Abstract
Objectives: To investigate the effects of a novel caesium-based thermal accelerant (TA) agent on ablation zone volumes following in vivo microwave ablation of porcine liver and skeletal muscle, and to correlate the effects of TA with target organ perfusion.
Materials and methods: This prospective study was performed following institutional animal care and use committee approval. Microwave ablation was performed in liver and resting skeletal muscle in eight Sus scrofa domesticus swine following administration of TA at concentrations of 0 mg/mL (control), 100 mg/mL and 250 mg/mL. Treated tissues were explanted and stained with triphenyltetrazolium chloride (TTC) for quantification of ablation zone volumes, which were compared between TA and control conditions. Hematoxylin and eosin (H&E) staining was also performed for histologic analysis. General mixed modelling with a log-normal distribution was used for all quantitative comparisons (p = 0.05).
Results: A total of 28 ablations were performed in the liver and 18 in the skeletal muscle. The use of TA significantly increased ablation zone volumes in a dose-dependent manner in both the porcine muscle and liver (p < 0.01). Both the absolute mean ablation zone volume and percentage increase in ablation zone volume were greater in the resting skeletal muscle than in the liver. In one swine, a qualitative mitigation of heat sink effects was observed by TTC and H&E staining. Non-lethal polymorphic ventricular tachycardia was identified in one swine, treated with intravenous amiodarone.
Conclusions: The use of a novel TA agent significantly increased mean ablation zone volumes following microwave ablation using a porcine model. The relationship between TA administration and ablation size was dose-dependent and inversely proportional to the degree of target organ perfusion, and a qualitative reduction in heat-sink effects was observed.
Introduction
The behaviour of microwave energy and its interactions with the dielectric properties of human tissues have been the subject of extensive prior investigation [Citation1–5]. In modern clinical practice, knowledge of these interactions is exploited for use in minimally invasive cancer therapies wherein two specific microwave frequencies (915 and 2450 MHz) are commonly utilised for solid tumour ablation. In this procedure, microwave energy is delivered from an external generator into an open-slot antenna placed directly into the tumour, either intraoperatively or percutaneously using imaging guidance with ultrasound (US), computed tomography (CT) or magnetic resonance (MR) imaging. The generation of local hyperthermia in the area surrounding the antenna results in irreversible cell injury, leaving behind a region of coagulative necrosis [Citation5–7]. This and other image-guided thermal ablation (IGTA) techniques – including radiofrequency (RF) ablation and cryoablation – offer several advantages over traditional surgical resection, including lower morbidity, improved preservation of surrounding tissues and a reduction in both hospitalisation cost and length of stay [Citation8–10].
The principal disadvantage of these energy-based treatment modalities is the risk of incomplete cancer cell death and resultant local tumour recurrence. Using currently available microwave ablation devices, the user is able to generate an ellipsoid ablation zone with a radius of approximately 5–20 mm. Within this zone, however, there is a gradient of circumferential isotherms, each with a unique profile of associated histologic effects ranging from complete necrosis to transient, reversible cell injury and reactive tissue hyperaemia. Accordingly, the phenomenon of incomplete ablation is felt to largely reflect the persistence of a population of viable cancer cells along the outer margin of the tumour following treatment [Citation5]. This appears to occur more commonly with radiofrequency ablation [Citation11] and, for this reason, the application of RF energy for oncologic therapy is typically reserved for tumours less than 3 cm in diameter [Citation12,Citation13]. Microwave ablation, by comparison, is associated with somewhat reduced recurrence risk, an effect that is mediated in part by higher ablation zone temperatures and faster ablations [Citation14].
Further contributing to the incidence of incomplete ablation is the heat sink effect, wherein cytotoxic thermal energy dissipates from the ablation zone secondary to flow within adjacent blood vessels; typically, those greater than 3 mm in diameter are considered significant [Citation15]. Prior work has demonstrated that flow rates as low as 1 ml/min within and around the ablation zone can reduce tissue heating [Citation16] and that the corresponding ablation margins may decrease by up to 11% [Citation17], thus increasing the rate of incomplete ablation.
In an attempt to mitigate these factors and improve treatment outcomes, we have created a novel agent intended to enhance the transmission of microwave energy within biologic tissues and generate larger ablation zones through an increase in the total volume of cytotoxic thermal energy. Preliminary results from an in vitro evaluation of this thermal accelerant (TA) have been previously published [Citation18] and demonstrated a more rapid increase in temperature beyond the cytotoxic threshold when compared with controls, a finding that was sustained over the entirety of the observed ablation period. Herein, we describe the results of in vivo ablation trials in swine with the hypothesis that TA administration will increase ablation zone volumes following microwave ablation in two different tissue types, and that the magnitude of this effect will correlate with the rate of blood flow to those tissues.
Materials and methods
The study design and animal usage parameters described herein were reviewed and approved by the Institutional Animal Care and Use Committee (IACUC) prior to study initiation. All animal welfare, housing and research procedures were conducted in compliance with federal animal welfare laws, policies and regulations, including the U.S. Department of Agriculture’s Animal Welfare Act, the Guide for the Care and Use of Laboratory Animals, the Guide for the Care and Use of Agricultural Animals in Research and Teaching, Public Health Service-National Institutes of Health Office of Laboratory Animal Welfare and the American Veterinary Medical Association Guidelines for Euthanasia.
Thermal accelerant (TA)
As previously described [Citation18], non-radioactive caesium chloride (CsCl; Sigma-Aldrich, St. Louis, MO) and a polyethylene glycol-based reverse phase-transition block-co-polymer (AK88; Akina Inc., West Lafayette, IN) were used to prepare the thermal accelerant. The polymer (200 mg) was mixed with a solution of 100–250 mg CsCl in 0.5 ml doubly distilled, deionised water. Following agitation overnight at 4 °C, the solution was diluted to 1 ml to prepare the TA.
In vivo trials
The ability of the thermal accelerant (TA) to increase ablation zone volumes was evaluated by conducting in vivo trials using 45–55 kg castrated male Sus scrofa domesticus swine as the animal model. To evaluate the effects of the TA on ablation in a highly vascular organ, serial microwave ablations were conducted in the porcine liver. Conversely, to evaluate the TA’s effects in a less-robustly perfused tissue, ablations were also performed in resting porcine skeletal muscle. A total of eight animals were used.
Following administration of general endotracheal anaesthesia by a board-certified doctor of veterinary medicine, midline laparotomy was performed. Continuous electrocardiographic monitoring, pulse oximetry, end-tidal carbon dioxide, and blood pressure monitoring was employed for real-time evaluation of animal hemodynamics and to assess for intraprocedural complications or cardiopulmonary toxicity. For all trials, microwave ablation was performed with a MicrothermX ST antenna (Perseon Medical, Salt Lake City, UT) at a frequency of 915 MHz using a power of 60 W and a TA dose of 100 mg/mL, or 250 mg/mL. Prior to ablation, a 1 ml bolus of TA was slowly injected parallel to the microwave antenna under ultrasound guidance at a distance of approximately 1.5 cm using a 5-french Yueh catheter (Cook Medical, Bloomington, IN). Ablation was then performed immediately following removal of the injection catheter. Depending on organ size and anatomy, up to four liver ablations and two skeletal muscle ablations were performed per swine. Multiple control trials were also performed using these methods without TA administration. Upon completion of the ablation trials, animals were euthanized by the veterinary staff using a sodium pentobarbital solution (Fatal Plus, Vortech Pharmaceutical Ltd, Dearborn, MI) and the ablated tissues were removed for gross and histologic evaluation.
In vivo and ex vivo imaging assessment
During the ablation trials, multiple real-time and static ultrasound images were acquired for assessment of the TA’s in vivo sonographic appearance using an GE Logic 9 ultrasound system with a 5–14 MHz transducer (General Healthcare, Milwaukee, WI). After explanation, the ablated tissues were immediately scanned using computed tomography (CT) (Optima CT540; GE Healthcare, Milwaukee, WI) to assess the TA’s CT appearance and to confirm retention of the TA at the site of administration.
Tissue characterization: triphenyltetrazolium chloride (TTC) staining and viability assessment
The ablated liver and skeletal muscle specimens were directly transported to the pathology department on ice in a shielded container following CT imaging. Each ablation zone was sectioned perpendicular to the long axis of the antennae. Sections measured approximately 4 mm in thickness and were first laid on a flat surface for visual examination. Each fresh tissue section was then rinsed in phosphate-buffered saline solution and incubated in 1% triphenyltetrazolium chloride solution (Sigma-Aldrich Corp., St. Louis, MO) for 20 min each using a light-blocked water bath at 37 °C. Sections were flipped after 10 min. The tissue was then removed and placed in 10% neutral-buffered formalin for an additional 10 min. Digital photographs were taken at a dedicated digital imaging workstation with measuring grid. Viable tissue was stained in a deep red colour, while nonviable tissue was left unstained.
The area of nonviable tissue within each section was measured using Image Pro Premier v9.1 digital image analysis software (Media Cybernetics, Rockville, MD). The total volume of nonviable tissue within each ablation zone was calculated as the product of ablation zone area and slice thickness, summed over the length of the total ablation zone.
Subsequently, routine haematoxylin and eosin (H&E) staining of the formalin-fixed sections of hepatic ablation zones and adjacent normal liver was performed for microscopic examination. Tissue samples were reviewed by light microscopy, and both ablation zone histology and associated cellular changes relative to adjacent normal liver tissue were evaluated.
Calculation of ablation volume
TTC-treated tissue sections were used to estimate the ablation volume. The ablated area of each tissue section was estimated by measuring a radius (control) or radii (TA) with subtraction of superimposed area. Subsequently, the tissue-sections belong to the same ablation were stacked together to calculate the ablation volume (Area × (n + 1), where n = number of cutting, thickness = ca. 4 mm).
Heat sink effects
To qualitatively evaluate the effects of TA administration on microwave ablation performed adjacent to large blood vessels, we identified a location within the liver of one swine that contained two major veins – each greater than 1.0 cm in diameter – running in parallel, separated by a distance of approximately 1.5 cm. The microwave antenna was placed at the mid-point between the two blood vessels, and thermal accelerant was introduced between the antenna and one of the identified veins. The morphology and size of the ablation zones were then assessed following TTC and H&E staining for tissue viability and histology, respectively.
Statistical methods
All statistical analyses were conducted using SAS Software 9.4 (SAS Inc., Cary, NC). Volume was modelled by both tissue type (muscle and liver) and TA concentration (0, 100 and 250 mg/mL) using general mixed modelling with a log-normal distribution and classic sandwich estimation, where observations were nested within pigs using PROC GLIMMIX. For purposes of replication and future power analyses, mean estimates were calculated along with interval estimates at a 95% confidence level; estimates of change, denoted as delta, were also examined. Significance was established a priori at 0.05. Multiple comparisons were examined using the Tukey method.
Results
Ablation zone volume
A total of 28 ablations were performed in the liver and 18 in the muscle. The liver and resting skeletal muscle ablation zone volumes were significantly increased 'with TA use in vivo when compared with controls (p < 0.01 for both). An interaction effect was observed for dosage and tissue type (p < 0.01); though an increase in volume was observed for both the liver and muscle, the magnitude of this increase differed between groups. Overall, ablations performed in skeletal muscle tissue yielded a larger mean ablation zone volume than those performed in the liver.
As can be seen in and , the mean ablation zone volume for skeletal muscle significantly increased by 55.5% between the control and 100 mg/mL TA dose conditions (p = 0.02). No significant difference in volume was observed between the 100 mg/mL and 250 mg/mL dosages, but a significant overall increase in mean volume of 69.7% was observed between control and the 250 mg/mL condition (p < 0.0001) ( and ). Similarly, a significant increase in mean ablation zone volume of 21.7% was observed in the liver when comparing the control and 100 mg/mL TA dose conditions (p < 0.0001), and a significant increase of 29.2% was also observed between the 100 mg/mL and 250 mg/mL TA conditions (p = 0.05). An overall increase in mean volume of 57.2% was observed in the liver between control and the 250 mg/mL condition (p < 0.0001) ( and ).
Figure 1. Mean ablation zone volumes in resting porcine skeletal muscle at increasing thermal accelerant concentrations.
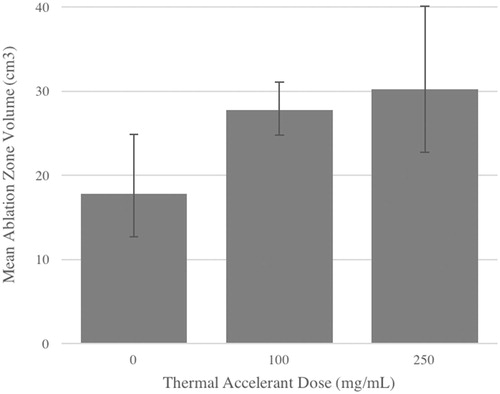
Figure 2. Gross tissue sections of porcine skeletal muscle following in vivo microwave ablation with and without 1 ml thermal accelerant at the 250 mg/mL dose. Ablation with TA (right) yielded a significantly larger ablation zone than without TA (left). Each grid measures 1 cm2.
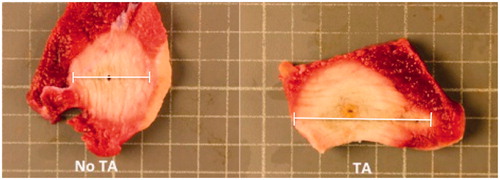
Figure 3. Mean ablation zone volumes in porcine liver at increasing thermal accelerant concentrations.
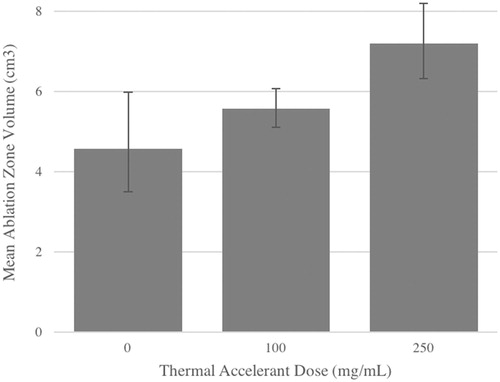
Figure 4. Gross tissue sections of porcine liver following in vivo microwave ablation with and without 1 ml thermal accelerant at the 250 mg/mL dose. Ablation with TA (left) yielded a significantly larger ablation zone than without TA (right). Note the visible TA injection mark (white arrow). Each grid measures 1 cm2.
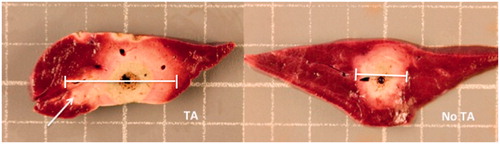
Table 1. Mean ablation zone volume estimates by tissue type and thermal accelerant concentration.
Table 2. Effect of thermal accelerant concentration on mean ablation zone volume by concentration interval.
Tissue viability
By TTC staining, microwave ablation produced three discrete viability zones (. Zone 1 consisted of a small brown area of distortion immediately adjacent to the probe that had undergone complete desiccation. Zone 2 consisted of a tan-white unstained area with some preserved live macrostructure. Zone 3 consisted of a ring of tissue with partial staining, consistent with viable, reversibly injured tissue.
Figure 5. Schematic of ablation zone anatomy by triphenyltetrazolium chloride staining following microwave ablation.
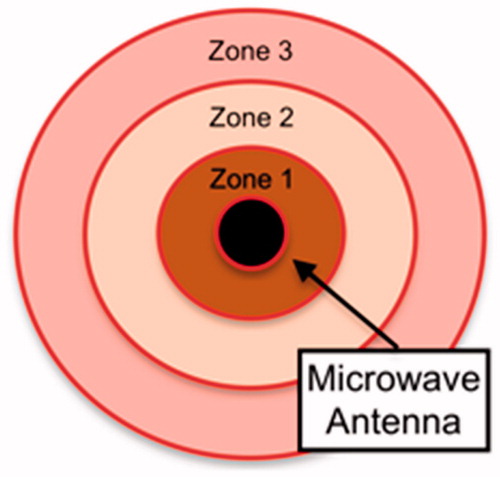
H&E-stained sections provided greater detail via microscopic examination (. Within Zone 1, the tissue was honeycomb-like, a finding derived from tissue vaporisation and bubble formation during ablation. The few remaining cells formed septa and bridges with marked nuclear smearing and streaming. In Zone 2, although the tissue was nonviable, it retained its lobular architecture. There were subtle changes noted within individual hepatocytes, with slightly oval-shaped nuclei arranged in parallel. Relative to zone 1, nuclear smearing was less common and decreased with increasing distance from the microwave antenna. The cytoplasm became dull and glassy. The sinusoidal spaces were slightly enlarged with very few red blood cells. The vascular channels lost their endothelial cells. Zone 3 started with a thin rim of congested tissue with sinusoidal spaces filled with red blood cells. Most hepatocytes appeared viable in this area, and the bile ducts remained intact.
Figure 6. H&E-stained section of porcine liver following in vivo microwave ablation with and without thermal accelerant. Ablation with TA (left) yielded a larger and more elongated ablation zone than without TA (right). An: antenna; AbT: ablated tissue; HT: healthy tissue; BV: blood vessel; Z: zone. A bar below the tissue image represents 5 mm.
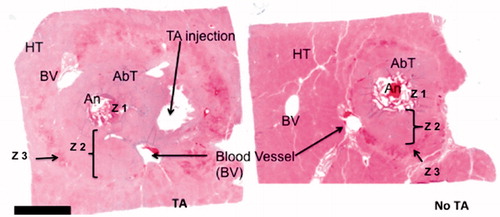
Heat sink effect
As demonstrated in , a qualitative difference was observed in ablation zone morphology with and without thermal accelerant administration adjacent to nearby hepatic veins. In the absence of TA, two discrete regions of viable tissue (as demonstrated by TTC staining) were identified interdigitating between the venous endothelium and the adjacent ablation zone, compatible with flow-related thermal diffusion away from Zone 3. Along the untreated margin, the ablation zone maintained a rounded, convex-outward configuration. In contrast, in the presence of interposed TA, the ablation zone directly approached and partially encompassed the adjacent vein, displaying concave-outward margins. The involved endothelium was thickened and somewhat distorted but remained viable by TTC staining.
Figure 7. Increased ablation zone volume following in vivo microwave ablation in porcine liver with TA use despite the presence of adjacent large blood vessels. Note the sharp interface between the ablation zone and adjacent hepatic vein in the absence of TA (open white arrows). In contrast, the ablation zone directly abuts and partially encompasses the other adjacent vein with TA administration (black arrow).
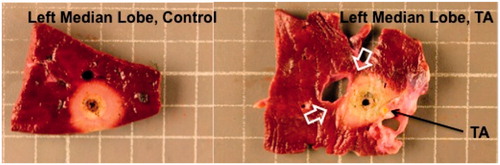
Imaging assessment
Following injection under ultrasound guidance, the TA appeared echogenic (. As the temperature within the TA increased, the echogenicity further increased commensurate with the degree of heating. Post-ablation CT images showed the TA as a discrete hyperattenuating area immediately adjacent to the hypoattenuation ablation zone (. Notably, the distance between the TA and the antenna had reduced to 1.0 cm from an in vivo distance of 1.5 cm secondary to ablation-induced tissue dehydration and contraction.
Toxicity
The median lethal dose (LD50) of CsCl in mice is 2300 mg/kg of body weight for oral administration and 910 mg/kg for intravenous injection [Citation19,Citation20]. Nevertheless, it was found that at high levels of caesium (i.e. up to 400 mg/mL per injection) administered to a 45 kg pig with a cumulative dose of approximately 4.0, a tachyarrythmia similar in morphology to polymorphic ventricular tachycardia (torsade de pointes) was provoked [Citation21,Citation22]. This was medically managed by the veterinarian by providing the anti-arrhythmic agent amiodarone (10–15 mg/kg). Subsequent to this event, all swine were treated with TA using a total caesium dose based on body weight (i.e. a total CsCl used <2 g per animal). No additional tachyarrythmic events or other signs of cardiopulmonary toxicity were observed.
Discussion
During microwave ablation of biologic tissues, heat is generated at the antenna site via kinetic energy produced from the rapid and synchronous alternating movements of polar molecules, primarily water. This energy propagates into the immediate surrounding tissues via conduction and causes cell death secondary to hyperthermia with resultant coagulative necrosis and apoptosis. Under these typical conditions, microwave energy transmission beyond the immediate ablation zone is limited by rapid, exponential decay [Citation23]. With the administration of a thermal accelerant agent adjacent to the intended ablation zone, however, there is a theoretical increase in the magnitude of tissue heating due to the presence of both thermal conduction and improved microwave energy delivery outside the immediate ablation zone, thereby extending treatment changes beyond the level currently achievable using a single antenna. This results in the same fundamental effect as increased microwave power without the associated increase in collateral tissue damage outside the treatment zone.
Using an in vivo swine model, we have herein demonstrated significantly increased ablation zone volumes through the use of a novel thermal accelerant agent following microwave ablation in different tissue types. The measured ablation zone volumes were consistently larger with TA than under control conditions in a dose-dependent manner. These preliminary results suggest a potential future role for thermal accelerant agents in the reduction of marginal recurrence rates following image-guided thermal ablation in humans.
In our study, the resting skeletal muscle demonstrated a larger absolute mean ablation zone volume when compared with the liver. Similarly, the overall percentage increase in mean ablation zone volume between the control and TA conditions was greater in the muscle than in the liver. These observations are likely reflective of the inherent hemodynamic differences between the two tissue types, with liver receiving a proportionally greater volume of cardiac output than skeletal muscle in the resting state with a resultant increase in perfusional heat loss.
The histologic effects of thermal accelerant were examined following ablation and tissue explanation. By H&E staining, a charring process was evident around the area in direct contact with the microwave antenna. Immediately adjacent to this within histologic zone 1, a 3 mm radius of complete desiccation occurred, producing a honeycomb-like structure derived from tissue vaporisation and bubble formation during the ablation. There was associated marked nuclear smearing and streaming within the remaining hepatocytes. These findings have been previously described [Citation24] and indicate extensive and synchronous agitation of polar intranuclear substances such as nucleic acids and proteins in response to the emitted alternating electric field from the antenna feed point, in turn yielding cytotoxic derangements. Thus, in zone 1 we observed two distinct effects leading to local cell death – indirect via improved heat conduction, and direct via improved microwave propagation.
In zone 2, the nuclear smearing observed within zone 1 was again present but appeared less obvious at greater distances from the antenna, concordant with the exponential decay in energy observed in prior in vitro trials [Citation18]. Using this finding as a surrogate for microwave-induced cytotoxicity, the histologic changes in zone 2 also appeared to derive from both direct (microwave) and indirect (thermal conduction) mechanisms, as in zone 1. Given that zone 2 under control conditions was characterised predominantly by temperature-mediated cytotoxic effects alone, this supports the hypothesis that thermal accelerant improves microwave transmission within biologic tissues.
On histologic analysis, zone 3 was characterised by a thin rim of congested tissue surrounding the entirety of zone 2. The sinusoidal spaces were filled with red blood cells and most hepatocytes in this region appeared viable, compatible with the region-at-risk for local tumour recurrence following image-guided tumour ablation. With thermal accelerant administration, there was observed to be a shift of viable zone 3 hepatocytes away from the centre of the ablation zone when compared with controls due to a corresponding enlargement of zone 2 by the effects described above. This represents the histologic correlation of the increased ablation zone volumes observed macroscopically; as microwave energy transmission is augmented by TA, viable cells along the outer margin of the ablation zone shift further outward secondary to expansion of the central cytotoxic ablation zone core. Additional work is necessary to demonstrate the translation of this observation into reduced rates of incomplete ablation.
The heat sink effect describes the phenomenon of thermal diffusion away from the ablation zone secondary to flow within adjacent blood vessels, which has been associated with decreased ablation zone size in multiple studies. This topic has been most extensively studied in the liver. In an early investigation by Goldberg et al. [Citation25], the adminsitration of vasoactive agents such as epinephrine and halothane significantly altered hepatic blood flow, and there was a significant relationship between blood flow changes and ablation zone volume with RF ablation. Patterson et al. subsequently demonstrated a significant positive association between the absolute number of blood vessels within 1 cm radius from a radiofrequency antenna and final ablation size [Citation26]. In a separate study by Goldberg et al. [Citation27], the use of direct portal venous occlusion resulted in a significantly greater area of coagulative necrosis than under normal physiologic conditions. These and other trials firmly established the role of heat sink effects on local thermal ablation outcomes and suggested that methods for heat sink mitigation may be of value in improving therapeutic outcomes.
In our study, we qualitatievly assessed ablation zone size and morphology in the liver following ablation performed in the presence of large adjacent blood vessels with and without thermal accelerant administration. We noted a difference in ablation zone morphology, with coagulative necrosis directly apposing and even partially enveloping the adjacent hepatic vein in the presence of TA without evidence of significant endothelial injury. In contrast, in the absence of TA there was a clear demarcation of viable hepatocytes interposed between the ablation zone and adjacent vessel by TTC staining. Though limited in generalizability, these observations suggest that the use of TA may be sufficient to overcome heat sink effects in vivo through improvements in microwave energy transmission beyond the central ablation zone. Further work is necessary to validate these findings using quantitative methods in a larger cohort with a greater number of observations.
The use of sodium chloride as an additive for augmentation of thermal energy delivery during thermal ablation has been the subject of multiple prior investigations. First described by Livraghi et al. [Citation28], saline was found to significantly increase ablation zone volumes in both ex vivo and in vivo animal liver experiments following RF ablation. In a canine ablation model study by Ahmed et al. [Citation29], RF-mediated tissue heating was significantly elevated in the presence of intratumoral saline, and complete tumour necrosis was achieved in all cases, even for large lesions greater than 3.0 cm. This effect was found to be proportional to the sodium chloride concentration. Subsequent articles demonstrated similar results treating hepatocellular carcinoma in humans [Citation30,Citation31].
Our thermal accelerant agent is similar in mechanism, with increased free water content and higher molecular dipole moment leading to improved energy transmission and greater coagulative necrosis. Unlike saline, however – which can freely dispserse within soft tissues due to its low viscosity [Citation32] – the thermal accelerant agent described herein demonstrates temperature-dependent biphasic properties, existing as a liquid at room temperature and a viscous gel at 37 °C. This behaviour improves localisation of TA within the intended region of treatment, which may improve energy deliver to the lesion of interest and minimise unwanted extension of the ablation zone into adjacent structures. Additionally, caesium chloride’s higher atomic number allows for improved intraprocedural visualisation compared with sodium chlorid when performing ablations using CT guidance.
This study has several limitations. As ablation trials were performed in normal healthy porcine tissues, the observed increase in ablation zone volume with TA may not accurately reflect the expected magnitude of volume change in neoplastic tissues, which differ in their cellular composition. The use of laparotomy for optimisation of ablation probe and TA placement may have affected the accuracy of our results, and further evaluation using a percutaneous approach is necessary. Finally, because animals were euthanized immediately following ablation, delayed toxicity or other complications could not be assessed, and survival trials are needed to establish long-term safety of the thermal accelerant agent.
Conclusions
In this study, the properties of a novel thermal accelerant were examined using an in vivo swine model, and we demonstrated increased ablation zone volumes in the liver and resting skeletal muscle with TA use when compared with control ablations. The magnitude of volume increase was dose-dependent and correlated with the degree of tissue perfusion. The TA was clearly visible using ultrasound and CT, and remained in place at the site of deposition following microwave ablation. At higher accumulated doses (>4 g), nonlethal cardiotoxicity was observed in one animal, necessitating medical intervention. Finally, we identified histologic changes suggestive of mitigation of heat sink effects using TA in one porcine liver specimen. Taken together, these findings suggest augmentation of microwave energy in vivo and support further investigation into the optimisation of our thermal accelerant agent with the ultimate goal of effective, non-toxic utilisation in human cancer patients.
Acknowledgments
The authors wish to thank Stephanie Bolcon for her assistance with ultrasound imaging, Christen D Owen and Rebecca Hamelin for their veterinary assistance, and Wendy J Smith and Susan M Foley for assisting with the in vivo experiments. WKCP thanks RIMI and Dr John J. Cronan for their financial support.
Disclosure statement
The authors report no conflicts of interest. The authors alone are responsible for the content and writing of the article.
References
- Gabriel C, Gabriel S, Corthout E. (1996). The dielectric properties of biological tissues: I. Literature survey. Phys Med Biol 41:2231–49.
- Gabriel S, Lau RW, Gabriel C. (1996). The dielectric properties of biological tissues: III. Parametric models for the dielectric spectrum of tissues. Phys Med Biol 41:2271–93.3.
- Lubner MG, Brace CL, Hinshaw JL, Lee FT. (2010). Microwave tumor ablation: mechanism of action, clinical results, and devices. J Vasc Interv Radiol 21(8 Suppl):S192–S203.
- Ward RC, Healey TT, Dupuy DE. (2013). Microwave ablation devices for interventional oncology. Expert Rev Med Devices 10:225–38.
- Chu KF, Dupuy DE. (2014). Thermal ablation of tumours: biological mechanisms and advances in therapy. Nat Rev Cancer 14:199–208.
- Mizukoshi E, Yamashita T, Arai K, et al. (2013). Enhancement of tumor-associated antigen-specific T cell responses by radiofrequency ablation of hepatocellular carcinoma. Hepatology 57:1448–57.
- Keisari Y, Hochman I, Confino H, et al. (2014). Activation of local and systemic anti-tumor immune responses by ablation of solid tumors with intratumoral electrochemical or alpha radiation treatments. Cancer Immunol Immunother 63:1–9.
- Ahmed M, Brace CL, Lee FT, Goldberg SN. (2011). Principles of and advances in percutaneous ablation. Radiology 258:351–69.
- Spolverato G, Vitale A, Ejaz A, et al. (2015). The relative net health benefit of liver resection, ablation, and transplantation for early hepatocellular carcinoma. World J Surg 39:1474–84.
- Welch BT, Brinjikji W, Schmit GD, et al. (2015). A national analysis of the complications, cost, and mortality of percutaneous lung ablation. J Vasc Interv Radiol 26:787–91.
- Wright AS, Sampson LA, Warner TF, et al. (2005). Radiofrequency versus microwave ablation in a hepatic porcine model. Radiology 236:132–9.
- Lu DS, Yu NC, Raman SS, et al. (2005). Radiofrequency ablation of hepatocellular carcinoma: treatment success as defined by histologic examination of the explanted liver. Radiology 234:954–60.
- Lanuti M, Sharma A, Willers H, et al. (2012). Radiofrequency ablation for stage I non-small cell lung cancer: management of locoregional recurrence. Ann Thorac Surg 93:921–7.
- Velez E, Goldberg SN, Kumar G, et al. (2016). Hepatic thermal ablation: effect of device and heating parameters on local tissue reactions and distant tumor growth. Radiology 281:782–92.
- Lu DS, Raman SS, Vodopich DJ, et al. (2002). Effect of vessel size on creation of hepatic radiofrequency lesions in pigs: assessment of the "heat sink" effect. AJR Am J Roentgenol 178:47–51.
- Lehmann KS, Poch FG, Rieder C, et al. (2016). Minimal vascular flows cause strong heat sink effects in hepatic radiofrequency ablation ex vivo. J Hepatobiliary Pancreat Sci 23:508–16.
- Deshazer G, Merck D, Hagmann M, et al. (2016). Physical modeling of microwave ablation zone clinical margin variance. Med Phys 43:1764.
- Park WKC, Maxwell AWP, Frank VE, et al. (2017). evaluation of a novel thermal accelerant for augmentation of microwave energy during image-guided tumor ablation. Theranostics 7:1026.
- Hands-on Science (H-Sci) Project: Chemical Safety Database. Physical and Theoretical Chemistry Laboratory. Oxford University.
- Chemical and Other Safety Information. The Physical and Theoretical Chemistry Laboratory, Oxford University.
- Wiens M, Gordon W, Baulcomb D, et al. (2009). Cesium chloride-induced torsades de pointes. Can J Cardiol 25:e329–31.
- Sessions D, Heard K, Kosnett M. (2013). Fatal cesium chloride toxicity after alternative cancer treatment. J Altern Complement Med 19:973–5.
- Garrean S, Hering J, Saied A, et al. (2009). Ultrasound monitoring of a novel microwave ablation (MWA) device in porcine liver: lessons learned and phenomena observed on ablative effects near major intrahepatic vessels. J Gastrointest Surg 13:334–40.
- Gravante G, Ong SL, Metcalfe MS, et al. (2008). Hepatic microwave ablation: a review of the histological changes following thermal damage. Liver Int 28:911–21.
- Goldberg SN, Hahn PF, Halpern EF, et al. (1998). Radio-frequency tissue ablation: effect of pharmacologic modulation of blood flow on coagulation diameter. Radiology 209:761–7.
- Patterson EJ, Scudamore CH, Owen DA, et al. (1998). Radiofrequency ablation of porcine liver in vivo: effects of blood flow and treatment time on lesion size. Ann Surg 227:559–65.
- Goldberg SN, Hahn PF, Tanabe KK, et al. (1998). Percutaneous radiofrequency tissue ablation: does perfusion-mediated tissue cooling limit coagulation necrosis? J Vasc Interv Radiol 9(1 Pt 1):101–11.
- Livraghi T, Goldberg SN, Monti F, et al. (1997). Saline-enhanced radio-frequency tissue ablation in the treatment of liver metastases. Radiology 202:205–10.
- Ahmed M, Lobo SM, Weinstein J, et al. (2002). Improved coagulation with saline solution pretreatment during radiofrequency tumor ablation in a canine model. J Vasc Interv Radiol 13:717–24.
- Kettenbach J, Köstler W, Rücklinger E, et al. (2003). Percutaneous saline-enhanced radiofrequency ablation of unresectable hepatic tumors: initial experience in 26 patients. AJR Am J Roentgenol 180:1537–45.
- Giorgio A, Tarantino L, de Stefano G, et al. (2003). Percutaneous sonographically guided saline-enhanced radiofrequency ablation of hepatocellular carcinoma. AJR Am J Roentgenol 181:479–84.
- Gillams AR, Lees WR. (2005). CT mapping of the distribution of saline during radiofrequency ablation with perfusion electrodes. Cardiovasc Intervent Radiol 28:476–80.