Abstract
Eradication of all malignant cells is the ultimate but challenging goal of anti-cancer treatment; most traditional clinically-available approaches fail because there are cells in a tumour that either escape therapy or become therapy-resistant. A subpopulation of cancer cells, the cancer stem cells (CSCs), is considered to be of particular significance for tumour initiation, progression and metastasis. CSCs are considered in particular to be therapy-resistant and may drive disease recurrence, which positions CSCs in the focus of anti-cancer research, but successful CSC-targeting therapies are limited. Here, we argue that hyperthermia – a therapeutic approach based on local heating of a tumour – is potentially beneficial for targeting CSCs in solid tumours. First, hyperthermia has been described to target cells in hypoxic and nutrient-deprived tumour areas where CSCs reside and ionising radiation and chemotherapy are least effective. Second, hyperthermia can modify factors that are essential for tumour survival and growth, such as the microenvironment, immune responses, vascularisation and oxygen supply. Third, hyperthermia targets multiple DNA repair pathways, which are generally upregulated in CSCs and protect them from DNA-damaging agents. Addition of hyperthermia to the therapeutic armamentarium of oncologists may thus be a promising strategy to eliminate therapy-escaping and -resistant CSCs.
Introduction
Despite advances in cancer treatment, overall survival of cancer patients remains limited and relapse occurs frequently, indicating that there are cancer cells in a tumour that escape from therapy or become therapy-resistant [Citation1]. It is now generally assumed that at least a fraction of these cells are tumour-initiating cells, or so-called cancer stem cells (CSCs) [Citation2]. CSCs are characterised by reduced or even arrested cell cycle progression and by increased DNA repair capacity, which render CSCs more resistant to therapy than the bulk of the tumour [Citation3,Citation4]. CSCs are found in oxygen-deprived (hypoxic) tumour areas, where they are surrounded by a specific microenvironment, the CSC niche, that supports and controls their growth [Citation5,Citation6]. CSCs and their niches have recently been visualised histochemically in primary brain tumours in hypoxic areas around arterioles, which are transport vessel and not oxygen- and carbon dioxide-exchanging vessels [Citation7,Citation8]. As CSCs are associated with aggressiveness of tumours and are negatively prognostic for overall survival [Citation9,Citation10], there is an urgent need for therapies targeting this CSC population, but so far only few successful approaches have been developed, such as in leukaemia where clinical trials are presently running that are focussed on forcing CSCs out of the haematopoietic stem cell niches [Citation11]. A successful anti-CSC therapy should be characterised by the ability to (i) target cells in areas populated by CSCs which are often difficult to reach by conventional therapies; (ii) destroy or modify the tumour microenvironment that sustains CSCs; (iii) kill cycling and non-cycling cells; and (iv) overcome the efficient DNA repair pathways that protect CSCs’ genomes. Here we argue that hyperthermia, defined as local heating of the tumour to 40–43 °C, is one anti-cancer treatment that may fit this challenging bill.
Cancer stem cells
The idea that a unique subset of cancer cells drives tumour (re)growth crystallised only a decade ago [Citation12–14], although the first hints of a unique population was found a decade earlier in acute myelogenous leukaemia [Citation15]. It was shown that CD34+/CD38− leukemic cells have the capacity of tumour-initiation and expansion, even though they only represent a small fraction of transformed cells that are hiding in hypoxic haematopoietic stem cell niches. All other leukemic cells that were not CD34+/CD38− lacked the capacity to re-establish the disease [Citation15,Citation16]. The study of Lapidot et al. [Citation15] described typical CSC features that are now routinely used to identify this population. CSCs share some hallmarks of normal tissue stem cells, like the potential of self-renewal, unlimited cell proliferation and the ability to re-populate and re-establish entire tissue or organ (or, in this case, tumour) structures, even from a single cell [Citation13,Citation17,Citation18]. They may propagate by cell proliferation or originate from non-CSCs by de-differentiation [Citation19–21] and their plasticity is stimulated by the microenvironment [Citation21]. Microenvironmental factors contributing to plasticity include low pH [Citation22], hypoxia [Citation6] and inflammation [Citation23]. Besides microenvironmental factors, de-differentiation of non-CSCs into CSCs is also induced by anti-cancer treatments, such as radiation therapy [Citation24–28] and chemotherapy [Citation29]. Some CSCs are characterised by reduced or arrested cell cycle progression and by increased DNA repair capacity, which makes them more resistant to therapies than the bulk of the tumour [Citation3,Citation4]. Glioblastoma CSCs are found in hypoxic tumour areas, supported by the vascular stem cell niche, containing endothelial cells [Citation5,Citation6]. Several markers have been shown to identify CSCs in various tumour types [Citation30]. Some of these markers seem to characterise CSCs in multiple cancer types, while some tumours have their own stem cell signature which correlates with aggressiveness of the disease and can predict the survival of patients [Citation9]. CSCs are considered to drive tumour recurrence and therapy resistance and some of the CSC markers, like aldehyde dehydrogenase (ALDH) activity, are indicative of radioresistance [Citation31] and chemoresistance [Citation32], hinting that this population may be difficult to eradicate by conventional anticancer therapies.
Hyperthermia
Hyperthermia, defined here as local heating of the tumour to relatively moderate temperatures of 40–43 °C for approximately one hour, is an excellent radiosensitiser and chemosensitiser effective in multiple tumour types [Citation33–41]. Clinically, hyperthermia is always combined with either radiotherapy or chemotherapy [Citation35,Citation42–44]. When applied prior to conventional therapy, hyperthermia can increase blood flow, improve oxygenation and enhance the therapy effects by stimulating production of oxygen radicals [Citation45–48]. An increased blood flow creates higher oxygen levels, changes the pH in the tumour, influencing hypoxic, malnourished and acidosis [Citation49–51]. When hyperthermia is applied simultaneously with or shortly after radiotherapy, hyperthermia can interfere with the repair of therapy-induced DNA damage, thereby indirectly contributing to tumour cell killing [Citation52,Citation53]. Hyperthermia can also activate heat shock proteins (HSPs), triggering protein unfolding, thereby causing loss of functionality and cell death [Citation54]. In all cases, hyperthermia can additionally enhance therapy responses by directly targeting the resistant hypoxic population [Citation55]. Whatever the mechanisms, the treatment results in significantly improved local tumour control, reduction of metastasis and improved overall survival. For instance, in sarcoma patients the addition of hyperthermia to radiotherapy resulted in a 50% lower probability to develop distant metastases [Citation56] and in cervical cancer patients it caused fewer recurrences, lower metastasis formation and higher overall survival than radiotherapy alone [Citation57]. A phase III clinical trial demonstrated a 15% increase in local progression-free survival for soft-tissue sarcoma patients when hyperthermia was added to chemotherapy [Citation37]. It is tempting to hypothesise that at least some of these effects are mediated by hyperthermia-mediated suppression of the CSC population via multiple avenues [Citation58], which will be discussed in the next paragraphs. An indication is that CSCs appear to be more sensitive to hyperthermia than their counterpart normal stem cells [Citation59].
Targeting the pro-CSC factors by hyperthermia
Quiescence and resistance to therapies targeting cycling cells
Like normal stem cells, CSCs exploit protective mechanisms that contribute to their survival and therapy resistance. Among these mechanisms is the maintenance of a quiescent state. Quiescent stem cells are characterised by their low RNA content and their lack of proliferation markers [Citation60]. Quiescence may be a protective response triggered, at least in part, by hypoxia (see also section “Hypoxia”) and poor nutrient supply [Citation61] or a high cell density [Citation62]. Since non-cycling cells are generally less susceptible to radiation and chemotherapeutic DNA-damaging agents [Citation63–65], quiescence may be an important contributor to CSC therapy resistance [Citation3]. Paradoxically, eradication of the cycling tumour cells arrests disease progression but it can also stimulate proliferation of quiescent cells that evaded therapy, which can then repopulate the tumour [Citation66–68].
Subsequently, cells enter S-phase, which is associated with increased (radio) resistance [Citation69]. A combination of irradiation with hyperthermia activates cells from quiescence to S-phase [Citation70]. Proliferating cells have been found to be sensitive to hyperthermia [Citation71] and these cells are also sensitive to chemotherapeutic agents and radiation. Although not all effects of hyperthermia are completely clear, previous studies have demonstrated that hyperthermia prolongs the cell cycle at G1/S transition [Citation72–74] and block cells in G2 [Citation75] Moreover, hyperthermia has been found to decrease the hypoxic fraction of quiescent cells [Citation76,Citation77]. Therefore, hyperthermia in combination with conventional therapy may push CSCs out of quiescent state, after which they become more vulnerable to anti-cancer therapies.
Hypoxia
Oxygen supply is a key factor that affects tumour homeostasis and microenvironment. Rapidly dividing solid malignancies struggle to maintain sufficient oxygenation due to poor vascularisation, increasing the likelihood of hypoxic regions [Citation78–82]. Tumour types in which hypoxia is common include malignant brain tumours [Citation83], melanomas [Citation84], cervical carcinomas [Citation85] and soft tissue sarcomas [Citation86]. Hypoxia is also correlated with poor prognosis and metastasis [Citation87–89]. For instance, patients with hypoxic soft tissue sarcomas have a significantly higher incidence of lung metastasis and shorter disease-free survival than those with less hypoxic tumours [Citation56,Citation86]. Similarly, patients with hypoxic lymph node-negative cervical cancer treated with radiotherapy have a significantly higher risk of distant metastasis than patients with better oxygenated tumours [Citation85]. Since oxygen stimulates induction of DNA damage by ionising radiation and some chemotherapeutic agents [Citation90], hypoxia can decrease the efficacy of treatment and thus protect tumour cells from therapy [Citation91–93].
Hypoxic areas are indispensable in any type of stem cell niche – either embryonic, adult or cancer. Hypoxia preserves the undifferentiated state of stem cells, reduces oxidative DNA damage [Citation5] and stimulates upregulation of markers associated with a stem-like phenotype [Citation94]. Importantly, the self-renewal and differentiation capacity of CSCs has been found to increase in regions deprived of oxygen [Citation5]. In addition, hypoxia-inducible factor 1α (HIF1α), a protein abundantly expressed after radiation exposure [Citation95] and under hypoxic conditions, contributes to CSC proliferation and survival [Citation96]. HIF1α can directly upregulate the activity of Notch signalling, a pathway promoting survival and self-renewal of CSCs [Citation97], and CSCs with a reduced HIF1 activity were unable to form tumours in vitro [Citation98]. As a consequence, hypoxia has been suggested to promote the phenotype of CSCs, to enhance their tumorigenicity and to be a biomarker of radioresistance [Citation99,Citation100].
Hyperthermia appears to counteract some of the effects of hypoxia, primarily by stimulating the blood flow and increasing the leakiness of tumour blood vessels [Citation101]. Hyperthermia has been shown to improve tumour oxygen supply in several clinical studies [Citation102–104]. This was not only evident in chronically hypoxic areas, where hyperthermia decreased the amount of quiescent cells considerably, but was also detectable in the total tumour cell population [Citation76]. In addition, re-oxygenation induces microenvironmental changes, such as decreased acidosis and induces cell cycle arrest, resulting in autophagy and eventually apoptosis [Citation105].
Immune responses
Tumorigenesis can only occur when cancer cells evade the immune system. The immune response is critical for eliminating cancer cells based on the expression of tumour-associated antigens [Citation106]. Additionally, inflammatory responses of the immune system can increase the burden of DNA damage and, as a consequence, induce tumorigenesis [Citation107]. On the other hand, inflammation can cause an increase in body temperature, which may activate specific HSPs, such as HSP70 [Citation108]. HSPs are potent immune modulators and can lead to stimulation of both the innate and adaptive immune responses against transformed cells. However, once a malignancy develops, immune cells, such as tumour-associated macrophages (TAMs), can also promote malignant cell growth by stimulating angiogenesis, invasion and metastasis [Citation109], and high levels of TAMs are correlated with poor prognosis [Citation110]. CD8+ and IFNɣ-producing helper T cells are also present frequently in the tumour microenvironment and they exert tumour and metastasis: promoting effects [Citation111–114]. A recent study demonstrated that tumour-bearing mice had higher CSC-specific IgG levels in serum after vaccination with CSC lysate-activated dendritic cells (DCs). When administered complement, these mice eradicated CSCs more efficiently than mice vaccinated with whole tumour-activated DCs [Citation115]. Furthermore, CSCs were targeted more efficiently in vitro by cytotoxic T cells from these CSC-vaccinated mice. CSC-derived antigens can thus directly stimulate anti-CSC immune responses. On the other hand, CSCs have been reported to suppress immune system functionality [Citation116–118].
Anti-tumour immune responses can be stimulated by radiotherapy or chemotherapy [Citation119] and enhanced by mild hyperthermia. Hyperthermia triggers the immune system by stimulating the production of HSPs [Citation120] and their release by necrotic tumour cells [Citation121,Citation122]. HSPs, in turn, can trigger maturation of DCs, resulting in systemic anti-tumour response [Citation123–125]. Furthermore, hyperthermia can enhance antigen display, facilitating recognition of malignant cells by the immune system [Citation126,Citation127]. In animal experiments, hyperthermia also increased levels of natural killer cells, resulting in significant tumour growth inhibition [Citation128]. In conclusion, these results suggest that hyperthermia can be exploited to potentiate anti-tumour and anti-CSC immune responses.
Microenvironment
The CSC population co-exists in a symbiotic relationship with the tumour microenvironment, which preserves and regulates their plasticity, but is also affected by their presence [Citation21,Citation129]. Many mechanisms are involved in this bi-directional communication, as summarised by Plaks et al. [Citation130]. Endothelial cells play an essential role in CSC proliferation [Citation131]. They secrete nitric oxide, activating the Notch signalling pathway [Citation132] which controls cell fate, self-renewal, angiogenesis and endothelium interactions in the CSC microenvironment. In glioblastoma, the Notch signalling causes radioresistance in CSCs [Citation133] and inhibition of this pathway or angiogenesis can deplete the CSC population [Citation134,Citation135]. Furthermore, fibroblasts that inhabit the tumour microenvironment are essential for cell proliferation and for promotion of angiogenesis [Citation136].
Both endothelial cells and fibroblasts have been found to be extremely sensitive to hyperthermia [Citation137]. Hyperthermia can additionally inhibit endothelial cell adhesion [Citation138] and cell proliferation and promote apoptosis [Citation139]. The resulting reorganisation of CSC microenvironment can deprive CSCs of the factors that are essential for the maintenance of their stem-like potential and that promote their resistance to therapy.
Viral infections
Approximately 15% of human cancers are caused by infections with oncoviruses [Citation140]. Infections with hepatitis B virus [Citation141], Epstein–Barr virus [Citation142], papilloma virus [Citation143] or herpes virus-8 [Citation144] can initiate cancer. Fortunately, in most cases a healthy immune system is capable of eliminating these viruses. However, in a small number of cases, viral infections can slowly progress into cancer, which can take years or even decades [Citation145].
Human papilloma virus (HPV) is a pathogen that has been shown to affect CSCs homeostasis. Recent studies suggest that HPV plays an important role in stimulating CSCs. This may be caused by production of the viral oncoproteins E6 and E7 that interfere with tumour suppressor proteins p53 and retinoblastoma protein (Rb), abolishing cell cycle regulation and apoptosis [Citation146–148]. Overexpression of E6 was found to promote stemness and self-renewal in HPV+ cervical CSCs [Citation149] and the ALDH1+ CSC population in HPV+ tumours was considerably higher, than in HPV− tumours [Citation150]. Furthermore, various transcription factors that are overexpressed in non-CSCs and CSCs, such as Oct4, Nanog and Notch – essential for self-renewal and for re-establishing pluripotency – are altered by HPV infections [Citation21]. HPV+ and HPV− tumours differ in therapy sensitivity, which may be at least partly due to CSC fractions in these tumours [Citation151–153]. Our recent study showed that hyperthermia temporary inactivates the viral oncoprotein E6 [Citation75]. Since E6 normally suppresses p53-mediated cell death in cervical cancer, it is plausible that hyperthermia may also block HPV-induced immortality.
Hyperthermia stimulates immune function and tumour immunogenicity which may affect the tumour microenvironment, and other viruses may be tackled successfully as well [Citation154]. However, further investigations are needed to elucidate the anti-viral effects and eradication of viral related cancer cells.
DNA repair
CSCs are often characterised by enhanced activity of DNA repair pathways [Citation1,Citation155]. Increased expression of major DNA repair proteins, including BRCA1, ATR and ATM, has been observed in pancreatic, breast and prostate CSCs [Citation156–158]. Reduced DNA repair capacity in CSCs has also been reported (reviewed by Wang [Citation159]). In any case, DNA-damaging therapies targeting CSCs certainly benefit from inhibition of DNA repair as well as from increasing the total DNA damage burden. Hyperthermia can likely perform both. First, elevated temperatures have been shown to inhibit pathways that are responsible for repairing DNA lesions that are relevant in clinical cancer treatment [Citation160]. More specifically, hyperthermia induces degradation of the BRCA2 protein and thereby inactivates homologous recombination, one of the major pathways responsible for repairing DNA double-strand breaks [Citation161,Citation162]. Furthermore, hyperthermia (above 43 °C) has been found to interfere with base excision repair [Citation163,Citation164], leading to more severe breaks. Interference with DNA repair pathways is important to increase levels of DNA breaks, causing accumulation of unrepaired DNA lesions, resulting in cell death. Hyperthermia has been found to decrease the CSC population due to inhibition of DNA repair [Citation28,Citation58,Citation165,Citation166]. Hyperthermia not only inhibits repair of DNA damage caused by radiation but also suppresses AKT signalling, a radiation-induced survival mechanism preferentially utilised by the CSC population [Citation167]. Second, it is becoming clear that hyperthermia can induce DNA damage by causing protein denaturation and interfering with DNA replication (reviewed by Oei et al. [Citation168]). There has been a long debate whether hyperthermia induces DNA damage or not, as increased (unrepaired) DNA damage is observed after combinational therapies that include hyperthermia in nearly all studies that tested the effectiveness of hyperthermia. Direct methods to detect immediate induction of DNA breaks failed in most of the studies as mild hyperthermia interferes predominantly with DNA repair pathways. Therefore, the effects of hyperthermia on (unrepaired) DNA damages may therefore be caused by indirect effects ([Citation169] and reviewed by Oei et al. [Citation168]).
Summary and future perspectives
Conventional radiotherapy and chemotherapy target the bulk of the tumour but not the therapy-resistant CSCs, often resulting in tumour relapse (). After tumour relapse, conventional therapies may be combined with hyperthermia, thereby increasing effectiveness of therapy. We argue that first-line conventional treatment combined with hyperthermia may directly sensitise CSCs by inducing leaky vessels that allow chemotherapeutics to reach deeper regions of the tumour; oxygen levels will rise (re-oxygenation) thereby increasing sensitivity to radiotherapy; increased number of DNA breaks and decrease of DNA repair cause accumulation of DNA damages; quiescent cells may repopulate after first doses of therapy and the immune system may be triggered to interfere with the CSCs microenvironment. All these factors may also prevent CSCs from acquiring resistance to therapy.
Figure 1. Schematic overview of targeting CSCs by hyperthermia. The tumour consists of many different cell types including CSCs. Conventional first-line radiotherapy and chemotherapy are able to destroy all tumour cells and especially CSCs that often reside in hypoxic areas. These cells are considered to drive tumour recurrence and metastasis formation. Combined with conventional first-line treatment, hyperthermia can target CSCs via multiple avenues, including stimulation of blood flow and re-oxygenation of hypoxic areas, causing blood vessel leakage, triggering the immune response and inhibiting DNA repair.
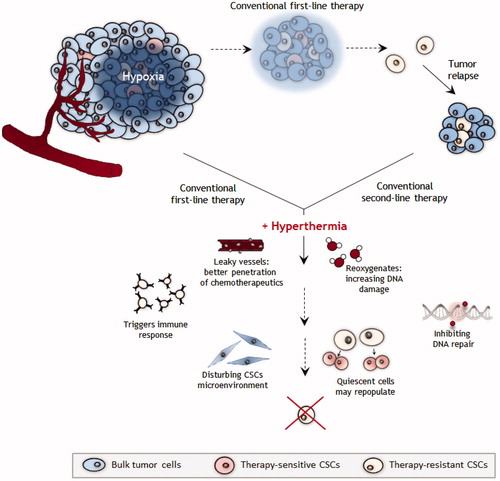
Hyperthermia is successful in sensitising tumours with hypoxic areas to radiotherapy and chemotherapy. The question is whether the effectiveness of hyperthermia on hypoxic tumour tissue is mediated through a direct effect of hyperthermia on CSCs or by a change in the tumour microenvironment after which the CSCs become more sensitive to radiotherapy or chemotherapy treatment, or both. One would expect that in case of a direct effect, hyperthermia affects the CSC population even without re-oxygenation of the CSC niche. However, clinical data seem to suggest that this re-oxygenation is needed for the effectiveness of hyperthermia [Citation104]. But we cannot be certain that this precludes a direct effect as radiotherapy is less effective in the absence of oxygen radicals and chemotherapy may not be delivered to poorly-perfused hypoxic areas. In other words, even when hyperthermia directly eliminates CSCs, the traditional modalities remain ineffective without reperfusion and re-oxygenation, and these modalities are needed to eliminate the non-CSC tumour cell population.
This suggests that hyperthermia can sensitise CSCs directly to traditional cancer therapy and that this sensitisation may be the underlying mechanism responsible for at least part of the clinical success of hyperthermia. However, a thorough understanding of the mechanisms driving this sensitisation is lacking. It is difficult to derive conclusions from the presently available data because any effects of hyperthermia on the CSCs are difficult to distinguish from other suggested mechanisms that can account for the established effects of hyperthermia on hypoxic tumour areas. This distinction requires further research, that focuses on determining reliable and specific CSC markers in preclinical in vitro and in vivo tumour models under various microenvironmental conditions.
Isolation of CSCs on the basis of expression of specific CSCs markers, can help to dissect how hyperthermia affects CSCs. However, it is essential to study the microenvironmental factors to eliminate and target the complete tumour. In vivo experiments should be focussed on comparison of the effectiveness of radiotherapy and hyperthermia in aerobic and hypoxic tumour regions. The first important step to accomplish this is to visualise these hypoxic areas [Citation170] to facilitate investigations whether hyperthermia indeed targets the cells in these areas. Imaging the dynamics of tumour responses in different parts of the tumour is also important. Furthermore, specific markers of CSCs are necessary to detect in order to observe whether CSCs are preferentially located in the tumour areas targeted by hyperthermia and to monitor whether the frequencies of cells that show stemness characteristics decrease sufficiently in numbers that they lose their ability to repopulate tumours.
Unravelling this mechanism may have major clinical impact as it will permit more effective multi-modality strategies against therapy-resistant tumour types.
Novelty and impact
Successful cancer therapy requires effective targeting of cancer stem cells. Cancer stem cells are resistant to conventional radiation and chemotherapy. Hyperthermia is an alternative therapeutic strategy to target this resistant cancer cell population.
Acknowledgements
The authors wish to thank Prof. Dr. C.J.F. van Noorden for discussion and useful suggestions on the manuscript. This work was supported by the Dutch Cancer Society (grants #UVA 2008-4019, #UVA 2012-5540 and #UVA 2011-4962), NWO Medium grant (#40-00506-98-16015) and the Maurits en Anna de Kock foundation.
Disclosure statement
No potential conflict of interests were disclosed.
Additional information
Funding
References
- Bao S, Wu Q, McLendon RE, et al. Glioma stem cells promote radioresistance by preferential activation of the DNA damage response. Nature 2006;444:756–60.
- Carnero A, Lleonart M. The hypoxic microenvironment: a determinant of cancer stem cell evolution. BioEssays: News Rev Mol Cell Dev Biol 2016;38:S65–S74.
- Orford KW, Scadden DT. Deconstructing stem cell self-renewal: genetic insights into cell-cycle regulation. Nat Rev Genet 2008;9:115–28.
- Cojoc M, Mabert K, Muders MH, Dubrovska A. A role for cancer stem cells in therapy resistance: cellular and molecular mechanisms. Semin Cancer Biol 2015;31:16–27.
- Gilbertson RJ, Rich JN. Making a tumour's bed: glioblastoma stem cells and the vascular niche. Nat Rev Cancer 2007;7:733–6.
- Conley SJ, Gheordunescu E, Kakarala P, et al. Antiangiogenic agents increase breast cancer stem cells via the generation of tumor hypoxia. Proc Natl Acad Sci USA 2012;109:2784–9.
- Hira VV, Ploegmakers KJ, Grevers F, et al. CD133+ and nestin + glioma stem-like cells reside around CD31+ arterioles in niches that express SDF-1alpha, CXCR4, osteopontin and cathepsin K. J Histochem Cytochem 2015;63:481–93.
- Verbovsek U, Van Noorden CJ, Lah TT. Complexity of cancer protease biology: cathepsin K expression and function in cancer progression. Semin Cancer Biol 2015;35:71–84.
- Suva ML, Riggi N, Janiszewska M, et al. EZH2 is essential for glioblastoma cancer stem cell maintenance. Cancer Res 2009;69:9211–18.
- Karnoub AE, Dash AB, Vo AP, et al. Mesenchymal stem cells within tumour stroma promote breast cancer metastasis. Nature 2007;449:557–63.
- Uy GL, Rettig MP, Motabi IH, et al. A phase 1/2 study of chemosensitization with the CXCR4 antagonist plerixafor in relapsed or refractory acute myeloid leukemia. Blood 2012;119:3917–24.
- Wicha MS, Liu S, Dontu G. Cancer stem cells: an old idea—a paradigm shift. Cancer Res 2006;66:1883–90. discussion 95–6.
- Clarke MF, Dick JE, Dirks PB, et al. Cancer stem cells—perspectives on current status and future directions: AACR Workshop on cancer stem cells. Cancer Res 2006;66:9339–44.
- Li F, Tiede B, Massague J, Kang Y. Beyond tumorigenesis: cancer stem cells in metastasis. Cell Res 2007;17:3–14.
- Lapidot T, Sirard C, Vormoor J, et al. A cell initiating human acute myeloid leukaemia after transplantation into SCID mice. Nature 1994;367:645–8.
- Bonnet D, Dick JE. Human acute myeloid leukemia is organized as a hierarchy that originates from a primitive hematopoietic cell. Nat Med 1997;3:730–7.
- Rycaj K, Tang DG. Cancer stem cells and radioresistance. Int J Radiat Biol 2014;90:615–21.
- Vermeulen L, Sprick MR, Kemper K, et al. Cancer stem cells—old concepts, new insights. Cell Death Different 2008;15:947–58.
- Gupta PB, Fillmore CM, Jiang G, et al. Stochastic state transitions give rise to phenotypic equilibrium in populations of cancer cells. Cell 2011;146:633–44.
- Kreso A, O'Brien CA, van Galen P, et al. Variable clonal repopulation dynamics influence chemotherapy response in colorectal cancer. Science 2013;339:543–8.
- Swanson MS, Kokot N, Sinha UK. The role of HPV in head and neck cancer stem cell formation and tumorigenesis. Cancers 2016;8:24.
- Hjelmeland AB, Wu Q, Heddleston JM, et al. Acidic stress promotes a glioma stem cell phenotype. Cell Death Different 2011;18:829–40.
- Iliopoulos D, Hirsch HA, Wang G, Struhl K. Inducible formation of breast cancer stem cells and their dynamic equilibrium with non-stem cancer cells via IL6 secretion. Proc Natl Acad Sci USA 2011;108:1397–402.
- Lagadec C, Vlashi E, Della Donna L, et al. Radiation-induced reprogramming of breast cancer cells. Stem Cells 2012;30:833–44.
- Salmina K, Jankevics E, Huna A, et al. Up-regulation of the embryonic self-renewal network through reversible polyploidy in irradiated p53-mutant tumour cells. Exp Cell Res 2010;316:2099–112.
- Ghisolfi L, Keates AC, Hu X, et al. Ionizing radiation induces stemness in cancer cells. PLoS One 2012;7:e43628.
- Gomez-Casal R, Bhattacharya C, Ganesh N, et al. Non-small cell lung cancer cells survived ionizing radiation treatment display cancer stem cell and epithelial-mesenchymal transition phenotypes. Mol Cancer 2013;12:94.
- Atkinson RL, Zhang M, Diagaradjane P, et al. Thermal enhancement with optically activated gold nanoshells sensitizes breast cancer stem cells to radiation therapy. Sci Transl Med 2010;2:55ra79.
- Auffinger B, Tobias AL, Han Y, et al. Conversion of differentiated cancer cells into cancer stem-like cells in a glioblastoma model after primary chemotherapy. Cell Death Different 2014;21:1119–31.
- Medema JP. Cancer stem cells: the challenges ahead. Nat Cell Biol 2013;15:338–44.
- Kurth I, Hein L, Mabert K, et al. Cancer stem cell related markers of radioresistance in head and neck squamous cell carcinoma. Oncotarget 2015;6:34494–509.
- Hilton J. Role of aldehyde dehydrogenase in cyclophosphamide-resistant L1210 leukemia. Cancer Res 1984;44:5156–60.
- Kim JH, Kim SH, Alfieri AA. Selective killing of glucose-deprived hypoxic cells by hyperthermia. I. Protection by purine ribonucleosides. Radiat Res 1988;116:337–42.
- LaRue SM, Vujaskovic Z. Combining radiation therapy with other treatment modalities. Semin Vet Med Surg Small Anim 1995;10:197–204.
- van der Zee J, Gonzalez Gonzalez D, van Rhoon GC, et al. Comparison of radiotherapy alone with radiotherapy plus hyperthermia in locally advanced pelvic tumours: a prospective, randomised, multicentre trial. Dutch Deep Hyperthermia Group. Lancet 2000;355:1119–25.
- Colombo R, Salonia A, Da Pozzo LF, et al. Combination of intravesical chemotherapy and hyperthermia for the treatment of superficial bladder cancer: preliminary clinical experience. Crit Rev Oncol/Hematol 2003;47:127–39.
- Issels RD, Lindner LH, Verweij J, et al. Neo-adjuvant chemotherapy alone or with regional hyperthermia for localised high-risk soft-tissue sarcoma: a randomised phase 3 multicentre study. Lancet Oncol 2010;11:561–70.
- Overgaard J, Gonzalez Gonzalez D, Hulshof MC, et al. Randomised trial of hyperthermia as adjuvant to radiotherapy for recurrent or metastatic malignant melanoma. European Society for Hyperthermic Oncology. Lancet 1995;345:540–3.
- Wessalowski R, Schneider DT, Mils O, et al. Regional deep hyperthermia for salvage treatment of children and adolescents with refractory or recurrent non-testicular malignant germ-cell tumours: an open-label, non-randomised, single-institution, phase 2 study. Lancet Oncol 2013;14:843–52.
- Cihoric N, Tsikkinis A, van Rhoon G, et al. Hyperthermia-related clinical trials on cancer treatment within the ClinicalTrials.gov registry. Int J Hypertherm 2015;31:609–14.
- Harima Y, Ohguri T, Imada H, et al. A multicentre randomised clinical trial of chemoradiotherapy plus hyperthermia versus chemoradiotherapy alone in patients with locally advanced cervical cancer. Int J Hypertherm 2016;32:801–8.
- Gonzalez Gonzalez D, van Dijk JD, Blank LE. Radiotherapy and hyperthermia. Eur J Cancer 1995;31A:1351–5.
- Issels R, Kampmann E, Kanaar R, Lindner LH. Hallmarks of hyperthermia in driving the future of clinical hyperthermia as targeted therapy: translation into clinical application. Int J Hypertherm 2016;32:89–95.
- Wust P, Hildebrandt B, Sreenivasa G, et al. Hyperthermia in combined treatment of cancer. Lancet Oncol 2002;3:487–97.
- Vujaskovic Z, Poulson JM, Gaskin AA, et al. Temperature-dependent changes in physiologic parameters of spontaneous canine soft tissue sarcomas after combined radiotherapy and hyperthermia treatment. Int J Radiat Oncol Biol Phys 2000;46:179–85.
- Sen A, Capitano ML, Spernyak JA, et al. Mild elevation of body temperature reduces tumor interstitial fluid pressure and hypoxia and enhances efficacy of radiotherapy in murine tumor models. Cancer Res 2011;71:3872–80.
- Winslow TB, Eranki A, Ullas S, et al. A pilot study of the effects of mild systemic heating on human head and neck tumour xenografts: analysis of tumour perfusion, interstitial fluid pressure, hypoxia and efficacy of radiation therapy. Int J Hypertherm 2015;31:693–701.
- Horsman MR. Realistic biological approaches for improving thermoradiotherapy. Int J Hypertherm 2016;32:14–22.
- Song CW, Kang MS, Rhee JG, Levitt SH. The effect of hyperthermia on vascular function, pH, and cell survival. Radiology 1980;137:795–803.
- Wike-Hooley JL, Van der Zee J, van Rhoon GC, et al. Human tumour pH changes following hyperthermia and radiation therapy. Eur J Cancer Clin Oncol 1984;20:619–23.
- Vaupel PW, Kelleher DK. Pathophysiological and vascular characteristics of tumours and their importance for hyperthermia: heterogeneity is the key issue. Int J Hypertherm 2010;26:211–23.
- Kampinga HH, Dikomey E. Hyperthermic radiosensitization: mode of action and clinical relevance. Int J Radiat Biol 2001;77:399–408.
- Roti Roti JL. Introduction: radiosensitization by hyperthermia. Int J Hypertherm 2004;20:109–14.
- Dubois MF, Hovanessian AG, Bensaude O. Heat-shock-induced denaturation of proteins. Characterization of the insolubilization of the interferon-induced p68 kinase. J Biol Chem 1991;266:9707–11.
- Horsman MR, Overgaard J. Hyperthermia: a potent enhancer of radiotherapy. Clin Oncol (R Coll Radiol) 2007;19:418–26.
- Brizel DM, Scully SP, Harrelson JM, et al. Tumor oxygenation predicts for the likelihood of distant metastases in human soft tissue sarcoma. Cancer Res 1996;56:941–3.
- van der Zee J, Gonzalez GD. The Dutch Deep Hyperthermia Trial: results in cervical cancer. Int J Hypertherm 2002;18:1–12.
- Dewhirst MW, Lee CT, Ashcraft KA. The future of biology in driving the field of hyperthermia. Int J Hypertherm 2016;32:4–13.
- Wierenga PK, Setroikromo R, Kamps G, et al. Differences in heat sensitivity between normal and acute myeloid leukemic stem cells: feasibility of hyperthermic purging of leukemic cells from autologous stem cell grafts. Exp Hematol 2003;31:421–7.
- Cheung TH, Rando TA. Molecular regulation of stem cell quiescence. Nat Rev Mol Cell Biol 2013;14:329–40.
- Vaupel P. Tumor microenvironmental physiology and its implications for radiation oncology. Semin Radiat Oncol 2004;14:198–206.
- Stoker MG. The Leeuwenhoek lecture, 1971. Tumour viruses and the sociology of fibroblasts. Proc R Soc Lond B, Biol Sci 1972;181:1–17.
- Clevers H. The cancer stem cell: premises, promises and challenges. Nat Med 2011;17:313–19.
- Baguley BC. Multiple drug resistance mechanisms in cancer. Mol Biotechnol 2010;46:308–16.
- Barendsen GW, Van Bree C, Franken NA. Importance of cell proliferative state and potentially lethal damage repair on radiation effectiveness: implications for combined tumor treatments (review). Int J Oncol 2001;19:247–56.
- Kim MJ, Kim RK, Yoon CH, et al. Importance of PKCdelta signaling in fractionated-radiation-induced expansion of glioma-initiating cells and resistance to cancer treatment. J Cell Sci 2011;124:3084–94.
- Phillips TM, McBride WH, Pajonk F. The response of CD24(-/low)/CD44+ breast cancer-initiating cells to radiation. J Natl Cancer Inst 2006;98:1777–85.
- Vlashi E, Kim K, Lagadec C, et al. In vivo imaging, tracking, and targeting of cancer stem cells. J Natl Cancer Inst 2009;101:350–9.
- Baumann M, Krause M, Hill R. Exploring the role of cancer stem cells in radioresistance. Nat Rev Cancer 2008;8:545–54.
- Zolzer F, Streffer C, Pelzer T. Induction of quiescent S-phase cells by irradiation and/or hyperthermia. II. Correlation with colony forming ability. Int J Radiat Biol 1993;63:77–82.
- Bhuyan BK. Kinetics of cell kill by hyperthermia. Cancer Res 1979;39:2277–84.
- Sisken JE, Morasca L, Kibby S. Effects of temperature on the kinetics of the mitotic cycle of mammalian cells in culture. Exp Cell Res 1965;39:103–16.
- Higashikubo R, Holland JM, Roti Roti JL. Comparative effects of caffeine on radiation- and heat-induced alterations in cell cycle progression. Radiat Res 1989;119:246–60.
- Nishita M, Inoue S, Tsuda M, et al. Nuclear translocation and increased expression of Bax and disturbance in cell cycle progression without prominent apoptosis induced by hyperthermia. Exp Cell Res 1998;244:357–66.
- Oei AL, van Leeuwen CM, ten Cate R, et al. Hyperthermia selectively targets human papillomavirus in cervical tumors via p53-dependent apoptosis. Cancer Res 2015;75:5120–9.
- Masunaga S, Ono K, Akaboshi M, et al. Reduction of hypoxic cells in solid tumours induced by mild hyperthermia: special reference to differences in changes in the hypoxic fraction between total and quiescent cell populations. Br J Cancer 1997;76:588–93.
- Masunaga S, Matsumoto Y, Hirayama R, et al. Significance of manipulating intratumor hypoxia in the effect on lung metastases in radiotherapy, with reference to its effect on the sensitivity of intratumor quiescent cells. Clin Exp Metast 2009;26:693–700.
- Brown JM, Giaccia AJ. The unique physiology of solid tumors: opportunities (and problems) for cancer therapy. Cancer Res 1998;58:1408–16.
- Li Z, Bao S, Wu Q, et al. Hypoxia-inducible factors regulate tumorigenic capacity of glioma stem cells. Cancer Cell 2009;15:501–13.
- Mao Q, Zhang Y, Fu X, et al. A tumor hypoxic niche protects human colon cancer stem cells from chemotherapy. J Cancer Res Clin Oncol 2013;139:211–22.
- Vaupel P, Harrison L. Tumor hypoxia: causative factors, compensatory mechanisms, and cellular response. Oncologist 2004;9:4–9.
- Vaupel P, Kallinowski F, Okunieff P. Blood flow, oxygen and nutrient supply, and metabolic microenvironment of human tumors: a review. Cancer Res 1989;49:6449–65.
- Rampling R, Cruickshank G, Lewis AD, et al. Direct measurement of pO2 distribution and bioreductive enzymes in human malignant brain tumors. Int J Radiat Oncol Biol Phys 1994;29:427–31.
- Lartigau E, Randrianarivelo H, Avril MF, et al. Intratumoral oxygen tension in metastatic melanoma. Melan Res 1997;7:400–6.
- Fyles A, Milosevic M, Hedley D, et al. Tumor hypoxia has independent predictor impact only in patients with node-negative cervix cancer. J Clin Oncol 2002;20:680–7.
- Nordsmark M, Alsner J, Keller J, et al. Hypoxia in human soft tissue sarcomas: adverse impact on survival and no association with p53 mutations. Br J Cancer 2001;84:1070–5.
- Ratcliffe PJ. Oxygen sensing and hypoxia signalling pathways in animals: the implications of physiology for cancer. J Physiol 2013;591:2027–42.
- Semenza GL. Baffled by bafilomycin: an anticancer agent that induces hypoxia-inducible factor-1alpha expression. Mol Pharmacol 2006;70:1841–3.
- Semenza GL. Cancer-stromal cell interactions mediated by hypoxia-inducible factors promote angiogenesis, lymphangiogenesis, and metastasis. Oncogene 2013;32:4057–63.
- Horan AD, Giandomenico AR, Koch CJ. Effect of oxygen on radiation-induced DNA damage in isolated nuclei. Radiat Res 1999;152:144–53.
- Littlewood TJ. The impact of hemoglobin levels on treatment outcomes in patients with cancer. Semin Oncol 2001;28:49–53.
- Rockwell S, Dobrucki IT, Kim EY, et al. Hypoxia and radiation therapy: past history, ongoing research, and future promise. Curr Mol Med 2009;9:442–58.
- Teicher BA, Kowal CD, Kennedy KA, Sartorelli AC. Enhancement by hyperthermia of the in vitro cytotoxicity of mitomycin C toward hypoxic tumor cells. Cancer Res 1981;41:1096–9.
- Heddleston JM, Li Z, Lathia JD, et al. Hypoxia inducible factors in cancer stem cells. Br J Cancer 2010;102:789–95.
- Moeller BJ, Cao Y, Li CY, Dewhirst MW. Radiation activates HIF-1 to regulate vascular radiosensitivity in tumors: role of reoxygenation, free radicals, and stress granules. Cancer Cell 2004;5:429–41.
- Schwab LP, Peacock DL, Majumdar D, et al. Hypoxia-inducible factor 1alpha promotes primary tumor growth and tumor-initiating cell activity in breast cancer. Breast Cancer Res: BCR 2012;14:R6.
- Quail DF, Taylor MJ, Postovit LM. Microenvironmental regulation of cancer stem cell phenotypes. Curr Stem Cell Res Ther 2012;7:197–216.
- Jogi A, Ora I, Nilsson H, et al. Hypoxia alters gene expression in human neuroblastoma cells toward an immature and neural crest-like phenotype. Proc Natl Acad Sci USA 2002;99:7021–6.
- Vinogradov S, Wei X. Cancer stem cells and drug resistance: the potential of nanomedicine. Nanomedicine (Lond) 2012;7:597–615.
- Peitzsch C, Perrin R, Hill RP, et al. Hypoxia as a biomarker for radioresistant cancer stem cells. Int J Radiat Biol 2014;90:636–52.
- Song CW. Effect of hyperthermia on vascular functions of normal tissues and experimental tumors; brief communication. J Natl Cancer Inst 1978;60:711–13.
- Oleson JR. Eugene Robertson Special Lecture. Hyperthermia from the clinic to the laboratory: a hypothesis. Int J Hypertherm 1995;11:315–22.
- Song CW, Shakil A, Osborn JL, Iwata K. Tumour oxygenation is increased by hyperthermia at mild temperatures. Int J Hypertherm 1996;12:367–73.
- Jones EL, Prosnitz LR, Dewhirst MW, et al. Thermochemoradiotherapy improves oxygenation in locally advanced breast cancer. Clin Cancer Res 2004;10:4287–93.
- Wu H, Ding Z, Hu D, et al. Central role of lactic acidosis in cancer cell resistance to glucose deprivation-induced cell death. J Pathol 2012;227:189–99.
- Vesely MD, Kershaw MH, Schreiber RD, Smyth MJ. Natural innate and adaptive immunity to cancer. Annu Rev Immunol 2011;29:235–71.
- Sakurai T, He G, Matsuzawa A, et al. Hepatocyte necrosis induced by oxidative stress and IL-1 alpha release mediate carcinogen-induced compensatory proliferation and liver tumorigenesis. Cancer Cell 2008;14:156–65.
- Bechtold DA, Rush SJ, Brown IR. Localization of the heat-shock protein Hsp70 to the synapse following hyperthermic stress in the brain. J Neurochem 2000;74:641–6.
- Condeelis J, Pollard JW. Macrophages: obligate partners for tumor cell migration, invasion, and metastasis. Cell 2006;124:263–6.
- Murdoch C, Muthana M, Coffelt SB, Lewis CE. The role of myeloid cells in the promotion of tumour angiogenesis. Nat Rev Cancer 2008;8:618–31.
- Aspord C, Pedroza-Gonzalez A, Gallegos M, et al. Breast cancer instructs dendritic cells to prime interleukin 13-secreting CD4+ T cells that facilitate tumor development. J Exp Med 2007;204:1037–47.
- DeNardo DG, Barreto JB, Andreu P, et al. CD4(+) T cells regulate pulmonary metastasis of mammary carcinomas by enhancing protumor properties of macrophages. Cancer Cell 2009;16:91–102.
- Hanada T, Kobayashi T, Chinen T, et al. IFNgamma-dependent, spontaneous development of colorectal carcinomas in SOCS1-deficient mice. J Exp Med 2006;203:1391–7.
- Roberts SJ, Ng BY, Filler RB, et al. Characterizing tumor-promoting T cells in chemically induced cutaneous carcinogenesis. Proc Natl Acad Sci USA 2007;104:6770–5.
- Ning N, Pan Q, Zheng F, et al. Cancer stem cell vaccination confers significant antitumor immunity. Cancer Res 2012;72:1853–64.
- Di Tomaso T, Mazzoleni S, Wang E, et al. Immunobiological characterization of cancer stem cells isolated from glioblastoma patients. Clin Cancer Res 2010;16:800–13.
- Kawakami Y, Matsushita M, Ueda R, et al. Immunotherapy targeting cancer stem cells. Nihon Rinsho Japan J Clin Med 2012;70:2142–6.
- Wei J, Barr J, Kong LY, et al. Glioma-associated cancer-initiating cells induce immunosuppression. Clin Cancer Res 2010;16:461–73.
- Calderwood SK, Khaleque MA, Sawyer DB, Ciocca DR. Heat shock proteins in cancer: chaperones of tumorigenesis. Trends Biochem Sci 2006;31:164–72.
- Wang HY, Fu JC, Lee YC, Lu PJ. Hyperthermia stress activates heat shock protein expression via propyl isomerase 1 regulation with heat shock factor 1. Mol Cell Biol 2013;33:4889–99.
- Sauter B, Albert ML, Francisco L, et al. Consequences of cell death: exposure to necrotic tumor cells, but not primary tissue cells or apoptotic cells, induces the maturation of immunostimulatory dendritic cells. J Exp Med 2000;191:423–34.
- Basu S, Binder RJ, Suto R, et al. Necrotic but not apoptotic cell death releases heat shock proteins, which deliver a partial maturation signal to dendritic cells and activate the NF-kappa B pathway. Int Immunol 2000;12:1539–46.
- Chen T, Guo J, Han C, et al. Heat shock protein 70, released from heat-stressed tumor cells, initiates antitumor immunity by inducing tumor cell chemokine production and activating dendritic cells via TLR4 pathway. J Immunol 2009;182:1449–59.
- Bleifuss E, Bendz H, Sirch B, et al. Differential capacity of chaperone-rich lysates in cross-presenting human endogenous and exogenous melanoma differentiation antigens. Int J Hypertherm 2008;24:623–37.
- Schueller G, Stift A, Friedl J, et al. Hyperthermia improves cellular immune response to human hepatocellular carcinoma subsequent to co-culture with tumor lysate pulsed dendritic cells. Int J Oncol 2003;22:1397–402.
- Shi H, Cao T, Connolly JE, et al. Hyperthermia enhances CTL cross-priming. J Immunol 2006;176:2134–41.
- Takahashi T, Mitsuhashi N, Sakurai H, Niibe H. Modifications of tumor-associated antigen expression on human lung cancer cells by hyperthermia and cytokine. Anticancer Res 1995;15:2601–6.
- Burd R, Dziedzic TS, Xu Y, et al. Tumor cell apoptosis, lymphocyte recruitment and tumor vascular changes are induced by low temperature, long duration (fever-like) whole body hyperthermia. J Cell Physiol 1998;177:137–47.
- Calabrese C, Poppleton H, Kocak M, et al. A perivascular niche for brain tumor stem cells. Cancer Cell 2007;11:69–82.
- Plaks V, Kong N, Werb Z. The cancer stem cell niche: how essential is the niche in regulating stemness of tumor cells? Cell Stem Cell 2015;16:225–38.
- Fessler E, Borovski T, Medema JP. Endothelial cells induce cancer stem cell features in differentiated glioblastoma cells via bFGF. Mol Cancer 2015;14:157.
- Charles N, Ozawa T, Squatrito M, et al. Perivascular nitric oxide activates notch signaling and promotes stem-like character in PDGF-induced glioma cells. Cell Stem Cell 2010;6:141–52.
- Wang J, Wakeman TP, Lathia JD, et al. Notch promotes radioresistance of glioma stem cells. Stem Cells 2010;28:17–28.
- Folkins C, Man S, Xu P, et al. Anticancer therapies combining antiangiogenic and tumor cell cytotoxic effects reduce the tumor stem-like cell fraction in glioma xenograft tumors. Cancer Res 2007;67:3560–4.
- Hovinga KE, Shimizu F, Wang R, et al. Inhibition of notch signaling in glioblastoma targets cancer stem cells via an endothelial cell intermediate. Stem Cells 2010;28:1019–29.
- Ingber DE. Cancer as a disease of epithelial–mesenchymal interactions and extracellular matrix regulation. Different Res Biol Divers 2002;70:547–60.
- Kalamida D, Karagounis IV, Mitrakas A, et al. Fever-range hyperthermia vs. hypothermia effect on cancer cell viability, proliferation and HSP90 expression. PLoS One 2015;10:e0116021.
- Nakabe N, Kokura S, Shimozawa M, et al. Hyperthermia attenuates TNF-alpha-induced up regulation of endothelial cell adhesion molecules in human arterial endothelial cells. Int J Hypertherm 2007;23:217–24.
- Basile A, Biziato D, Sherbet GV, et al. Hyperthermia inhibits cell proliferation and induces apoptosis: relative signaling status of P53, S100A4, and Notch in heat sensitive and resistant cell lines. J Cell Biochem 2008;103:212–20.
- zur Hausen H. Viruses in human cancers. Science 1991;254:1167–73.
- Beasley RP, Hwang LY. Hepatocellular carcinoma and hepatitis B virus. Semin Liver Dis 1984;4:113–21.
- Epstein MA, Achong BG, Barr YM. Virus particles in cultured lymphoblasts from Burkitt's lymphoma. Lancet 1964;1:702–3.
- zur Hausen H, Gissmann L, Steiner W, et al. Human papilloma viruses and cancer. Biblioth Haematol 1975;43:569–71.
- Chang Y, Cesarman E, Pessin MS, et al. Identification of herpesvirus-like DNA sequences in AIDS-associated Kaposi's sarcoma. Science 1994;266:1865–9.
- Liao JB. Viruses and human cancer. Yale J Biol Med 2006;79:115–22.
- Scheffner M, Werness BA, Huibregtse JM, et al. The E6 oncoprotein encoded by human papillomavirus types 16 and 18 promotes the degradation of p53. Cell 1990;63:1129–36.
- Jin L, Xu ZX. Recent advances in the study of HPV-associated carcinogenesis. Virol Sin 2015;30:101–6.
- Wise-Draper TM, Wells SI. Papillomavirus E6 and E7 proteins and their cellular targets. Front Biosci 2008;13:1003–17.
- Tyagi A, Vishnoi K, Mahata S, et al. Cervical cancer stem cells selectively overexpress HPV oncoprotein E6 that controls stemness and self-renewal through upregulation of HES1. Clin Cancer Res 2016;22:4170–84.
- Zhang M, Kumar B, Piao L, et al. Elevated intrinsic cancer stem cell population in human papillomavirus-associated head and neck squamous cell carcinoma. Cancer 2014;120:992–1001.
- Lewis JS Jr, Chernock RD, Ma XJ, et al. Partial p16 staining in oropharyngeal squamous cell carcinoma: extent and pattern correlate with human papillomavirus RNA status. Mod Pathol 2012;25:1212–20.
- Schwartz SM, Daling JR, Doody DR, et al. Oral cancer risk in relation to sexual history and evidence of human papillomavirus infection. J Natl Cancer Inst 1998;90:1626–36.
- Weinberger PM, Yu Z, Haffty BG, et al. Molecular classification identifies a subset of human papillomavirus-associated oropharyngeal cancers with favorable prognosis. J Clin Oncol 2006;24:736–47.
- Repasky EA, Evans SS, Dewhirst MW. Temperature matters! And why it should matter to tumor immunologists. Cancer Immunol Res 2013;1:210–16.
- Diehn M, Cho RW, Lobo NA, et al. Association of reactive oxygen species levels and radioresistance in cancer stem cells. Nature 2009;458:780–3.
- Mathews LA, Cabarcas SM, Farrar WL. DNA repair: the culprit for tumor-initiating cell survival? Cancer Metast Rev 2011;30:185–97.
- Mathews LA, Cabarcas SM, Hurt EM, et al. Increased expression of DNA repair genes in invasive human pancreatic cancer cells. Pancreas 2011;40:730–9.
- Maugeri-Sacca M, Bartucci M, De Maria R. DNA damage repair pathways in cancer stem cells. Mol Cancer Ther 2012;11:1627–36.
- Wang QE. DNA damage responses in cancer stem cells: implications for cancer therapeutic strategies. World J Biol Chem 2015;6:57–64.
- Corry PM, Robinson S, Getz S. Hyperthermic effects on DNA repair mechanisms. Radiology 1977;123:475–82.
- Eppink B, Krawczyk PM, Stap J, Kanaar R. Hyperthermia-induced DNA repair deficiency suggests novel therapeutic anti-cancer strategies. Int J Hypertherm 2012;28:509–17.
- Krawczyk PM, Eppink B, Essers J, et al. Mild hyperthermia inhibits homologous recombination, induces BRCA2 degradation, and sensitizes cancer cells to poly (ADP-ribose) polymerase-1 inhibition. Proc Natl Acad Sci USA 2011;108:9851–6.
- Kampinga HH. Cell biological effects of hyperthermia alone or combined with radiation or drugs: a short introduction to newcomers in the field. Int J Hypertherm 2006;22:191–6.
- Kampinga HH, Dynlacht JR, Dikomey E. Mechanism of radiosensitization by hyperthermia (> or =43 degrees C) as derived from studies with DNA repair defective mutant cell lines. Int J Hypertherm 2004;20:131–9.
- Pelicci PG, Dalton P, Orecchia R. Heating cancer stem cells to reduce tumor relapse. Breast Cancer Res: BCR 2011;13:305.
- van Oorschot B, Granata G, Franco Ten Cate DS, et al. Targeting DNA double strand break repair with hyperthermia and DNA-PKcs inhibition to enhance the effect of radiation treatment. Oncotarget 2016;7:65504–13.
- Man J, Shoemake JD, Ma T, et al. Hyperthermia sensitizes glioma stem-like cells to radiation by inhibiting AKT signaling. Cancer Res 2015;75:1760–9.
- Oei AL, Vriend LE, Crezee J, et al. Effects of hyperthermia on DNA repair pathways: one treatment to inhibit them all. Radiat Oncol 2015;10:165.
- Takahashi A, Mori E, Nakagawa Y, et al. Homologous recombination preferentially repairs heat-induced DNA double-strand breaks in mammalian cells. Int J Hypertherm 2016:1–7. [Epub ahead of print].
- Horsman MR, Mortensen LS, Petersen JB, et al. Imaging hypoxia to improve radiotherapy outcome. Nat Rev Clin Oncol 2012;9:674–87.