 was higher compared with Fe3O4/SiO2 100 MNPs. This is another confirmation of the importance of the temperature of initial synthesis that cause the decrease of the SAR value for nanoparticle synthesised at 100 °C despite an increase of Brownian motion of these nanoparticles. Moreover, the SAR value decreases with increase in shell thickness. The cell culture experiments demonstrated that the viability of HeLa cells was reduced to 42% as these cells were exposed to a temperature of 43 °C (for 1000s), which was induced by applying an AMF (applied current = 400 A, f = 250 kHz, t = 20 min) to Fe3O4/SiO2 80 MNPs [Citation77].
Nanoparticle dispersion media has an important role in the resulting heat. This point has been investigated by some studies. In one research, firstly 4 mg ml−1 IONs and silica coated IONs were dispersed in water. It has been found that the ΔT of the IONs dispersed in an aqueous solution of tetramethylammonium hydroxide (TMAOH) when exposed to AMF (198 kHz, 20 min) is higher than free TMAOH IONs and silica coated IONs. Both IONs and IONs/TMAOH had similar grain size particles, but the sample IONs/TMAOH was better dispersed [Citation78]. According to literature, Brownian rotation of an aggregate was much slower than that of single particles under an oscillating magnetic field [Citation79]. The well-dispersed MNPs generated higher SAR values than non-dispersed samples [Citation80]. In the next step, 4 mg g−1 IONs and silica coated IONs were dispersed in the hydrogel and were exposed to AMF (198 kHz, 20 min). Interestingly, the ΔT of the coated IONs was higher than IONs and IONs/TMAOH. In the hydrogel-dispersed samples, rotation of particles was restricted and the magnetic moment relaxed only through Néel relaxation. Furthermore, Hurley et al. reported that complex and viscous materials (2X PBS, 2X PBS and 1% agarose mixture, culture media) result in aggregation and a subsequent decrease in SAR for IONPs in agreement with previous work (190 kHz and 20 kAm−1 acquisition parameters) [Citation81]. In contrast, Si-IONPs demonstrated a lower SAR in water but maintain that value in all tested media. The 2X PBS and 1% agarose mixture is intended to mimic evaluation in the in vivo biological environments like tissue or extracellular matrix [Citation54].
Controlled release nanocomposites
The use of specific functionalities to block nanoparticle pores is an efficient method for prevention of premature drug leakage of drug embedded inside the pores of the nanoparticle. In non-covalent binding strategies, potential drug pre-release or leakage can be avoided by using stimulus functionalities on the nanoparticle shell to block or cover the drug releasing pores [Citation82]. In this regard, nucleic acid-magnetic nanocomposites were developed as components of “on-off” drug delivery systems with controlled drug release. In 2011, Vallet-Regí et al. designed a smart thermoresponsive drug delivery system. Single-stranded DNA molecules were conjugated to the magnetic nanocrystals and then hybridised with complementary DNA sequence attached to the MSNCs to cap the nanopores of MSNCs. In this drug delivery system, γ-Fe2O3 nanoparticles were encapsulated in the mesoporous silica matrix. The magnetic component allows reaching to hyperthermic temperatures (42–47 °C) under an AC magnetic field of 24 kAm−1 and 100 kHz. This increase in temperature causes denaturation of the DNA-duplex. As a result, pores on the silica surface are opened and fluorescein (as a model drug) is released. On cooling to 20 °C, complementary oligonucleotides are hybridised with each other and pores are blocked by nanoparticles and fluorescein-labelled oligonucleotides are retained again within the mesoporous silica [Citation83]. In similar work, Yufang Zhu et al. conjugated carboxyl-modified DNA20 as a gatekeeper onto the aminated magnetic mesoporous silica nanoparticles (MMSNs) to form a reversible “on-off” system. Their system showed that the release of DOX was very slow when the temperature decreased to 37 °C, but the drug release rate speeded up once the temperature increased to 43 °C. It might be that the electrostatic interaction between MMSN-NH2 and another carboxyl-DNA20 would be weaker when the temperature increases. Accordingly, the MMSN-NH2 could efficiently enhance the release of the drug upon exposure to an AMF due to their superparamagnetic behaviour [Citation84]. Heat is generated as a result of dynamic hysteresis. Dynamic hysteresis is one of the characteristics of superparamagnetic materials. When a superparamagnetic nanoparticle exposure to the alternating magnetic field, dynamic hysteresis is appeared [Citation85]. In another study, this group prepared DNA-capped Fe3O4/SiO2 MNPs with the capability of loading chemotherapeutic agents and drug release at 50 °C due to the denaturation of the dsDNA capping chains after hyperthermia treatment [Citation86]. Such an approach also was used with thermo-sensitive polymer instead of DNA. A thermo-sensitive polymer P(EO-co-LLA) was used as “gatekeeper” outside of Fe3O4@mSiO2. After loading of the anticancer drug DOX, the composites showed controlled drug release behaviour triggered by the hyperthermia of Fe3O4 under AMF. Chemotherapy with the anticancer drug combined with magnetic hyperthermia makes the cytotoxicity of these nanocomposites higher than that of free DOX on HeLa cells [Citation87]. In similar fashion, Vallet-Regí et al. formed a temperature-responsive controlled release system, in which the Fe3O4-MSNCs’ surface was decorated with a thermosensitive copolymer of poly (ethyleneimine)-b-poly (N-isopropylacrylamide) (PEI/NIPAM). The polymer acted as a gatekeeper that traps the fluorescein as a model drug loaded in pores, as well as embedding protein into the polymer shell by hydrogen or electrostatic interactions. This design has the ability to create synergy effect associated with hyperthermia and chemotherapy as well as preventing uncontrolled release of the drug at low temperatures (∼20 °C); it enabled the controlled release of the drug when the temperature exceeded 35–40 °C under alternating magnetic fields [Citation88].
It is reported that hollow and porous silica nanospheres containing IONs and DOX have an ability to release the drug in a temperature-dependent manner. In the absence of AMF, the release of DOX can be done via diffusion through the pores of the silica shell. However, when AMF induces magnetic hyperthermia effects, the generated heat increases the drug release rate [Citation89]. In 2014, Mancuso et al. have reported on the development of new maghemite-magnetite@silica core–shell nanostructure conjugated via a thermolabile linker to the ansamitocin derivatives. This superparamagnetic nanostructure can be heated under an AMF and then the cytotoxic ansamitocin derivatives are released. Most importantly, released ansamitocin derivatives show strong antiproliferative activity against several mammalian cancer cell lines. Also, this nanostructure can act simultaneously in hyperthermia and in chemotherapy [Citation59]. In another study in this area, zinc-doped iron oxide nanocrystals (ZnNCs) containing mesoporous silica nanoparticles were developed that were surface-decorated with valves for controlling drug release. Upon application of an AC magnetic field, the ZnNCs generate local internal heat and, therefore, this non-invasive system is disassembled and easily releases the loaded drug. When breast cancer cells (MDA-MB-231) were treated with this doxorubicin-loaded nanosystem and exposed to an alternating magnetic field, cell death occurred as a result of both chemotherapy and magnetic hyperthermia [Citation90].
In vitro and in vivo studies
To extend the magnetic silica nanocomposites to clinical treatments, in vitro and in vivo evaluations are essential. introduces some of the MSNCs that have been used in cellular and in vivo experiments. Very recently, Ting-Yu Liub and co-workers reported the use of magnetic core/shell nanovehicles with polymeric core involved Fe3O4 nanoparticles and the silica shell presenting the antibody-targeting peptide AP-1. These magnetic nanovehicles could encapsulate drugs of various hydrophilicities through amphiphilic poly (vinyl alcohol) as a polymer binder. CT26, a colorectal cancer cell line with over-expressed IL-4Rα was used in their study. In vitro experiments revealed that under a HFMF of 50–100 kHz, magnetic nanovehicles incorporating doxorubicin were collapsed and approximately 80% of the drug payload was released due to heat generation. This system showed rapid and accurate drug release. Moreover, in vivo experiments demonstrated that concurrent with the release of the drug, the magnetic Fe3O4 nanoparticles themselves could also destroy the tumour cells through hyperthermia [Citation63].
Table 1. Magnetic silica nanocomposites used in cellular and in vivo tests.
By trapping the SPIONs embedded in silica microparticles into in situ forming implants (ISFI), SPIONs are protected and durably circumvented at the injection site, avoiding their phagocytosis and distant migration. The designed system can potentially decrease the deleterious effect of spreading particles and maintain them within the tumour site for successive heating. Such an approach was investigated by Le Renard et al. They synthesised 900 nm silica nanoparticles embedding SPIONs and carried out hyperthermia therapy on colorectal cancer-bearing Swiss nude mice. The microparticles were injected intratumorally and an AMF at 141 kHz with field strengths between 9 and 12 mT was applied for 20 min. As the field strength increased, the amount of viable tumour tissue decreased and areas of damaged tissue due to hyperthermia increased. After using these nanoparticles, mice receiving a single 20 min treatment at 12 mT had a median survival of 37 d compared to 12 d without treatment. Moreover, 45% of the animals had a 1-year survival after treatment [Citation91]. In the next study, a group developed organogel formulations (i.e., water-precipitating polymer dissolved in a water-miscible organic solvent) incorporating SPIONs embedded in silica microparticles for treating nude mice carrying a subcutaneous human colon carcinoma by magnetically induced local hyperthermia [Citation31]. In addition, two ISFI formulations based on alginate and on a poly(ethylene-co-vinyl alcohol) (EVAL)) have been developed that entrap silica microparticles embedding maghemite for investigation of their ability to block heat transfer. Heat delivery plays a critical role and is enervated by more or less efficient body cooling, which is largely mediated by blood flow. The temperature of the blood flowing into the tumour is lower than the tumour region and takes away the heat from the tumour during hyperthermia. A heat sink effect significantly occurs through large blood vessels continuously surrounding the tumour and thus limits the thermal ablative area. Many theoretical, experimental, and clinical studies on body cooling of large blood vessels have demonstrated such effects [Citation92,Citation93]. In this regard, both formulations showed the ability to act as embolising agents that block blood flow in vascular or tumour lesions, not only when injected through intravascular routes but also when injected in solid tumours. The embolising properties could contribute to increasing the heating efficiency in situ and thereby increase the therapeutic potential [Citation94].
Generally, for clinical application of the magnetic silica nanocomposites, estimation of their compatibility and toxicity is necessary. For this purpose, composites of maghemite nanoparticles embedded in mesoporous silica matrix forming magnetic microspheres have been examined. The results showed a biocompatible behaviour when these nanocomposites were assessed in in vitro assays with human A549, Saos-2 and HepG2 cells. Confocal microscopy studies revealed that the material did not interfere with cell extension or morphology. The microparticles were efficiently internalised by human tumour cells and localised outside the cell nucleus, and there were no signs of apoptosis, such as nuclear condensation or fragmentation; neither was metabolic activity significantly altered. They also carried out the magnetic hyperthermia experiments and proved that these microparticles have the ability to control the temperature rise in the cell culture environment upon exposure to an AMF, thus generating heat treatments that severely compromise cell survival [Citation95]. Prasad et al. also observed the same phenomenon of cell death in HeLa cancer cells using γ-MnxFe2–xO3 nanoparticles, as did Bae et al. in human lung carcinoma A549 cells using chitosan coated ferrimagnetic iron oxide cube nanoparticles and Kumar et al. in HeLa cells with nanoassemblies@Dye-SiO2@SiO2 [Citation53,Citation96,Citation97]. Shanta Singh et al. have developed mesoporous silica encapsulated with luminescent YVO4: Eu3C nanoparticles. These nanocomposites were loaded with the anticancer drug DOX and have been investigated for in vitro experiments. The pH-dependent release of DOX causes death in HeLa and MCF-7 cells. After adding SPIONs, a synergistic effect could be achieved by applying an alternating magnetic field, which gives a more effective tumour cell death of about 90% due to the simultaneous effect of the chemo and thermal therapies. Besides, the drug release is enhanced in the presence of an oscillating AC magnetic field [Citation98].
Toxicity and biocompatibility
The prelude of achieving to a resultful treatment of cancer through magnetic hyperthermia is confident of biocompatibility of magnetic nanoparticles. In this sense, numerous researchers have been studied the toxicity and biocompatibility of magnetic nanoparticles. Following paragraphs summarise some selected points in this issue.
Recently Arami and co-workers reviewed the in vivo toxicity of IONPs [Citation99]. IONPs are one of the most reliable candidates for use in a wide range of biomedical applications. Three major parameters guarantee clinical success of the IONPs: pharmacokinetics, short- and long-term tolerability in the body and therapeutic or diagnostic functionality in the desired organ. In reality, in vivo performance of the IONPs effected by various characteristics of these particles, such as core and hydrodynamic size, morphology, size polydispersity, surface charge and type of the coating molecules. Other experimental variations, such as method of administration, variations between animal models and humans and different characterisation techniques used can be also considered as influential factors [Citation99].
However, biomedical applications of IONPs doped with magnetically susceptible elements (e.g., MnFe2O4 and CoFe2O4) and metal alloys nanoparticles (e.g., FeCo and FePt) are much more restricted, because of their potential toxicity and rapid oxidation, even though the magnetism of these ferrites and metal alloys is stronger than pure IONPs [Citation100].
Biocompatible shell-coated IONPs are generally less toxic compared with naked ones; and the biocompatibility depends on the type of coating [Citation99]. Up to now, clinical trials have been done for only two families of IONPs, i.e., those coated with polysaccharides or silica [Citation99].
Silica is “generally recognized as safe” by the US FDA. Amorphous silica is an FDA-approved food additive, whereas crystalline silica is a suspected human carcinogen and is involved in the pathogenesis of silicosis [Citation101]. However, Up to now, toxicology studies on inorganic-shell coated IONPs have been very limited; MTT results showed that silica coated IONPs oxide nanoparticles are non-toxic because these inorganic coating are generally biologically inert and provide a stable protective layer against oxidation and reactive species [Citation100].
Conclusion
This article is a review on magnetic silica nanocomposites in applied AMF and explains parameters that affect the magnetic properties of nanocomposites, such as size, silica coat, synthesis conditions and dispersion media. When the MNPs heat under AMF exposure and create a proper SAR value for hyperthermia applications, the optimal magnetic properties are provided. The size of magnetic agent is of particular importance and should be an optimal value to provide good heat generation ability without any aggregation. This optimum amount depends on the type of nanoparticle. This optimal size is approximately 30 nm for Fe3O4 in aqueous solution. The silica coat thickness is also important. The optimal amount of silica should be the minimum necessary to keep the nanocomposites stable in water as well as does not reduce the heat generation ability. Another important point is the dispersion medium. When the dispersion medium of water goes to a more complex medium that simulates the conditions of the body, silica coated nanoparticles induce better heat efficiency than uncoated nanoparticles. Synthesis condition of nanoparticles is also of great importance. For example, the temperature of the initial synthesis of MNPs affects its heat generation properties. Therefore, the reaction conditions should be optimised.
Magnetic hyperthermia is one of several types of hyperthermia that have attracted significant attention in recent years with the development of magnetic particles. Using alternating magnetic field, the MNPs can be heated in the target tissue, so the efficacy of the combination of this treatment method and chemotherapy is greater compared to the only traditional chemotherapy. A wide range of MNPs has been developed so far. According to the literature, IONPs and perovskite are two types of nanoparticles that have been most widely used in the fabrication of magnetic nanocomposites. Magnetic properties and heat generation are dependent on field conditions, type of magnetic agents, size, aggregations, silica, thickness, and functionality of silica, dispersion media and condition of synthesis. It was observed that in some studies the generated heat was reduced due to the presence of silica but other studies showed contradictory results. Thus, any assumption concerning this influence seems to be rather speculative and future more detailed experiments to elucidate this problem should be carried out. Controlled drug release from various nanostructures has been considered. It is shown that silica nanocomposites are good candidates for combining both conventional chemotherapy and hyperthermia, therefore, they have a potential for further applications in clinical trials.
Disclosure statement
The authors report no declarations of interest.
References
- Sun T, Zhang YS, Pang B, et al. (2014). Engineered nanoparticles for drug delivery in cancer therapy. Angew Chem Int Ed Engl 53:12320–64.
- Hehr T, Wust P, Bamberg M, Budach W. (2003). Current and potential role of thermoradiotherapy for solid tumours. Onkologie 26:295–302.
- Falk M, Issels R. (2001). Hyperthermia in oncology. Int J Hyperthermia 17:1–18.
- Kobayashi T. (2011). Cancer hyperthermia using magnetic nanoparticles. Biotechnol J 6:1342–7.
- Drake P, Cho HJ, Shih PS, et al. (2007). Gd-doped iron-oxide nanoparticles for tumour therapy via magnetic field hyperthermia. J Mater Chem 17:4914–18.
- Zhang M, Garbuzenko OB, Reuhl KR, et al. (2012). Two-in-one: combined targeted chemo and gene therapy for tumor suppression and prevention of metastases. Nanomedicine (Lond) 7:185–97.
- Levi-Polyachenko NH, Stewart JH. (2011). Clinical relevance of nanoparticle induced hyperthermia for drug delivery and treatment of abdominal cancers. Open Nanomed J 3:24–37.
- Wang Q, Deng ZS, Liu J. (2012). Theoretical evaluations of magnetic nanoparticle-enhanced heating on tumour embedded with large blood vessels during hyperthermia. J Nanopart Res 14:974.
- Epherre R, Duguet E, Mornet S, et al. (2011). Manganite perovskite nanoparticles for self-controlled magnetic fluid hyperthermia: about the suitability of an aqueous combustion synthesis route. J Mater Chem 21:4393–401.
- Attaluri A, Ma R, Qiu Y, et al. (2011). Nanoparticle distribution and temperature elevations in prostatic tumours in mice during magnetic nanoparticle hyperthermia. Int J Hyperthermia 27:491–502.
- Lee H, Kim S, Choi BH, et al. (2011). Hyperthermia improves therapeutic efficacy of doxorubicin carried by mesoporous silica nanocontainers in human lung cancer cells. Int J Hyperthermia 27:698–707.
- Taratula O, Dani RK, Schumann C, et al. (2013). Multifunctional nanomedicine platform for concurrent delivery of chemotherapeutic drugs and mild hyperthermia to ovarian cancer cells. Int J Pharm 458:169–80.
- Ren Y, Zhang H, Chen B, et al. (2012). Multifunctional magnetic Fe3O4 nanoparticles combined with chemotherapy and hyperthermia to overcome multidrug resistance. Int J Nanomedicine 7:2261–9.
- Pradhan P, Giri J, Rieken F, et al. (2010). Targeted temperature sensitive magnetic liposomes for thermo-chemotherapy. J Control Release 142:108–21.
- Kumar CS, Mohammad F. (2011). Magnetic nanomaterials for hyperthermia-based therapy and controlled drug delivery. Adv Drug Deliv Rev 63:789–808.
- Engin K. (1994). Biological rationale for hyperthermia in cancer treatment (II). Neoplasma 41:277–83.
- Masuko Y, Tazawa K, Viroonchatapan E, et al. (1995). Possibility of thermosensitive magnetoliposomes as a new agent for electromagnetic induced hyperthermia. Biol Pharm Bull 18:1802–4.
- Johannsen M, Jordan A, Scholz R, et al. (2004). Evaluation of magnetic fluid hyperthermia in a standard rat model of prostate cancer. J Endourol 18:495–500.
- Jordan A, Scholz R, Wust P, et al. (1999). Magnetic fluid hyperthermia (MFH): cancer treatment with AC magnetic field induced excitation of biocompatible superparamagnetic nanoparticles. J Magn Magn Mater 201:413–19.
- Hergt R, Hiergeist R, Hilger I, et al. (2004). Maghemite nanoparticles with very high AC-losses for application in RF-magnetic hyperthermia. J Magn Magn Mater 270:345–57.
- Bubnovskaya L, Belous A, Solopan A, et al. (2012). Nanohyperthermia of malignant tumors. II. In vivo tumor heating with manganese perovskite nanoparticles. Exp Oncol 34:336–9.
- Johannsen M, Gneveckow U, Thiesen B, et al. (2007). Thermotherapy of prostate cancer using magnetic nanoparticles: feasibility, imaging, and three-dimensional temperature distribution. Eur Urol 52:1653–62.
- Silva AC, Oliveira TR, Mamani JB, et al. (2011). Application of hyperthermia induced by superparamagnetic iron oxide nanoparticles in glioma treatment. Int J Nanomedicine 6:591–603.
- Salloum M, Ma R, Zhu L. (2009). Enhancement in treatment planning for magnetic nanoparticle hyperthermia: optimization of the heat absorption pattern. Int J Hyperthermia 25:309–21.
- Gilchrist R, Medal R, Shorey WD, et al. (1957). Selective inductive heating of lymph nodes. Ann Surg 146:596
- Pankhurst QA, Connolly J, Jones S, Dobson J. (2003). Applications of magnetic nanoparticles in biomedicine. J Phys D Appl Phys 36:R167.
- Tampieri A, D’Alessandro T, Sandri M, et al. (2012). Intrinsic magnetism and hyperthermia in bioactive Fe-doped hydroxyapatite. Acta Biomater 8:843–51.
- Murakami S, Hosono T, Jeyadevan B, et al. (2008). Hydrothermal synthesis of magnetite/hydroxyapatite composite material for hyperthermia therapy for bone cancer. J Ceram Soc Jpn 116:950–4.
- Lévy M, Wilhelm C, Siaugue J-M, et al. (2008). Magnetically induced hyperthermia: size-dependent heating power of γ-Fe(2)O(3) nanoparticles. J Phys Condens Matter 20:204133
- Muthana M, Multhoff G, Graham Pockley A. (2010). Tumour infiltrating host cells and their significance for hyperthermia. Int J Hyperthermia 26:247–55.
- Le Renard PE, Jordan O, Faes A, et al. (2010). The in vivo performance of magnetic particle-loaded injectable, in situ gelling, carriers for the delivery of local hyperthermia. Biomaterials 31:691–705.
- Latorre-Esteves M, Cortés A, Torres-Lugo M, Rinaldi C. (2009). Synthesis and characterisation of carboxymethyl dextran-coated Mn/Zn ferrite for biomedical applications. J Magn Magn Mater 321:3061–6.
- Brullot W, Reddy NK, Wouters J, et al. (2012). Versatile ferrofluids based on polyethylene glycol coated iron oxide nanoparticles. J Magn Magn Mater 324:1919–25.
- Xu H, Aguilar ZP, Yang L, et al. (2011). Antibody conjugated magnetic iron oxide nanoparticles for cancer cell separation in fresh whole blood. Biomaterials 32:9758–65.
- Shahbazi-Gahrouei D, Abdolahi M, Zarkesh-Esfahani SH, et al. (2013). Functionalised magnetic nanoparticles for the detection and quantitative analysis of cell surface antigen. Biomed Res Int 2013:349408.
- Marx S, Baiker A. (2009). Beneficial interaction of gold and palladium in bimetallic catalysts for the selective oxidation of benzyl alcohol. J Phys Chem C 113:6191–201.
- Bedanta S, Kleemann W. (2009). Supermagnetism. J Phys D Appl Phys 42:013001.
- Bloemen M, Denis C, Peeters M, et al. (2015). Antibody-modified iron oxide nanoparticles for efficient magnetic isolation and flow cytometric determination of L. pneumophila. Microchim Acta 182:1439–46.
- Mahon E, Salvati A, Bombelli FB, et al. (2012). Designing the nanoparticle-biomolecule interface for “targeting and therapeutic delivery". J Control Release 161:164–74.
- Lartigue L, Innocenti C, Kalaivani T, et al. (2011). Water-dispersible sugar-coated iron oxide nanoparticles. An evaluation of their relaxometric and magnetic hyperthermia properties. J Am Chem Soc 133:10459–72.
- Larson TA, Bankson J, Aaron J, Sokolov K. (2007). Hybrid plasmonic magnetic nanoparticles as molecular specific agents for MRI/optical imaging and photothermal therapy of cancer cells. Nanotechnology 18:325101.
- Brollo M, Orozco-Henao J, López-Ruiz R, et al. (2016). Magnetic hyperthermia in brick-like Ag@ Fe3O4 core–shell nanoparticles. J Magn Magn Mater 397:20–7.
- Bruno M, Miola M, Bretcanu O, et al. (2014). Composite bone cements loaded with a bioactive and ferrimagnetic glass-ceramic. Part I: morphological, mechanical and calorimetric characterisation. J Biomater Appl 29:254–67.
- Li G, Feng S, Zhou D. (2011). Magnetic bioactive glass ceramic in the system CaO-P2O5-SiO2-MgO-CaF2-MnO2-Fe2O3 for hyperthermia treatment of bone tumor. J Mater Sci Mater Med 22:2197–206.
- Li Z, Kawashita M, Kudo TA, Kanetaka H. (2012). Sol-gel synthesis, characterisation, and in vitro compatibility of iron nanoparticle-encapsulating silica microspheres for hyperthermia in cancer therapy. J Mater Sci Mater Med 23:2461–9.
- Soleymani M, Edrissi M. (2015). Heating ability and biocompatibility study of silica-coated magnetic nanoparticles as heating mediators for magnetic hyperthermia and magnetically triggered drug delivery systems. B Mater Sci 38:1633–8.
- Veverka M, Závěta K, Kaman O, et al. (2014). Magnetic heating by silica-coated Co–Zn ferrite particles. J Phys D Appl Phys 47:065503.
- Chen X, Klingeler R, Kath M, et al. (2012). Magnetic silica nanotubes: synthesis, drug release, and feasibility for magnetic hyperthermia. ACS Appl Mater Interfaces 4:2303–9.
- Souza KC, Mohallem NDS, Sousa EMB. (2009). Mesoporous silica-magnetite nanocomposite: facile synthesis route for application in hyperthermia. J Sol-Gel Sci Techn 53:418–27.
- Liu TY, Chen CL, Lee YC, et al. (2016). First observation of physically capturing and manoeuvring bacteria using magnetic clays. ACS Appl Mater Interfaces 8:411–18.
- Gruttner C, Muller K, Teller J, Westphal F. (2013). Synthesis and functionalisation of magnetic nanoparticles for hyperthermia applications. Int J Hyperthermia 29:777–89.
- Martínez-Carmona M, Colilla M, Vallet-Regí M. (2015). Smart mesoporous nanomaterials for antitumor therapy. Nanomaterials 5:1906–37.
- Kumar S, Daverey A, Khalilzad-Sharghi V, et al. (2015). Theranostic fluorescent silica encapsulated magnetic nanoassemblies for in vitro MRI imaging and hyperthermia. RSC Adv 5:53180–8.
- Hurley KR, Ring HL, Etheridge M, et al. (2016). Predictable heating and positive MRI contrast from a mesoporous silica-coated iron oxide nanoparticle. Mol Pharm 13:2172–83.
- Haase C, Nowak U. (2012). Role of dipole-dipole interactions for hyperthermia heating of magnetic nanoparticle ensembles. Phys Rev B 85:045435.
- Arteaga-Cardona F, Rojas-Rojas K, Costo R, et al. (2016). Improving the magnetic heating by disaggregating nanoparticles. J Alloys Compd 663:636–44.
- Lartigue L, Hugounenq P, Alloyeau D, et al. (2012). Cooperative organisation in iron oxide multi-core nanoparticles potentiates their efficiency as heating mediators and MRI contrast agents. ACS Nano 6:10935–49.
- Yallapu MM, Othman SF, Curtis ET, et al. (2011). Multi-functional magnetic nanoparticles for magnetic resonance imaging and cancer therapy. Biomaterials 32:1890–905.
- Mancuso L, Knobloch T, Buchholz J, et al. (2014). Preparation of thermocleavable conjugates based on ansamitocin and superparamagnetic nanostructured particles by a chemobiosynthetic approach. Chemistry 20:17541–51.
- Hanafi-Bojd MY, Jaafari MR, Ramezanian N, et al. (2015). Surface functionalised mesoporous silica nanoparticles as an effective carrier for epirubicin delivery to cancer cells. Eur J Pharm Biopharm 89:248–58.
- Deka S, Singh RK, Kannan S. (2015). In-situ synthesis, structural, magnetic and in vitro analysis of α-Fe2O3–SiO2 binary oxides for applications in hyperthermia. Ceram Int 41:13164–70.
- Pollert E, Kaman O, Veverka P, et al. (2010). Core–shell La(1-x)Sr(x)MnO3 nanoparticles as colloidal mediators for magnetic fluid hyperthermia. Philos Trans a Math Phys Eng Sci 368:4389–405.
- Kuo CY, Liu TY, Chan TY, et al. (2016). Magnetically triggered nanovehicles for controlled drug release as a colorectal cancer therapy. Colloids Surf B Biointerfaces 140:567–73.
- Qiu QW, Xu XW, He M, Zhang HW. (2015). Radio-frequency-heating capability of silica-coated manganese ferrite nanoparticles. Chinese Phys B 24:067503.
- Villanueva A, De La Presa P, Alonso J, et al. (2010). Hyperthermia HeLa cell treatment with silica-coated manganese oxide nanoparticles. J Phys Chem C 114:1976–81.
- Kaman O, Veverka P, Jirák Z, et al. (2010). The magnetic and hyperthermia studies of bare and silica-coated La0.75Sr0.25MnO3 nanoparticles. J Nanopart Res 13:1237–52.
- Yu JH, Lee JS, Choa YH, Hofmann H. (2010). Synthesis and characterisation of SiO2 coated γ-Fe2O3 nanocomposite powder for hyperthermic application. J Mater Sci Technol 26:333–6.
- Kaman O, Pollert E, Veverka P, et al. (2009). Silica encapsulated manganese perovskite nanoparticles for magnetically induced hyperthermia without the risk of overheating. Nanotechnology 20:275610.
- Tao C, Zhu Y. (2014). Magnetic mesoporous silica nanoparticles for potential delivery of chemotherapeutic drugs and hyperthermia. Dalton Trans 43:15482–90.
- Larumbe S, Gomez-Polo C, Perez-Landazabal JI, Pastor JM. (2012). Effect of a SiO2 coating on the magnetic properties of Fe3O4 nanoparticles. J Phys Condens Matter 24:266007.
- Gonzalez-Fernandez M, Torres T, Andrés-Vergés M, et al. (2009). Magnetic nanoparticles for power absorption: optimising size, shape and magnetic properties. J Solid State Chem 182:2779–84.
- Arcos D, Fal-Miyar V, Ruiz-Hernández E, et al. (2012). Supramolecular mechanisms in the synthesis of mesoporous magnetic nanospheres for hyperthermia. J Mater Chem 22:64–72.
- Rosensweig RE. (2002). Heating magnetic fluid with alternating magnetic field. J Magn Magn Mater 252:370–4.
- Fannin P. (1991). Measurement of the Neel relaxation of magnetic particles in the frequency range 1 kHz to 160 MHz. J Phys D Appl Phys 24:76–7.
- Fortin JP, Gazeau F, Wilhelm C. (2008). Intracellular heating of living cells through Néel relaxation of magnetic nanoparticles. Eur Biophys J 37:223–8.
- Li Z, Kawashita M, Araki N, et al. (2010). Magnetic SiO2 gel microspheres for arterial embolization hyperthermia. Biomed Mater 5:065010.
- Majeed J, Pradhan L, Ningthoujam RS, et al. (2014). Enhanced specific absorption rate in silanol functionalized Fe3O4 core-shell nanoparticles: study of Fe leaching in Fe3O4 and hyperthermia in L929 and HeLa cells. Colloids Surf B Biointerfaces 122:396–403.
- Andrade AL, Fabris JD, Pereira MC, et al. (2012). Preparation of composite with silica-coated nanoparticles of iron oxide spinels for applications based on magnetically induced hyperthermia. Hyperfine Interact 218:71–82.
- Erné BH, Butter K, Kuipers BW, Vroege GJ. (2003). Rotational diffusion in iron ferrofluids. Langmuir 19:8218–25.
- Wang X, Gu H, Yang Z. (2005). The heating effect of magnetic fluids in an alternating magnetic field. J Magn Magn Mater 293:334–40.
- Etheridge ML, Hurley KR, Zhang J, et al. (2014). Accounting for biological aggregation in heating and imaging of magnetic nanoparticles. Technology 2:214–28.
- Miller KP, Wang L, Benicewicz BC, Decho AW. (2015). Inorganic nanoparticles engineered to attack bacteria. Chem Soc Rev 44:7787–807.
- Ruiz-Hernandez E, Baeza A, Vallet-Regí M. (2011). Smart drug delivery through DNA/magnetic nanoparticle gates. ACS Nano 5:1259–66.
- Xu Y, Zhu Y, Kaskel S. (2015). A smart magnetic nanosystem with controllable drug release and hyperthermia for potential cancer therapy. RSC Adv 5:99875–83.
- Rodrigues HF, Mello FM, Branquinho LC, et al. (2013). Real-time infrared thermography detection of magnetic nanoparticle hyperthermia in a murine model under a non-uniform field configuration. Int J Hyperthermia 29:752–67.
- Zhu Y, Tao C. (2015). DNA-capped Fe 3 O 4/SiO 2 magnetic mesoporous silica nanoparticles for potential controlled drug release and hyperthermia. RSC Adv 5:22365–72.
- Guo W, Yang C, Lin H, Qu F. (2014). P(EO-co-LLA) functionalized Fe3O4@mSiO2 nanocomposites for thermo/pH responsive drug controlled release and hyperthermia. Dalton Trans 43:18056–65.
- Baeza A, Guisasola E, Ruiz-Hernández E, Vallet-Regí M. (2012). Magnetically triggered multidrug release by hybrid mesoporous silica nanoparticles. Chem Mater 24:517–24.
- Lu F, Popa A, Zhou S, et al. (2013). Iron oxide-loaded hollow mesoporous silica nanocapsules for controlled drug release and hyperthermia. Chem Commun (Camb) 49:11436–8.
- Thomas CR, Ferris DP, Lee JH, et al. (2010). Noninvasive remote-controlled release of drug molecules in vitro using magnetic actuation of mechanized nanoparticlesinvasive. J Am Chem Soc 132:10623–5.
- Le Renard PE, Buchegger F, Petri-Fink A, et al. (2009). Local moderate magnetically induced hyperthermia using an implant formed in situ in a mouse tumour model. Int J Hyperthermia 25:229–39.
- Chen Z-P, Roemer RB. (1992).The effects of large blood vessels on temperature distributions during simulated hyperthermia. J Biomech Eng 114:473–81.
- Kolios M, Sherar M, Hunt J. (1995). Large blood vessel cooling in heated tissues: a numerical study. Phys Med Biol 40:477–94.
- Le Renard P-E, Lortz R, Senatore C, et al. (2011). Magnetic and in vitro heating properties of implants formed in situ from injectable formulations and containing superparamagnetic iron oxide nanoparticles (SPIONs) embedded in silica microparticles for magnetically induced local hyperthermia. J Magn Magn Mater 323:1054–63.
- Martin-Saavedra FM, Ruiz-Hernandez E, Bore A, et al. (2010). Magnetic mesoporous silica spheres for hyperthermia therapy. Acta Biomater 6:4522–31.
- Prasad N, Rathinasamy K, Panda D, Bahadur D. (2007). Mechanism of cell death induced by magnetic hyperthermia with nanoparticles of γ-Mn x Fe 2–x O 3 synthesised by a single step process. J Mater Chem 17:5042–51.
- Bae KH, Park M, Do MJ, et al. (2012). Chitosan oligosaccharide-stabilized ferrimagnetic iron oxide nanocubes for magnetically modulated cancer hyperthermia. ACS Nano 6:5266–73.
- Shanta Singh N, Kulkarni H, Pradhan L, Bahadur D. (2013). A multifunctional biphasic suspension of mesoporous silica encapsulated with YVO4:Eu3+ and Fe3O4 nanoparticles: synergistic effect towards cancer therapy and imaging. Nanotechnology 24:065101.
- Arami H, Khandhar A, Liggitt D, Krishnan KM. (2015). In vivo delivery, pharmacokinetics, biodistribution and toxicity of iron oxide nanoparticles. Chem Soc Rev 44:8576–607.
- Ling D, Hyeon T. (2013). Chemical design of biocompatible iron oxide nanoparticles for medical applications. Small 9:1450–66.
- Petersen EJ, Nelson BC. (2010). Mechanisms and measurements of nanomaterial-induced oxidative damage to DNA. Anal Bioanal Chem 398:613–50.