Abstract
The usability of amino acid–functionalised nitrogen-doped graphene oxide (GO) structures (AA-NGO) in drug delivery (DD) systems was studied using the computational approaches. Docking, molecular dynamic (MD) and molecular mechanics generalised born surface area studies were carried out. The calculations were done at 37 and 42 °C to study the usability of the mentioned structures in thermally induced DD systems. In this method, the drug is released by increasing the temperature. For the representative cases, studies at 32, 37, 42 and 47 °C were carried out, as well. Both one-to-one and two-to-one ratio between the AA-NGO and Taxol were studied. In the one-to-one system the Lys–NGO is the best structure to be used in the thermo-sensitive DD systems, based on the binding energy differences calculated at mentioned four temperatures. In the two-to-one systems, the Glu–NGO is leading to the most promising results. In this case, the longer distance between the two layers at higher temperature was regarded as the reason for the better release of the drug at a higher temperature. In this case, at a lower temperature, the Taxol is trapped between two layers of Glu–NGO and has interactions with both of them. At higher temperature, the interaction with just one of the layers is observable, which means lesser interactions and better release of the Taxol.
Introduction
Cancer is one of the main reasons for the mortality of humankind. Since there is no effective treatment for cancer currently; therefore, there is a high demand to introduce new pharmaceutics to treat it or modify the usage methods of currently available drugs to make them more effective with lesser damage to the normal cells in the body. In this content, using drug delivery (DD) systems, especially nano-based systems are of interest [Citation1]. Now a day, nano-technology is growing rapidly and it widely used in different areas including the cancer treatment via different ways, including the introduction of new DD systems [Citation2,Citation3]. Among the numerous introduced DD systems, the graphene (G) based systems has attracted a great consideration [Citation4,Citation5]. The G, as the two-dimensional allotrope of carbon, has different interesting properties which, makes it a suitable candidate to be used in the DD systems. For example, it has very high surface area that enables it to load high amount of drugs on it [Citation6]. Also, the lipophilic properties of the surface of the G make it a very good choice to be used for the delivery of the hydrophobic drugs. Because, most of the drugs used for the treatment of cancer are lipophilic in nature, so it is a great advantage to use G and related compounds [Citation7,Citation8]. It was mentioned that the lipophilic properties of the G and the related compound are suitable to load the hydrophobic compounds. But the hydrophobicity is creating problems for the bioavailability of G itself. Therefore, the treatment of the surface of the G to make it more hydrophilic, has attracted the consideration of the research teams as well [Citation7]. In addition, to make DD systems more effective, the cancer cells targeting compounds such as folic acid and transferrins were used to functionalise the G to make the targeted-DD systems [Citation9,Citation10].
From another point of view, insufficient biocompatibility of the G and graphene oxide (GO) is creating difficulties in the usability of the mentioned systems. To solve the problem, the functionalised G and GO were used extensively to develop the DD systems [Citation11]. For example, the carboxymethyl chitosan functionalised GO was used as the carrier for the anticancer drug doxorubicin hydrochloride (DOX). Based on the cell uptake behaviours, the mentioned DD system was targeting only the cancer cells [Citation12]. Although the DOX has enough solubility in the aqueous media but its effect on the normal cells is limiting its usability. To solve the problem, different cancer cell targeting DD systems developed for the mentioned drug. GO modified by poly(ethylene glycol) methyl ether methacrylate (PEGMA) was used to deliver the DOX to the cancer cells, for example [Citation13]. The studied system was showed 84.8% load of the DOX and the release was sensitive to the pH. At the pH 5.5, the highest amount of release was determined. A similar system, containing GO functionalised with lactobionic acid and carboxymethyl chitosan was used for the targeted DD of DOX as well. The studied system was pH sensitive [Citation14]. Using chitosan/dextran modified GO for the release of the DOX was reported, too. It was observed that the mentioned system was up-taken by MCF-7 cells and had a robust cytotoxicity to the cancer cells [Citation15].
In other study, pegylated folate modified GO was used for the targeted DD of anticancer drugs. The mentioned system can deliver different hydrophobic and aromatic ring containing drugs. The results of this study showed that the targeting of cancer cells, which are containing the folic acid receptor, was very high due to the presence of the folic acid in the system [Citation16].
The delivery of the vancomycin hydrochloride was done using the polyvinyl pyrrolidone blended with the GO. The mentioned structure was the core section of a core/sheath system. The poly(ɛ-caprolactone) was used to create the sheath [Citation17]. In a similar study, chemotherapy and photothermal therapy using the sorafenib-loaded functionalised GO as a receptor-targeted approach was reported. In this study, the folic acid-conjugated polyvinyl pyrrolidone functionalised GOs was studied [Citation18].
A co-delivery system based on the modified GO was developed using membrane-related proteins and intracellular-functioning small-molecule drugs joined in one system. The site specified release of the compounds were done to their distinct sites of action individually [Citation19,Citation20].
Multi-functionalised GO was developed to target the prostate cancer and the magnetic resonance imaging at the same time. The gadolinium diethylene triamine pentaacetate and DOX were loaded on the mentioned systems; they were playing the role of the imaging and cancer therapy, respectively [Citation21]. The carrying of DOX using GO/dendrimer hybrid was reported, as well, in which the maximum amount of release was observed at the pH 5.5. The role of the dendrimer is making stable suspension of the drug carrier in the aqueous media [Citation22].
Since taxol and docetaxel are important drugs to treat cancer; therefore different DD systems were developed for them. As representative examples, delivery of taxol was achieved using PEGylated graphene oxide. The prepared carrier showed excellent water solubility that caused prolonged blood circulation time that is increasing the efficiency of DD [Citation23]. Delivery of docetaxel by targeted manner was achieved using the transferrin/poly (allylamine hydrochloride)-functionalised graphene oxide. The targeting ability was achieved because of the presence of the transferrin section of the system that can detect the extracellular transferrin receptors [Citation24].
The photodynamic therapy of the tumours using the GO-based systems was reported as well. In a study, the monoclonal antibody was attached to the GO to target the tumours. The pyropheophorbide conjugated with polyethylene-glycol was used to induce phototoxicity. The polyethylene-glycol was used to improve the water solubility of the system [Citation25].
In addition to the experimental studies on the usability of G- and GO-based systems in the DD and targeted-DD, different computational investigations were done to obtained more details about the mechanistic aspects of the phenomena as much as possible. Such a data are valuable in designing new systems with higher efficiency. Herein representative examples are provided [Citation26–30].
Protein energy landscape exploration (PELE) method was used to predict the binding modes of the ligands with proteins. This method was able to detect the side chain movement and small to medium backbone movements; although this method has difficulty to detect large secondary structure rearrangements [Citation31].
A molecular dynamic (MD) study was carried out on the linear polyethylene glycol-block-dendritic oligo-cholic acid (telodendrimer) to evaluate and improve hemo-compatibility of mentioned nano-carriers to be used in the anticancer DD systems. The conformation of the subunits were studied. These subunits had different glycerol substitution. In addition, membrane activities of nano-carriers were examined computationally by analysing the binding energies and the polar surface areas of the hairpin conformations of the system. The computational results were examined and confirmed by experimental approach. The in vivo studies indicated that the designed targeted-DD system is more efficient than free drug administration to deliver the studied drugs (taxol and DOX) to the tumour sites [Citation32].
To obtain an improved drug-specific nano-carrier, computational design and customising of linear dendritic copolymers (telodendrimers) were examined, as well. Identifying the optimal building block was done by examining a library of small molecules. The efficiency of nano-carriers were examined computationally by analysing the binding energies of the carriers to the target compounds. Also, the usability and the efficiency of the designed systems were examined experimentally for the delivery of the DOX. Rhein-containing nano-carriers showed the best results from different point of views such as prolonged circulation, effective tumour targeting, increased tolerated dose, sustained drug release, reduced toxicity and superior anticancer effects [Citation33].
Due to importance in the use of nano-cage-based metal-organic frameworks (MOF) in the DD systems, mixed computational/experimental studies were done on the MOFs. The MOF systems were applied to absorb and release 5-fluorouracil. Gran Canonical Monte Carlo (GCMC) simulation approaches were used to analyse the detailed absorption behaviour of the 5-fluorouracil on the studied MOF systems. This method explicitly elucidates density fluctuations at fixed temperature and volume via trial insertion and deletion of molecules that makes it the preferred choice for the studying of interfacial phenomena. In that study, the simulation process was able to reproduce the experimental results [Citation34].
The MD simulation studies on the structure and drug loading properties of PEGylated poly (amidoamine) with different percentage of PEG were done to evaluate the usability of the system to deliver 5-fluorouracil. The results showed that the 25% of the PEG is the optimum amount to deliver the mentioned drug [Citation35].
Since pH of the tumour cells is lower than regular cells, therefore, it was used to design pH-responsive molecular tweezers using a computational approach. The binding energies were examined by steered MD method. The results showed that change in the pH is crucial to release the drug [Citation36] because a change in the pH and consequently, the change in the conformation is the reason to release the drug.
Behaviour of nano-particulate lipid and polymeric systems as the DD systems using their modelling by computational approaches were studied. At first, the modelling of the system was done by MD method. At the next step, the binding energies of the selected drugs with the modelled system was analysed. The experimental studies were done to evaluate the computational results. The comparison has confirmed the accuracy of the computational method [Citation37].
Age-related molecular degeneration is the leading cause of vision loss. This can be treated using the anti-vascular epithelial growth factor (anti-VEGF) drugs. A computational study on the thermally responsive-poly(N-isopolyacrylamide) gel was done to evaluate its usability to deliver anti-VEGF. The simulation showed that the drug therapeutic levels in the posterior eye are sustained for 8 weeks. This finding confirmed the usability of this DD system instead of the intravitreal injection method [Citation38].
The stimulated release of the drugs is of interest, as well; because in this method, the release of the drug is carrying out only to the target positions without losing of the drug during the transferring in the bloodstream. Because of mentioned advantage, the design of the pH-responsive biomaterials to be used in targeted-DD was studied. MD method was used to study the mentioned systems. PEG and PEG-tethered oligopeptide were used. The PEG-tethered oligopeptide is undergoing conformational change because of a change in the pH which is leading to drug release [Citation39].
Ligand functionalised nano-carriers can be selectively bind to the diseased cells, therefore the modified nano-carriers can be used in the targeted-DD system. Such a behaviour was studied using the Monte Carlo simulation process to determine the selectivity of binding of nano-carrier to the cell surface by targeting the membrane proteins. The results showed that multiple-targeting agents with weaker affinities are acting better in targeting the diseased cells [Citation40].
The usability of chitin as nano-carrier for the cancer DD was studied using computational and experimental approaches. Three drugs, including curcumin, docetaxel and 5-flurouracil were used in this study. The drug-loaded chitin structures were examined against gastric adenocarcinoma cells, which the results showed an enhanced drug uptake and cancer cell death. Excellent relation between the calculated binding energies using docking approach and the drug loadings on the carrier was observed in this study [Citation41].
Using mathematical and computational approaches to study and analyse the hyperthermia approach to heal the different cancers are of interest as well. For example, the treatment planning using the laser in the thermal therapy was evaluated by model evaluation method. Based on the cross-validation analysis, the computer models can be used successfully for planning of magnetic resonance-guided laser-induced thermal therapy [Citation42]. In another study, a computational method was used to model an pulsing model based on the impedance-control [Citation43]. The results of this study showed that the model can successfully simulate the coagulation zone size and electrical-thermal performance. The ablation of radiofrequency by controlling of the temperature was modelled using computer model, too [Citation44]. In this study, the healthy tissue was modelled in 3 D way using a 12 cm cubical model. A 2.5 cm tumour has been modelled inside of it. The breast, lung, liver and kidney tissues were modelled in this study. The model showed that the tissues have an important role on the ablation of radiofrequency. A similar study was used to compare the results of the experimental pattern of ablation of the microwave heating by results obtained by computer modelling [Citation45]. They suggested that the simplified computer modelling can be used to predict the temperature of the tissues.
The mentioned examples of the usage of GO-based systems are showing their importance in the development of DD systems. The nitrogen doped GO (NGO) systems, as relatively new variants of GO system, have attracted the consideration of different research groups [Citation46–49]. There is ongoing interest to use the NGO systems in different areas and DD related application is not out of mind. To the best of our knowledge, there is no computational study on the NGO-based systems in the DD applications. Therefore, in the present study, a computational study was done on the NGO and amino acid functionalised NGO (AA-NGO) systems to examine their usability in the DD applications. The well-known anticancer drug, taxol (TXL), was used as a model in this study.
Computational methods
The structure of the nitrogen doped graphene oxide (NG), amino acids (AA) and taxol (TXL) were optimised using the Gaussian 09 (Rev. D0.01) programme [Citation50]. B3LYP/6-31 g* level of theory was used to optimise all of the mentioned structures [Citation51–53]. Docking studies were done using AutoDock Vina [Citation54]. AutoDockTools was used as a graphical interface for docking step [Citation55]. Grid box with 1 Å spacing was used. The 60 × 60 × 40 grid box was used in X, Y and Z directions, respectively. The iterated local search global optimiser of the AutoDock Vina was used in docking step. MD simulation studies were done using the Amber 12 and AmberTools 12 programmes [Citation56]. The ff99SB and general Amber force field (GAFF) were used to prepare the required files for the MD simulation process [Citation57]. The system was solvated using the TIP3P water model [Citation58]. The systems were placed in a solvent box, 10 Å away from each side of solute. To remove close contacts, a minimisation was done on the initial structures. Two thousand steps were carried out in this step. One thousand steps using the steepest descent algorithm and 1000 steps using the conjugate gradient algorithm. The MD simulations were carried out at different temperatures. In each case, the gradual increasing of temperature during first 25 000 steps was done. Then, the temperature was kept constant at the required temperature for 75 000 steps to ensure the stability of temperature [Citation59]. The Langevin thermostat was used in this step. At the next step, two successive runs, including 100 000 steps for each one were carried out to obtain the constant density and equilibrate the system. SHAKE algorithm was applied to the system and 2 fs time steps were used for MD [Citation60]. At the end, 20 ns MD simulation was done on all of the investigated systems. The molecular mechanics generalised born surface area (MM/GBSA) calculations were done to calculate binding free energies between the TXL/NGO, TXL/AA-NGO and AA-NGO/TXL/AA-NGO systems. The VMD programme was used as the graphical interface for MD simulation process [Citation61].
Results and discussion
Optimizing the structures
All of 20 AA structures were used in this study. The initial structures were optimised with B3LYP/6-31 g* level of theory. The structure of TXL was optimised at the same level of theory. The NGO system was created by manually altering the carbon atoms of G with appropriate nitrogen atoms and changing the selected hydrogen atoms to oxygen, hydroxyl and carboxylic acid moieties to obtain the NGO structure. The final optimised structure for the NGO, at B3LYP/6-31 g* level of theory, is presented in . Previously, it was confirmed that nitrogen atoms can occupy three different positions named as graphitic, pyridinic and pyrrolic positions [Citation62]. Therefore, in the present study, all of the mentioned types of nitrogen atoms were considered.
Figure 1. (a) The optimised structure of NGO at B3LYP/6-31 g*, containing epoxy, hydroxyl and carboxylic acid functional groups; (b) the position of AA on the NGO (Gly–NGO is showed as the representative example) and (c) The graphitic, pyridinic and pyrrolic nitrogen atoms.
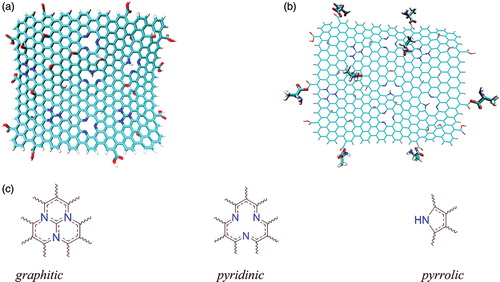
The usual method for the substituting the GO system is converting the OH of the hydroxyl and carboxylic acid moieties to the aryl halide and acyl halide, respectively, and then carrying out of suitable substitution reaction to change the halide by desired functional groups. In the present study, to create AA-NGO systems, totally eight OH functional groups (including two hydroxyl and six carboxylic acid moieties) were replaced with the AA. In all of the cases, the substitution was done in similar positions. Six of AA were added to the edges of the NGO and two of them were placed in the central section of the NGO (). All in all, 20 AA-NGO systems and NGO, itself, were studied.
Docking studies
Because the binding site of the TXL to the NGO and AA-NGO was not clear, at first, docking studies were done to find best binding modes. The binding energies calculated by docking approach are presented in . It is evident that the binding energies are between the −11.3 and −12.1 kcal/mol. The docking method is not able to differentiate the effect of altering of the AA moieties. The binding energy related to the TXL/NGO system is provided as well. In this case, the binding energy is equal to −11.3 kcal/mol. Based on docking binding energies, substituting OH functional groups with AA is not changing the affinity of TXL to it.
Figure 2. Binding energies between the TXL and AA-NGO systems obtained by docking studies. The binding energy related to the NGO is provided for the sake of comparison. The His and Trp grafted NGOs are showing the most affinity toward the TXL based on the calculated binding energies.
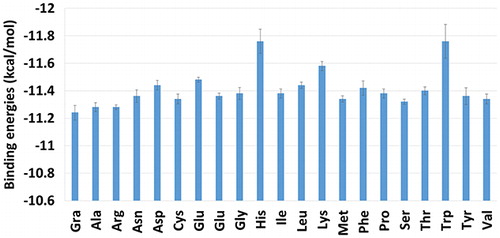
In continue, to further analyse the system, MD simulation studies were carried out.
MD simulation studies
TXL/AA-NGO systems
Based on the binding energy criteria, the best structure in docking step in each case was selected as the starting point of the MD simulation process. After finishing the MD simulations, MM/GBSA approach was used to calculate the binding energy between the TXL and (AA-)NGO systems. The hyperthermia, as a relatively well-known approach in the treatment of cancer, is known as thermal therapy or thermotherapy which in this method, it is usual to increase the temperature of the body to 40–43 °C because the high temperature can damage and kill the cancer cells. Also, it is possible to increase the temperature of the selected region in the body to just affect the desired tissue [Citation63,Citation64]. From another point of view, induced DD systems have attracted great considerations. In this method, the release of the drug is occurring in the neighbourhood of the cancer cell. In this approach, the healthy cells will encounter lesser damage and from another point of view, the lesser amount of the drug is needed. Different factors were used to act as a trigger to release the drugs. The most well-known inducers are changing the pH or temperature [Citation65–69].
Considering these statements, this question is arising, is it possible to use the AA-NGO systems as an induced DD system? Herein, the usability of temperature, as a trigger, to release the TXL was examined using the computational methods. To examine the usability of AA-NGO systems, MD simulation calculations were carried out at two different temperatures: 37 °C (as the normal body temperature) and 42 °C (temperature which is usually used in the hyperthermia approach). It was assumed that mixing the hyperthermia with induced release of TXL will be more effective than each method separately.
To evaluate the mentioned statement, and to examine the usability of the AA-NGO in the thermally induced DD systems, the binding energies were calculated using the MM/GBSA approach. The results are presented in . In , the binding energy differences (E42 – E37) are presented. Based on the results provided in , it could be seen that the binding energies in 37 and 42 °C are very close to each other except for tryptophan (Trp) functionalised NGO system. In this case, the binding energy difference between the 32 and 42 °C is 12.2 kcal/mol. In another word, binding of the TXL to the Trp-NGO in the 42 °C is looser than the 37 °C and TXL prefers to leave the surface of the NGO in higher temperature. This finding is according to our goal to release the drug in higher temperature. The Gly, Ile and Lys grafted NGO systems are showing energy differences equal to 3.4, 2.8 and 3.4 kcal/mol, respectively.
Figure 3. (a) Binding free energy between the TXL/(AA-)NGO systems calculated by MM/GBSA approach; (b) Binding energy differences between 42 and 37 °C (E42 – E37). Based on the results Trp is showing most affinity to the TXL at lower temperature in comparison with higher one. The Gly, Ile and Lys are acting in a similar way. They show more affinity to the TXL at lower temperature.
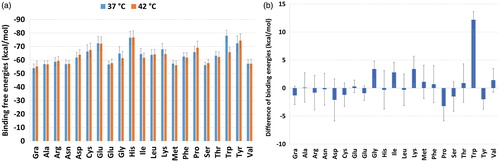
To figure out the reason for the mentioned observations, the distance and hydrogen bonding between the TXL and Trp-NGO system were analysed. The results for 37 and 42 °C are presented in Figure S1 (Supporting Information). Based on the binding energies, the TXL is showing more affinity toward the Trp-NGO at a lower temperature than higher one. Such a behaviour can be analysed using the presented hydrogen bonding interactions and the distance between the TXL and the Trp-NGO. It is evident that at a lower temperature the number of hydrogen bonding interactions is more than a higher temperature. From another point of view, the distance between the TXL and Trp-NGO at a lower temperature is shorter than the distance at a higher temperature, especially after mid of MD simulation. The higher number of hydrogen bonding at a lower temperature is consistent with lesser movement of the TXL and Trp-NGO that makes it possible to contribute to hydrogen bonding interaction. From another point of view, the shorter distance of the TXL and Trp-NGO is positioning these two species in a better situation to create hydrogen bond interactions and other kinds of interactions such as van der Waals ones. It can be seen that at a higher temperature, the TXL and Trp-NGO prefer to be away about 13.5 Å during the MD simulation process. At lower temperature, although their distance is about 14 Å at the beginning of the simulation process, but they come close to each other (12 Å), especially after the mid of simulation process. Another important point which it is worthy to mention is the number of hydrogen bonding interactions between the TXL and Trp-NGO. From the data presented in Figure S1, it is clear that a higher number of hydrogen bonding can be observed at a lower temperature while at the higher temperature the opposite situation is observed.
AA-NGO/TXL/AA-NGO sandwich-like systems
In the previous section, the one-to-one ratio of TXL and AA-NGO was used. To continue, it was assumed that the ratio of AA-NGO to TXL is two-to-one. In this case, sandwich-like structures could be obtained. To create such a system, in the previous TXL/AA-NGO system, an additional AA-NGO was added in 15 Å away from the original AA-NGO layer. In these structures the TXL was positioned between two mentioned layers (). The distance between two layers was selected long enough to give the TXL chance to change its conformation and position as much as needed to adapt itself to the new situation.
Figure 4. Sandwich-like AA–NGO/TXL/AA–NGO system. The AA–NGOs were positioned 15 Å away from each other (red = TXL, green = water molecules). (The colored image is available in online version.)
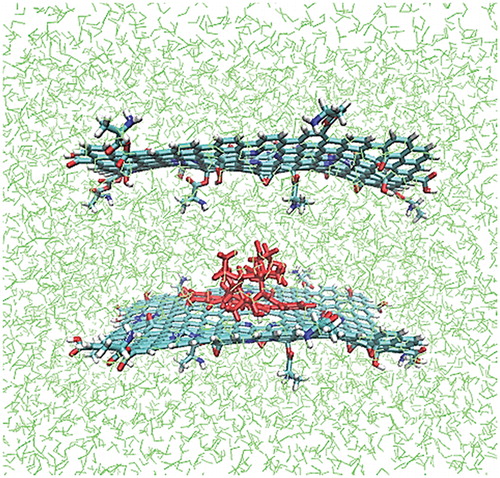
The MD simulation studies were done as before and finally, MM/GBSA approach was used to calculate the binding energies. The binding energies related to sandwich-like systems at 37 and 42 °C and binding energy differences (E42–E37) are presented in , respectively. The result related to the NGO/TXL/NGO system, without any modification of the NGO structure is provided as well. The result of this structure is not acceptable, similar to the monolayer one. In the case of NGO, based on the binding energy difference, at a lower temperature, the TXL prefers to leave the NGOs, while at a higher temperature it prefers to bind to the NGOs. Between the studied sandwich-like systems, Trp-NGO is acting like the one-layer system and E42–E37 is calculated to be 10.2 kcal/mol. By altering the temperature from 37 to 42 °C, glycine (Gly), threonine (Thr), arginine (Arg) and glutamine (Gln) are showing 24.2, 13.6, 9.4 and 7.7 kcal/mol binding free energy change among the studied structures. The mentioned systems, especially Gly–NGO system, are good candidates to be used in temperature induced DD system.
Figure 5. (a) Binding energies calculated for the sandwich-like systems at two studied temperatures (37 and 42 °C); (b) Binding energy differences = E42 – E37. The Asp–, Gly–, Thr– and Tyr–NGO are showing more affinity toward the TXL in lower temperature in comparison with hither temperature.
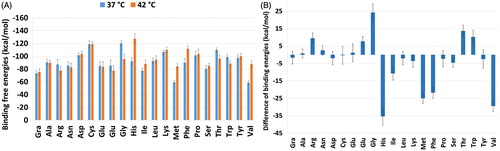
To study the origin of the observed phenomena, the hydrogen bonding interaction between the TXL and Gly–NGO and the distance between two Gly–NGO sheets was studied. The obtained results are shown in . The data for both 37 and 42 °C were provided for the sake of comparison. It is seen that at 37 °C there is more hydrogen bonding interaction between Gly–NGOs and TXL. The data related to the distance between Gly–NGO systems is consistent with the hydrogen bonding data. As it can be seen in , the distance between the two layers is longer at 42 °C relative to 37 °C. The longer distance between the two layers is decreasing the chance to create hydrogen bonding interactions between the TXL and the Gly–NGOs.
Figure 6. (a) Hydrogen bonding between the TXL and Gly–NGOs during MD simulation process. More hydrogen bonding interaction is lower temperature (I) are observable. (b) Representative hydrogen bonding interaction between the TXL and Gly–NGOs. Both OH moiety of the NGO and O of the Gly have contribution in the hydrogen bonding interaction with the TXL. (c) Distance between centre of mass of the two Gly–NGO sheets during MD simulation process. I and II are implying to 37 and 42 °C, respectively. At lower temperature the sheets have shorter distance. At higher temperature the sheets prefer to separate from each other.
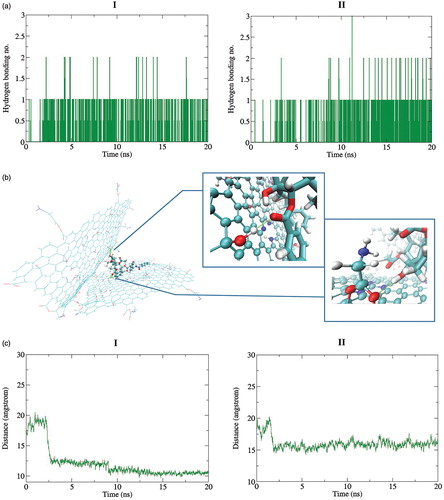
At lower temperature, the distance between two layers is remaining relatively constant about 10 Å, while at higher temperature the distance is about 15 Å, during MD simulation process.
The position of two Gly–NGO sheets during the MD simulation process is provided in . From , it can be seen that two Gly–NGO layers at higher temperature prefer to keep away from each other. Although the two layers did not separate completely, but their positions were changed very much during the MD process. At lower temperature, the lesser tendency was observed to separate two layers from each other. These findings, including the lesser number of hydrogen bonding interactions, longer distance between the layers and separation of two layers at higher temperature are all consistent with the better release of TXL at a higher temperature in comparison with the lower one.
Figure 7. The change in the position of two Gly–NGO sheets during MD simulation process at 37 (I) and 42 °C (II), respectively. Snapshots for selected trajectories are presented. At higher temperature the sheets prefer to go away from each other that make it easy to release the TXL.
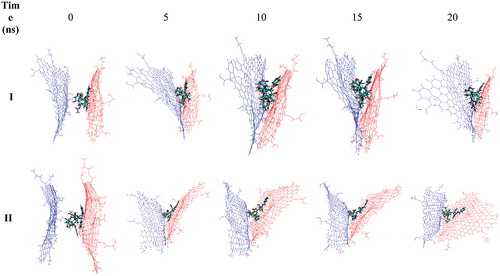
His-NGO was showed the worst results. Based on the binding energies, in the case of His-NGO, the TXL and His-NGO are showing more affinity at a higher temperature than the lower one. The result related to the distance between two His-NGO layers is presented in Figure S2. Although at higher temperature the distance between the layers was increased to some extent, but this change is not as much as change related to the Gly–NGO case. Based on these results the lower affinity at 37 °C in comparison with 42 °C is not explainable.
To shed light on the origin of the observed phenomena, the structural changes during the MD simulation process was examined. The snapshots related to the selected MD simulation trajectories are presented in . The large structural change, which was observed in the case of Gly–NGO system, is not observed here. However, structural change is the opposite of the Gly–NGO. In Gly–NGO system, two layers have a tendency to get separate from each other at higher temperature, while in the case of His-NGO two layers are showing more affinity toward each other and prefer to adhere to each other; this closeness is pushing the TXL away from the structure, as it can be seen in the side view of the final structure of MD simulation. This behaviour is forcing the TXL to leave the system, which is the reason for the expulsion of TXL in a lower temperature in comparison with a higher one. At higher temperature the Gly–NGO structures prefer to have a longer distance from each other (Figure S2II) which the longer distance provides enough space for TXL to remain inside the sandwich-like structure. At a lower temperature, TXL diffused out from the system due to the shorter distance of the two layers which there is no enough space for the TXL.
Figure 8. Snapshots related to selected trajectories of the sandwich-like Gly–NGO/TXL/Gly–NGO at 37 (I) and 42 °C (II) temperatures. At lower temperature (I) two Gly–NGO sheets are close to each other which is forcing the TXL to leave the system. At higher temperature (II) two mentioned sheets have enough distance from each other to keep the TXL inside the system and prevent it from leaving the system.
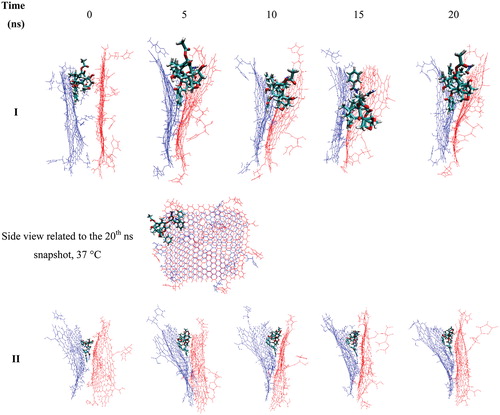
Based on the results presented in which indicated the binding energies calculated at 37 and 42 °C, the Val–NGO/TXL/Val–NGO system has the next worst situation after Gly–NGO. In this case as well as the Gly–NGO system, better release at a lower temperature is suggested in comparison with higher temperature. To make it brief, only the final structures related to the MD simulation process are presented in Figure S3. In this case, relatively different reason can be made based on the final step snapshot of the MD simulations. It can be seen that at a lower temperature, two layers are completely separated from each other and the TXL is making contact with only one layer. At higher temperature, although there is a tendency to push away the TXL from the system, but until 20 ns, it did not happen completely and the TXL has interactions with both layers. The interaction with two layers is making it more difficult for the TXL to leave the sandwich-like system in comparison with the other system, which is acting like the monolayer one.
Based on the calculated binding free energies at the 37 and 42 °C, in one-layer systems the Trp, Gly, Ile and Lys grafted NGO systems are showing to be useful in the temperature induced release of the TXL. In the sandwich-like systems, the Arg, Glu, Gly, Thr and Trp were showed to be more potent as temperature-responsive DD systems. To further investigate the mentioned statements, two various temperatures were used to evaluate the usability of the one-layer and sandwich-like systems in the release of TXL in temperature-responsive manner. Therefore, MD simulations and binding energy calculations for the mentioned systems were carried out in 32 and 47 °C. The obtained results are presented in the Figure S4.
The results of these studies are consistent with the previous ones except for Ile and Trp in the one layer systems. These two systems are acting in a fluctuating manner and no direct relation between the temperature and binding free energy can be seen. In other structures the new results are confirming the usability of the mentioned one- and two-layer systems in the thermally induced release of the TXL. The results are showing that in two layered systems, the Glu is showing the highest thermo-responsivity based on the slope of the line representing the change in the binding energies by changing the temperature (fitted line slope = 3.1); the Trp is positioned in the next level based on the slope of the line (fitted line slope = 2.6). The more value of the line slopes is showing the higher sensitivity of the binding energies to the change of the temperature. In the one-layer ones, the Lys is showing the line slope equal to 0.25. The line slope in the one layer systems are much lesser than the sandwich-like ones. Based on these results it can be concluded that the sandwich-like systems are more potent to be used in the temperature-responsive DD system to release the TXL.
From the results previous sections, it seems that it will be very difficult to create a global governing role to predict the affinity of AA–NGO layers to each other and/or TXL. From another point of view, due to adding AA, the change in the solubility of the NGO is making the situation more difficult to be analysed. For example, the Phe, Tyr and Trp (Figure S5) have a relatively similar structures. It was presumed, at first, that their results should be very similar to each other, but the obtained results were not consistent with each other. For example, in one layer system, the Trp is showing the most promising results while the Phe is acting below the average and the Tyr does not lead to acceptable results at all. In sandwich-like systems, the Trp is acting in an acceptable manner, while the Phe is giving one of the worst results. The Tyr is acting in a bad way and the results are not acceptable. It is suggested that in each case, for any special usage, the new calculation being carried out.
The mentioned inconsistencies are due to different aspects which are acting in reverse directions. For example, adding a benzene ring can increase the possibility of new π–π bonding and van der Waals interactions with the TXL and increases adherence to AA–NGO; such a case is observable by adding the Phe AA that has a Ph ring, as a side chain. In this case, the affinity of TXL to the Phe–NGO is increased and higher binding energy is calculated, especially at a higher temperature (). While adding the Tyr that has 4-hydroxy-Ph side chain is acting in a different way. In this case, it seems that adding the OH moiety that can increase the solubility of functionalised NGO and add the aromatic benzene ring at the same time is causing difficulties about the prediction of the behaviour of the Tyr–NGO. The OH is increasing the solubility while the benzene ring is decreasing it. In the case of Trp, adding an indole ring is causing completely different results. The indole ring has both the aromatic ring and hydrogen bonding donor/acceptor functional group (NH) at the same time, similar to 4-hydroxy-Ph of Tyr, but it is acting in a different way. It can be concluded that both the identity and size of the functional groups are important and must be considered.
The AAs consist of alkyl side chain (Ala, Leu, Ile, Met and Val) are acting in relatively similar way (but not exact). In most of the mentioned cases, the release of the TXL is difficult even at a higher temperature because alkyl side chain was caused to an increase in the affinity of the hydrophobe TXL to the AA–NGO.
Most of the AAs consist of a polar side chain with hydrogen bond making capabilities, such as Arg, Asn, Asp, Gln, Glu and Thr, are acting in such a way. The mentioned AAs can keep the TXL at a lower temperature and release it at a higher temperature. In this case, it seems that at lower temperatures the interactions between the TXL and AA–NGO is the governing criteria while at higher temperature the hydrogen bonding interactions are playing a more important role, which lead to the increased solubility of AA-NGOs and consequently, the release of the TXL.
Conclusions
In conclusion, detailed computational studies, including docking, MD simulations and MM/GBSA studies were carried out on the NGO and AA functionalised variants of the mentioned structures (AA–NGO). Taxol (TXL) was used as the model drug. Calculations were done at two different temperatures, 37 and 42 °C, to investigate the usability of the mentioned systems in the thermally induced DD systems. In selected cases calculations at 32 and 47 °C were done as well. Calculations were done in one-to-one ratio of (AA-)NGO to TXL and in the two-to-one ratio to create sandwich-like configurations. The results showed that at one-to-one ratio the Lys-NGO is the best structure to be used in the thermally induced DD systems. In the sandwich-like structures, the Glu–NGO is showing the most promising results to be used in thermally induced DD systems. In this case, the separation of two layers of Glu–NGO at a higher temperature that is facilitating the release of the TXL can be regarded as the main reason for the observed behaviour. By considering the all of the results related to the sandwich-like structures, it can be suggested that there is a relative, but not exact, relation between the structures of the AA used for the functionalising the NGO and its usability in thermally induced DD systems. The AA with a polar side chain that they have functional groups with the hydrogen bond making capability is making the system be used in thermally induced DD systems.
Supplementary File
Download PDF (851.6 KB)Acknowledgements
The financial support from the Research Council of the University of Tabriz is gratefully acknowledged. The computational facilities provided by High-Performance Computing (HPC) section of IT centre at the University of Tabriz and Sheikh Bahaei National High-Performance Computing Centre (NHPCC) at Isfahan University of Technology are acknowledged.
Additional information
Funding
References
- Siegel RL, Miller KD, Jemal A. (2015). Cancer statistics, 2015. CA Cancer J Clin 65:5–29.
- Luo Z, Ding X, Hu Y, et al. (2013). Engineering a hollow nanocontainer platform with multifunctional molecular machines for tumor-targeted therapy in vitro and in vivo. ACS Nano 7:10271–84.
- Zhao F, Zhao Y, Liu Y, et al. (2011). Cellular uptake, intracellular trafficking, and cytotoxicity of nanomaterials. Small 7:1322–37.
- Gurunathan S, Kim J-H. (2016). Synthesis, toxicity, biocompatibility, and biomedical applications of graphene and graphene-related materials. Int J Nanomedicine 11:1927–45.
- Yang K, Feng L, Liu Z. (2016). Stimuli responsive drug delivery systems based on nano-graphene for cancer therapy. Adv Drug Deliv Rev 105:228–41.
- Nergiz SZ, Gandra N, Tadepalli S, Singamaneni S. (2014). Multifunctional hybrid nanopatches of graphene oxide and gold nanostars for ultraefficient photothermal cancer therapy. ACS Appl Mater Interf 6:16395–402.
- Zhang B, Wang Y, Zhai G. (2016). Biomedical applications of the graphene-based materials. Mater Sci Eng C Mater Biol Appl C 61:953–64.
- Thomas RG, Moon M, Lee S, Jeong YY. (2015). Paclitaxel loaded hyaluronic acid nanoparticles for targeted cancer therapy: in vitro and in vivo analysis. Int J Biol Macromol 72:510–18.
- Shim G, Kim M-G, Park JY, Oh Y-K. (2016). Graphene-based nanosheets for delivery of chemotherapeutics and biological drugs. Adv Drug Deliv Rev 105:205–27.
- Kim M-G, Shon Y, Miao W, et al. (2016). Biodegradable graphene oxide and polyaptamer DNA hybrid hydrogels for implantable drug delivery. Carbon N Y 105:14–22.
- Kiew SF, Kiew LV, Lee HB, et al. (2016). Assessing biocompatibility of graphene oxide-based nanocarriers: A review. J Control Release 226:217–28.
- Yang H, Bremner DH, Tao L, et al. (2016). Carboxymethyl chitosan-mediated synthesis of hyaluronic acid-targeted graphene oxide for cancer drug delivery. Carbohydr Polym 135:72–8.
- Gao P, Liu M, Tian J, et al. (2016). Improving the drug delivery characteristics of graphene oxide based polymer nanocomposites through the “one-pot” synthetic approach of single-electron-transfer living radical polymerization. Appl Surf Sci 378:22–9.
- Pan Q, Lv Y, Williams GR, et al. (2016). Lactobionic acid and carboxymethyl chitosan functionalized graphene oxide nanocomposites as targeted anticancer drug delivery systems. Carbohydr Polym 151:812–20.
- Xie M, Lei H, Zhang Y, et al. (2016). Non-covalent modification of graphene oxide nanocomposites with chitosan/dextran and its application in drug delivery. RSC Adv 6:9328–37.
- Tian J, Luo Y, Huang L, et al. (2016). Pegylated folate and peptide-decorated graphene oxide nanovehicle for in vivo targeted delivery of anticancer drugs and therapeutic self-monitoring. Biosens Bioelectron 80:519–24.
- Yu H, Yang P, Jia Y, et al. (2016). Regulation of biphasic drug release behavior by graphene oxide in polyvinyl pyrrolidone/poly(ɛ-caprolactone) core/sheath nanofiber mats. Coll Surf B Biointerf 146:63–9.
- Kim JO, Thapa RK, Choi JY, et al. (2016). Receptor-targeted, drug-loaded, functionalized graphene oxides for chemotherapy and photothermal therapy. Int J Nanomed 11:2799–813.
- Jiang T, Sun W, Zhu Q, et al. (2015). Furin-mediated sequential delivery of anticancer cytokine and small-molecule drug shuttled by graphene. Adv Mater 27:1021–8.
- Hu Q, Sun W, Wang C, Gu Z. (2016). Recent advances of cocktail chemotherapy by combination drug delivery systems. Adv Drug Deliv Rev 98:19–34.
- Guo L, Shi H, Wu H, et al. (2016). Prostate cancer targeted multifunctionalized graphene oxide for magnetic resonance imaging and drug delivery. Carbon N Y 107:87–99.
- Siriviriyanun A, Popova M, Imae T, et al. (2015). Preparation of graphene oxide/dendrimer hybrid carriers for delivery of doxorubicin. Chem Eng J 281:771–81.
- Xu H, Fan M, Elhissi AM, et al. (2015). PEGylated graphene oxide for tumor-targeted delivery of paclitaxel. Nanomedicine 10:1247–62.
- Nasrollahi F, Varshosaz J, Khodadadi AA, et al. (2016). Targeted delivery of docetaxel by use of transferrin/poly(allylamine hydrochloride)-functionalized graphene oxide nanocarrier. ACS Appl Mater Interf 8:13282–93.
- Wei Y, Zhou F, Zhang D, et al. (2016). A graphene oxide based smart drug delivery system for tumor mitochondria-targeting photodynamic therapy. Nanoscale 8:3530–8.
- An B, Lin Y-S, Brodsky B. (2016). Collagen interactions: drug design and delivery. Adv Drug Deliv Rev 97:69–84.
- Ramezanpour M, Leung SSW, Delgado-Magnero KH, et al. (2016). Computational and experimental approaches for investigating nanoparticle-based drug delivery systems. Biochim Biophys Acta 1858:1688–709.
- Barnard AS. (2016). Challenges in modelling nanoparticles for drug delivery. J Phys Condens Matter 28:023002.
- Groh CM, Hubbard ME, Jones PF, et al. (2014). Mathematical and computational models of drug transport in tumours. J R Soc Interface 11:20131173.
- Ahmed S, Vepuri SB, Kalhapure RS, et al. (2016). Interactions of dendrimers with biological drug targets: reality or mystery – a gap in drug delivery and development research. Biomater Sci 4:1032–50.
- Grebner C, Iegre J, Ulander J, et al. (2016). Binding mode and induced fit predictions for prospective computational drug design. J Chem Inf Model 56:774–87.
- Shi C, Yuan D, Nangia S, et al. (2014). A structure–property relationship study of the well-defined telodendrimers to improve hemocompatibility of nanocarriers for anticancer drug delivery. Langmuir 30:6878–88.
- Shi C, Guo D, Xiao K, et al. (2015). A drug-specific nanocarrier design for efficient anticancer therapy. Nat Commun 6:7449.
- Liu J-Q, Li X-F, Gu C-Y, et al. (2015). A combined experimental and computational study of novel nanocage-based metal-organic frameworks for drug delivery. Dalt Trans 44:19370–82.
- Barraza LF, Jiménez VA, Alderete JB. (2015). Effect of PEGylation on the structure and drug loading capacity of PAMAM-G4 dendrimers: a molecular modeling approach on the complexation of 5-fluorouracil with native and PEGylated PAMAM-G4. Macromol Chem Phys 216:1689–701.
- Mohammed AAK, Burger SK, Ayers PW. (2014). Drug release by pH-responsive molecular tweezers: atomistic details from molecular modeling. J Comput Chem 35:1545–51.
- Metwally AA, Hathout RM. (2015). Computer-assisted drug formulation design: novel approach in drug delivery. Mol Pharm 12:2800–10.
- Kavousanakis ME, Kalogeropoulos NG, Hatziavramidis DT. (2014). Computational modeling of drug delivery to the posterior eye. Chem Eng Sci 108:203–12.
- Stanzione F, Jayaraman A. (2015). Computational design of oligopeptide containing poly(ethylene glycol) brushes for stimuli-responsive drug delivery. J Phys Chem B 119:13309–20.
- Duncan GA, Bevan MA, Brannon-Peppas L, et al. (2015). Computational design of nanoparticle drug delivery systems for selective targeting. Nanoscale 7:15332–40.
- Geetha P, Sivaram AJ, Jayakumar R, Gopi Mohan C. (2016). Integration of in silico modeling, prediction by binding energy and experimental approach to study the amorphous chitin nanocarriers for cancer drug delivery. Carbohydr Polym 142:240–9.
- Fahrenholtz SJ, Moon TY, Franco M, et al. (2015). A model evaluation study for treatment planning of laser-induced thermal therapy. Int J Hyperth 31:705–14.
- Trujillo M, Bon J, José Rivera M, et al. (2016). Computer modelling of an impedance-controlled pulsing protocol for RF tumour ablation with a cooled electrode. Int J Hyperth 32:931–9.
- Singh S, Repaka R. (2017). Temperature-controlled radiofrequency ablation of different tissues using two-compartment models. Int J Hyperth 33:122–34.
- Deshazer G, Prakash P, Merck D, Haemmerich D. (2017). Experimental measurement of microwave ablation heating pattern and comparison to computer simulations. Int J Hyperth 33:74–82.
- Wei Q, Tong X, Zhang G, et al. (2015). Nitrogen-doped carbon nanotube and graphene materials for oxygen reduction reactions. Catalysts 5:1574–1602.
- Lin TT, Lv QF. (2015). Preparation and application of nitrogen-doped graphene. J Function Mater 5:7–12.
- Lu Y, Huang Y, Zhang M, Chen Y. (2014). Nitrogen-doped graphene materials for supercapacitor applications. J Nanosci Nanotechnol 14:1134–44.
- Rao CNR, Gopalakrishnan K, Govindaraj A. (2014). Synthesis, properties and applications of graphene doped with boron, nitrogen and other elements. Nano Today 9:324–343.
- Frisch MJ, Trucks GW, Schlegel HB, et al. (2009). Gaussian 9. Wallingford, CT: Gaussian, Inc.
- Gill PMW, Johnson BG, Pople JA, Frisch MJ. (1992). The performance of the Becke–Lee–Yang–Parr (B-LYP) density functional theory with various basis sets. Chem Phys Lett 197:499–505.
- Becke AD. (1993). Density-functional thermochemistry. III. The role of exact exchange. J Chem Phys 98:5648–52.
- Rassolov VA, Ratner MA, Pople JA, et al. (2001). 6-31G* basis set for third-row atoms. J Comput Chem 22:976–84.
- Trott O, Olson AJ. (2010). AutoDock Vina: improving the speed and accuracy of docking with a new scoring function, efficient optimization, and multithreading. J Comput Chem 31:455–61.
- Morris GM, Huey R, Lindstrom W, et al. (2009). AutoDock4 and AutoDockTools4: automated docking with selective receptor flexibility. J Comput Chem 30:2785–91.
- Case DA, Berryman JT, Betz RM, et al. (2012). AMBER12. San Francisco: University of California.
- Wang J, Wolf RM, Caldwell JW, et al. (2004). Development and testing of a general amber force field. J Comput Chem 25:1157–74.
- Mahoney MW, Jorgensen WL. (2000). A five-site model for liquid water and the reproduction of the density anomaly by rigid, nonpolarizable potential functions. J Chem Phys 112:8910–22.
- Allen MP, Tildesley DJ, Computer simulation of liquids. Oxford: Clarendon Press; 1987.
- Yoneya M, Berendsen HJC, Hirasawa K (1994). A non-iterative matrix method for constraint molecular dynamics simulations. Mol Simul 13:395–405.
- Humphrey W, Dalke A, Schulten K. (1996). VMD: visual molecular dynamics. J Mol Graph 14:33–8.
- Wei D, Liu Y, Wang Y, et al. (2009). Synthesis of N-doped graphene by chemical vapor deposition and its electrical properties. Nano Lett 9:1752–8.
- Hildebrandt B, Wust P, Ahlers O, et al. (2002). The cellular and molecular basis of hyperthermia. Crit Rev Oncol Hematol 43:33–56.
- van der Zee J. (2002). Heating the patient: a promising approach?. Ann Oncol 13:1173–84.
- Yu L, Ren N, Yang K, et al. (2016). Photo/pH dual-responsive biocompatible poly(methacrylic acid)-based particles for triggered drug delivery. J Appl Polym Sci 133:44003.
- Mao J, Li Y, Wu T, et al. (2016). A simple dual-pH responsive prodrug-based polymeric micelles for drug delivery. ACS Appl Mater Interf 8:17109–17.
- Zhao H, Li Q, Hong Z. (2016). Paclitaxel-loaded mixed micelles enhance ovarian cancer therapy through extracellular pH-triggered PEG detachment and endosomal escape. Am Chem Soc 13:2411–22.
- Kneepkens E, Fernandes A, Nicolay K, Grüll H. (2016). Iron(III)-based magnetic resonance–imageable liposomal T1 contrast agent for monitoring temperature-induced image-guided drug delivery. Invest Radiol 51:735–45.
- Panja S, Dey G, Bharti R, et al. (2016). Tailor-made temperature-sensitive micelle for targeted and on-demand release of anticancer drugs. Am Chem Soc 8:12063–74.