Abstract
Purpose: The aim of this study is to investigate whether radiofrequency ablation (RFA) improves the efficacy of adoptive T cell immunotherapy in preclinical mouse cancer models.
Method: Mice implanted subcutaneously (sc) with syngeneic colon adenocarcinoma or melanoma were treated with sub-curative in situ RFA (90 °C, 1 min). Trafficking of T cells to lymph nodes (LN) or tumors was quantified by homing assays and intravital microscopy (IVM) after sham procedure or RFA. Expression of trafficking molecules (CCL21 and intercellular adhesion molecule-1 [ICAM-1]) on high endothelial venules (HEV) in LN and tumor vessels was evaluated by immunofluorescence microscopy. Tumor-bearing mice were pretreated with RFA to investigate the therapeutic benefit when combined with adoptive transfer of in vitro-activated tumor-specific CD8+ T cells.
Results: RFA increased trafficking of naïve CD8+ T cells to tumor-draining LN (TdLN). A corresponding increase in expression of ICAM-1 and CCL21 was detected on HEV in TdLN but not in contralateral (c)LN. IVM revealed that RFA substantially enhanced secondary firm arrest of lymphocytes selectively in HEV in TdLN. Furthermore, strong induction of ICAM-1 in tumor vessels was associated with significantly augmented trafficking of adoptively transferred in vitro-activated CD8+ T cells to tumors after RFA. Finally, preconditioning tumors with RFA augmented CD8+ T cell-mediated apoptosis of tumor targets and delayed growth of established tumors when combined with adoptive T cell transfer immunotherapy.
Conclusions: These studies suggest that in addition to its role as a palliative therapeutic modality, RFA may have clinical potential as an immune-adjuvant therapy by augmenting the efficacy of adoptive T cell therapy.
Introduction
Adoptive cell therapy (ACT) with tumor antigen-specific T cells has emerged as a promising approach for treating various cancers [Citation1–10]. ACT using autologous tumor-infiltrating lymphocytes (TIL) has been reported to mediate objective tumor regression in 50–70% of metastatic melanoma patients in combination with lymphodepleting chemotherapy and systemic high-dose IL-2 administration [Citation10,Citation11]. In addition, ACT with gene-modified peripheral blood lymphocytes engineered to express T cell receptors (TcR) or chimeric antigen receptors (CAR) specific for tumor-associated antigens can mediate regression in multiple cancer histologies [Citation1–8]. ACT has demonstrated durable clinical response in significant numbers of patients with hematological malignancies; however, this success has not reproducibly translated into solid malignancies.
One of the major obstacles for ACT is limited trafficking of tumor-reactive cytotoxic CD8+ T cells across microvascular checkpoints in solid tumors despite the presence of local inflammatory cytokines [Citation12,Citation13]. Trafficking of infused cytotoxic T cells to tumor sites is a critical step for successful immunotherapy [Citation14]. T cell trafficking proceeds by a stepwise cascade of adhesion and activation events. Briefly, circulating T cells utilize the selectin family of adhesion proteins and their ligands to slowly roll along blood vessels. This primary adhesion step leads to chemokine-dependent activation of β2 integrins which enables T cells to stably adhere to endothelial counter-receptors such as intercellular adhesion molecule-1 (ICAM-1). This secondary firm adhesion step is a prerequisite for T cell extravasation from the blood compartment into tumor sites or lymph nodes (LN) [Citation12,Citation15]. The tumor microenvironment; however, is characterized by aberrant vasculature and interstitium, which limits T cell infiltration into the tumor parenchyma [Citation16,Citation17]. Preclinical murine studies established that only a minor fraction of ex vivo expanded T cells can infiltrate into the tumor tissue during ACT, thus allowing tumor cell targets to escape from contact-dependent killing by adoptively transferred T cells [Citation12,Citation18]. Of note, long-term efficacy of ACT depends not only on direct access of transferred cytotoxic CD8+ T cells to the tumor microenvironment, but also localization of transferred T cell subsets (i.e., naïve, stem cell memory [TSCM] and central memory [TCM]) in secondary lymphoid organs such as LN. Tumor-draining LN (TdLN) that are enriched for tumor antigens serve as a site for in situ expansion of adoptively transferred T cells into cytotoxic CD8+ T cells [Citation19–21]. Thus, regimens that dually boost T cell trafficking to the tumor microenvironment and LN would be expected to improve current ACT regimens.
In situ thermal ablation techniques, such as radiofrequency ablation (RFA) and cryoablation, have emerged as promising treatment options for unresectable solid malignant tumors [Citation22–24]. RFA and cryoablation are approved by the US Food and Drug Administration for ablation of primary and metastatic tumors and have been applied in the treatment of a variety of neoplasms including liver, colorectal, lung, melanoma, prostate, kidney, breast, brain, thyroid, and bone tumors [Citation22,Citation23]. Interestingly, in addition to producing localized tumor destruction, thermal ablation has been shown to elicit immunomodulatory effects in preclinical and clinical studies [Citation24–32]. The ablated tumor tissue remains in situ and provides a source of tumor antigens that are taken up and processed by dendritic cells (DC) and presented to T lymphocytes in TdLN [Citation24,Citation33,Citation34]. RFA or cryoablation activate adaptive immune responses through increased migration of Ag-loaded DC to TdLN and enhanced accumulation of endogenous T cells in TdLN and the tumor microenvironment [Citation25–27]. However, a clinical issue is that while RFA induces coagulative necrosis in the tumor mass, there often remains a surrounding non-ablated zone of viable cells and the endogenous immune response is not sufficient to prevent either local recurrence or metastasis. Therapeutic strategies that combine RFA with resection, cytokine therapy, immune checkpoint blockade, or vaccination including DC-based vaccines can improve outcomes in preclinical models and patients [Citation25,Citation32,Citation35–43]. RFA was reported to alter the distribution of circulating T cells [Citation30], but the influence of this ablative treatment on T cell trafficking to LN and the tumor microenvironment is an open area of investigation.
Here, we examined whether RFA could improve trafficking of antigen-specific T cells across tumor vessels and high endothelial venules (HEV) in lymphoid organs in preclinical models of melanoma and colon cancer. We report that RFA increases the intravascular display of critical gatekeeper trafficking molecules in TdLN and the tumor, effectively mobilizing T cells to TdLN and the tumor microenvironment while augmenting the antitumor efficacy of adoptively transferred antigen-specific CD8+ T cells.
Methods
Animals
Female BALB/c and C57BL/6 mice (8–12-week) were from National Cancer Institute or Charles River (Wilmington, MA, USA). OT-I mice (C57BL/6-Tg(TcraTcrb)1100Mjb/J) expressing an α/β TcR specific for ovalbumin (OVA) were from The Jackson Laboratory (Bar Harbor, ME, USA). Clone 4 mice (CBy.Cg-Thy1a Tg(Tcra(C14,TcrbC14)1Shrm/ShrmJ) expressing an α/β TcR specific for influenza hemagglutinin (HA; from Linda Sherman, Scripps Research Institute, La Jolla, CA, USA) were bred in the Roswell Park Department of Laboratory Animal Resources. Mice were maintained in pathogen-free barrier conditions. All animal care and procedures were in accordance with institutional policies for animal health and well-being and approved by the Roswell Park Comprehensive Cancer Center Institutional Animal Care and Use Committee (IACUC).
Tumor models
Tumor cells (CT26 colon adenocarcinoma cells and the CT26-HA derivative transfected with a gene encoding HA, syngeneic to BALB/c mice; B16.F10 melanoma and the B16F10-OVA derivative transfected with OVA, syngeneic to C57BL/6 mice [Citation12,Citation18,Citation32,Citation44]) were cultured in RPMI 1640 supplemented with 10% heat-inactivated fetal calf serum (FCS) (Life Technologies, Carlsbad, CA, USA), 2 mM L-glutamine, 100 U/ml penicillin, 50 µg/ml streptomycin, 50 μM β-mercaptoethanol, and G418 (400 μg/ml; for CT26-HA). Tumor cells (106 for CT26 and CT26-HA or 3 × 105 for B16F10 and B16-OVA; in 100 μL PBS) were injected sc in the left flank of syngeneic mice.
RFA
RFA was performed as previously described to produce sub-curative ablation when used as a monotherapy [Citation32]. In brief, mice were anesthetized with inhalational isoflurane gas (4% isoflurane for anesthesia induction; 1.5% for maintenance). Mice were positioned prone on an electricity-conducting grounding pad and the tumor area was wet with distilled water. A StarBurst SDE probe (AngioDynamics, Inc., Latham, NY, USA) was inserted into the center of the tumor and probe tip temperature was maintained at 90 °C for 1 min using the RFA RITA 1500 generator (AngioDynamics). This treatment regimen simulated the clinical setting of tumor recurrence after RFA, as previously described by Johnson et al. [Citation39]. Sham RFA was performed by inserting the probe into the tumor without electrical current. Mice were recovered on a warming blanket and given analgesic (buprenorphine 0.05 mg/kg body weight, administered sc) for pain control.
In vivo homing assay
Short-term (1 h and 12 h) homing assays were performed as described [Citation12,Citation15,Citation44,Citation45]. For studies involving the homing of naive CD8+ T cells, freshly isolated splenocytes and popliteal, inguinal, mesenteric, axillary, and brachial LN cells from OT-I or Clone 4 transgenic mice were prepared as single-cell suspensions and pooled. Lymphoid cells were negatively enriched by the Magnetic Activated Cell Sorting device (Miltenyi Biotec, Auburn, CA, USA), using the naïve CD8a T-cell isolation kit according to the manufacturer’s protocol. For studies involving the homing of activated CD8+ T cells, freshly isolated splenocytes from Clone 4 or OT-I TcR-transgenic mice were activated for 2 days in vitro with plate-bound anti-mouse CD3 antibody (Ab) (145-2C11; BD Biosciences, San Jose, CA, USA), followed by treatment for 3 days with recombinant IL-2 (12.5 ng/ml; R&D Systems, Minneapolis, MN, USA) in RPMI 1640 supplemented with 10% FCS, 2 mM L-glutamine, 100 U/ml penicillin, 50 μg/ml streptomycin, and 50 μM β-mercaptoethanol (Invitrogen, Carlsbad, CA, USA) [Citation46].
CD8+ T cells at a density of 5 × 107 cells per ml were labeled for 20 min at 37 °C with 180 μg/ml of TRITC (tetramethylrhodamine-6-isothiocyanate; Molecular Probes, Eugene, OR, USA) in RPMI 1640 medium (Invitrogen) and labeling was stopped by centrifugation through a 'cushion' of FCS (Invitrogen) [Citation15,Citation45,Citation47,Citation48]. Within a given experiment, equivalent numbers of labeled cells (1–2 × 107 cells per mouse in 300 μl PBS) were injected intravenously (iv) via the tail vein into RFA- or sham-treated mice bearing 10–11 day established tumors. Organs were collected 1 or 12 h after cell transfer, embedded in OCT freezing medium, and snap-frozen in liquid nitrogen. Tissues were counterstained with primary Ab specific for vascular markers to demark the position of peripheral lymph node addressin-positive (PNAd+) HEV in LN (i.e., MECA79 Ab, 20 µg/ml, BD Biosciences) or CD31+ vessels in tumors, pancreas, or spleen (MEC13.1 Ab, 20 µg/ml, BD Biosciences). After washing, primary Ab was detected by fluorochrome-conjugated (fluorescein) goat-anti-rat IgM, or goat-anti-rat IgG, respectively (Jackson ImmunoResearch, Laboratories, Inc., West Grove, PA, USA). The number of fluorescence-labeled transferred cells was quantified, double-blinded, using a BH2/RFL fluorescence microscope (Olympus Optical, Center Valley, PA, USA) in ≥10 fields (unit area of each field, 0.34 mm2) of non-sequential cryosections 9 μm in thickness.
To evaluate trafficking of red blood cells (RBC), freshly isolated splenocytes from BALB/c mice were prepared as single-cell suspensions. After using density-gradient centrifugation with Ficoll-Paque (GE Healthcare Life Sciences, Pittsburgh, PA, USA), pelleted cells (>90% RBC) were collected and labeled with TRITC before infusion as described above. Target organs were collected after 2 h to allow for sufficient numbers of RBC to be detected in organs.
Immunofluorescence analysis of vascular trafficking molecules
Methods used for immunofluorescence staining and analyses are based on validated published protocols [Citation12,Citation15,Citation18,Citation44,Citation45]. Tissue cryosections (9 µm) were incubated with goat serum (1:10 dilution) for 10 min to block Fc receptors. Primary Ab specific for the following antigens was then applied to designated tissues for 1 h at room temperature: PNAd (MECA79, 20 µg/ml, BD Biosciences) and CD31 (MEC13.1, 20 µg/ml, BD Biosciences). After washing, primary Ab was detected by fluorochrome-conjugated (fluorescein) goat-anti-rat IgM, or goat-anti-rat IgG, respectively (Jackson ImmunoResearch). For intravascular staining, mAb specific for ICAM-1 (3E2, 50 µg/mouse, BD Biosciences); goat polyclonal Ab for CCL21 (10 µg/mouse; R&D Systems); were injected iv via the tail vein as described [Citation12,Citation15,Citation44,Citation45]. After 20 min, organs were frozen and cryosections (9 µm) were stained with rhodamine-conjugated secondary Ab. Prior studies established that isotype control Ab at the same concentrations did not provide a positive immunofluorescence signal [Citation12,Citation15,Citation18,Citation44,Citation45]. Digital images were captured with an Olympus BX50 upright fluorescence microscope equipped with a SPOT RT camera (Spectra Services, Inc., Ontario, NY, USA). All images were captured with identical exposure times and image settings in each experiment. Images were analyzed with ImageJ software [Citation49] (http://rsb.info.nih.gov/ij) for double-blinded analysis of the relative fluorescence staining intensity for trafficking molecules on all PNAd+ HEV in n ≥ 10 fields of non-sequential 9 μm thick cross-section of PNAd+ LN HEV or CD31+ vessels within tumor tissues [Citation12,Citation15,Citation45]. For normalization of the varying sizes of individual vessels in cryosections, fluorescence intensity is expressed in terms of pixels (reflecting a fixed unit area). For this, vascular endothelium was encircled during analysis based on PNAd or CD31 staining and each pixel in those defined regions was assigned a fluorescence intensity value (based on a scale from 0 to 255). Numeric mean fluorescence intensity (MFI) values and histograms represent data from all pixels analyzed within fields; similar numbers of vessels and pixels were analyzed for each treatment condition.
Intravital microscopy
Intravital microscopy (IVM) of inguinal TdLN was performed as described [Citation15,Citation44,Citation47,Citation50]. Mice were anesthetized by intraperitoneal injection of 1 mg/ml of xylazine and 10 mg/ml of ketamine (10 ml per kg body weight). The left inguinal TdLN was exposed in an abdominal skin flap and the surrounding fatty tissue was removed to expose the LN microvasculature. Approximately, 2.5 × 107 calcein-labeled splenocytes were injected through a catheter inserted into the right femoral artery and were visualized with a customized IVM system (Spectra Services). Brightfield microscopy was used to identify the vascular structure and blood flow status in venular branches; fluorescent microscopy was used to visualize calcein-labeled cells. At the end of the observation period, 150-kilodalton fluorescein isothiocyanate-conjugated dextran (10 mg/ml; Molecular Probes) was injected to define the venular structure. All images were captured with an EB charge-coupled device camera (Hamamatsu Photonics, Shizuoka, Japan) and were recorded with a digital videocassette recorder (DSR-11, Sony, Tokyo, Japan) for off-line analysis of cell activity. The rolling fraction was defined as the percentage of total calcein-labeled cells passing through the vessel that transiently interacted with HEV during the observation period [Citation15,Citation44,Citation47,Citation50]. The sticking fraction was defined as the percentage of rolling cells that adhered to HEV for ≥30 s. For rolling and sticking fractions, data were generated from independent experiments for three sham-treated control mice and three RFA-treated mice.
Adoptive T cell therapy, TUNEL assay, and growth delay studies
For TUNEL assays, 2 × 107 in vitro-activated OT-I CD8+ T cells were adoptively transferred, and TUNEL+ cells (Apoptag Kit; Millipore, Burlington, MA, USA) were quantified in ≥10 fields (unit area of each field, 0.34 mm2) in non-sequential 9 μm thick cryosections. For tumor growth delay studies, mice (n = 5 for all groups) were treated 10–11 days post tumor implantation with iv adoptive transfer of in vitro-activated OT-I or Clone 4 CD8+ T cells (106 cells transferred per mouse). Tumor volumes were calculated by determining the length of long (L) and short (S) diameters (volume = (L × S×S)/2). Experimental endpoints were reached when tumors exceeded 20 mm in diameter or when mice became moribund and showed signs of lateral recumbency, cachexia, lack of response to noxious stimuli, or observable weight loss.
Statistical analyses
Experimental design and samples sizes were based on prior experience for published data shown to be sufficient to demonstrate statistically and biologically significant differences [Citation12,Citation15,Citation18,Citation44,Citation45]. All data sets except for tumor growth delay and survival experiments were compared using 2-tailed unpaired Student's t test. Tumor growth delay was analyzed by Mann–Whitney U test and survival was analyzed with the Kaplan–Meier method using GraphPad Prism 5.0 (GraphPad Software Inc., San Diego, CA, USA), and groups were compared using log-rank (Mantel–Cox) test. Data are presented as mean ± SEM and p < 0.05 was considered statistically significant.
Results
RFA enhances CD8+ T cell trafficking in TdLN
We previously reported that fever-range systemic thermal therapy (core temperature elevated to 39.5 ± 0.5 °C for 6 h) stimulates lymphocyte-HEV interactions and increases homing of lymphocytes to secondary lymphoid organs with HEV structures [Citation12,Citation15,Citation44]. However, the influence of local thermal ablation on adhesive interactions in LN that drain the treatment site remains unknown. To investigate whether RFA enhances lymphocyte entry across HEV, we evaluated T cell homing to LN in mice shortly after intratumoral RFA treatment. RFA was administered to B16F10 melanoma and CT26 colon adenocarcinoma expressing surrogate tumor-associated antigens; ovalbumin (B16-OVA) or hemagglutinin (CT26-HA), respectively. CD8+ T cells from OT-I or Clone 4 TcR-transgenic mice were used to monitor trafficking of tumor-antigen (OVA or HA, respectively)-specific T cells to LN. For these studies, B16-OVA or CT26-HA sc tumors of approximately equivalent size within a given experiment (ranging from ∼150–500 mm3 volume) were treated with RFA (1 min of ablation at 90 °C) on day 10–11 after implantation as previously described [Citation32]. This model for sub-curative RFA treatment generates a core of coagulative necrosis surrounded by a residual viable zone that experienced non-lethal temperatures [Citation32]. Control mice underwent sham procedure in which the probe was inserted into the tumor without electrical current. Based on our previous data that fever-range systemic thermal therapy requires ∼6 h to exert maximal pro-trafficking effects on HEV [Citation15,Citation44,Citation45], we performed adoptive transfer of fluorescence-labeled OT-I or Clone 4 CD8+ T cells 6 h after RFA/sham treatment of mice bearing B16-OVA or CT26-HA, respectively. We initially used non-activated naïve CD8+ T cells (isolated from spleens of OT-I or Clone 4 mice) for homing assays since L-selectin+ naïve T cells, TSCM, and TCM, but not cytotoxic T cells, are the major populations that traffic to LN [Citation12]. We quantified fluorescent-tagged CD8+ T cells in TdLN and contralateral LN (i.e., cLN, outside of treatment region) at 1 h or 12 h after iv ACT (. The 1 h time-point allows for stringent evaluation of the entry rate of blood-borne T cells via HEV while analysis at 12 h reflects T cell trafficking across HEV, together with T cell retention and the rate of egress from LN via the lymphatic system [Citation51].
Figure 1. RFA enhances lymphocyte-HEV interactions in TdLN. (A) Scheme for experimental design to investigate the immunomodulatory effect of RFA in TdLN and cLN. (B) Representative photomicrographs and quantification of TRITC-labeled naïve OT-I CD8+ T cells (red) in cLN and TdLN of C57BL/6 mice bearing B16-OVA tumors after sham procedure or RFA. LN were counterstained for PNAd to identify cuboidal endothelium lining HEV (green). (C, D) In vivo short-term (1 hour, left; 12 hour, right) homing of TRITC-labeled naïve (C) or activated (D) clone 4 CD8+ T cells in TdLN and cLN from BALB/c mice bearing CT26-HA tumors after sham procedure or RFA. Data are the mean ± SEM of ≥10 fields for non-sequential cyrosections analyzed from independent mice (n = 3 mice per group) and are representative of 2 experiments. ***p < 0.001 by unpaired, two-tailed Student’s t-test. Scale bars (B–D), 100 μm.
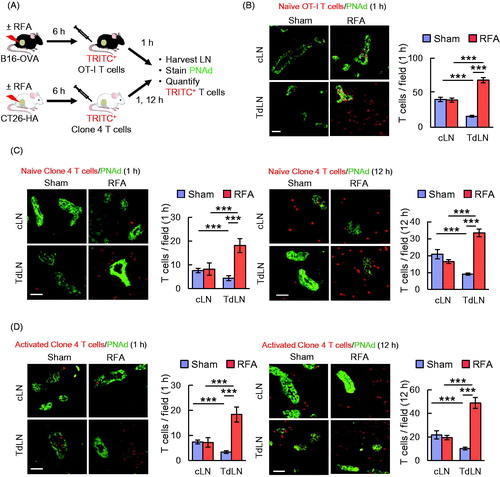
Data in indicate that baseline short-term 1 h homing of naïve T cells in untreated control mice was significantly decreased in TdLN compared to cLN. These results are in line with a prior report showing that the recruitment of naïve lymphocytes is impaired in the TdLN of C57BL/6 mice bearing B16F10 melanoma [Citation52]. We found that RFA caused approximately a six-fold increase in homing of adoptively transferred naïve OT-I CD8+ T cells selectively in TdLN but not in cLN (. Notably, the majority of transferred T cells identified in either TdLN or cLN were located outside HEV that were identified by staining cuboidal endothelial cells with Ab specific for peripheral lymph node addressin (PNAd), which is the cognate ligand for the L-selectin LN homing receptor. Thus, T cells transferred into the bloodstream gained access to antigen within the LN parenchyma within 1 h following extravasation across HEV. Similar results were observed for 1 h homing of naïve Clone 4 CD8+ T cells in CT26-HA tumor-bearing BALB/c mice in which RFA also overcame the deficit in baseline homing detected in TdLN (. Moreover, the benefit of RFA on T cell localization in TdLN was also detected at 12 h (), suggesting that overall T cell retention is improved in LN that are immediately downstream of the tumor-treatment area.
We next sought to determine if RFA enhances TdLN trafficking of a mixed population of CD8+ T cells that were activated ex vivo to generate cytotoxic T cells using clinically relevant protocols [Citation12]. Splenocytes from Clone 4 TcR-transgenic mice were stimulated with anti-CD3 antibody (Ab) and IL-2 for 5 days which results in >95% of cells with a cytotoxic effector CD8+ T cell or TCM phenotype (L-selectinloCD44hi or L-selectinhiCD44hi, respectively, as described previously [Citation12]). Activated CD8+ T cells were adoptively transferred into CT26-HA-bearing mice 6 h after RFA/sham treatment. RFA significantly increased trafficking of in vitro activated CD8+ T cells to TdLN but not cLN at both 1 h and 12 h post-adoptive transfer (. Collectively, these findings demonstrate that RFA profoundly enhances access of naïve or activated T cells to tumor antigens within TdLN in a setting of ACT.
RFA selectively increases the expression of CCL21 and ICAM-1 on HEV in TdLN
To gain mechanistic insight into the impact of RFA on the increased entry of lymphocytes into TdLN, we examined the expression of the three prototypical trafficking molecules expressed in LN HEV that mediate the multistep adhesion cascade that directs lymphocyte extravasation into nodal tissues. Specifically, we quantified vascular staining for PNAd, which is obligatory for L-selectin-dependent tethering and rolling interactions with HEV; CCL21, the homeostatic chemokine that initiates the transition between rolling interactions to firm arrest; and ICAM-1, which stabilizes β2 integrin-dependent firm arrest of lymphocytes and guides transendothelial migration [Citation53–57]. PNAd and CCL21 are expressed exclusively by cuboidal endothelial cells lining HEV [Citation54]; thus, we identified individual HEV based on PNAd+ staining.
For these studies, we harvested LN 6 h after RFA versus sham treatment and evaluated the intravascular expression of PNAd, CCL21, and ICAM-1 in cLN and TdLN from BALB/c mice bearing CT26 tumors. Quantitative measurement based on mean fluorescence intensity (MFI) for staining within PNAd+ HEV showed that the overall expression of PNAd is unaltered by RFA treatment, as indicated by the representative images shown in and example histograms shown in i.e. numbers in images and histograms indicate MFI for pixels within PNAd+ HEV for ≥10 fields of non-sequential cryosections from individual mice). Further analysis revealed that baseline intravascular expression of CCL21 in sham-treated CT26 tumor-bearing BALB/c mice was lower in TdLN HEV compared to cLN (). These findings are consistent with previous work performed in the B16F10 model in which low CCL21 expression in HEV accounted for poor homing of naïve T cells in TdLN [Citation52]. Despite this deficit, RFA substantially increased the intravascular presentation of CCL21 selectively in TdLN that drain the site of RFA treatment ().
Figure 2. RFA selectively increases the expression of CCL21 and ICAM-1 on HEV in TdLN. (A) Intravascular staining of CCL21 in cLN and TdLN by iv injection of primary Ab at 6 hours after RFA or sham procedure in CT26-bearing BALB/c mice. Organs obtained 20 min later were counterstained with fluorochrome-conjugated secondary Ab to detect anti-CCL21 Ab (red) and with anti-PNAd Ab (green) to demark the position of HEV. (B) Representative histograms for experiment shown in (A). Histograms denote quantitative image analysis of the mean fluorescence intensity (MFI) for fluorescence of PNAd (from tissue section staining) and CCL21 (from intravascular staining) in PNAd+ cuboidal LN HEV. Horizontal axes, fluorescence intensity; vertical axes, pixels with each intensity; numbers in plots, MFI. (C) Intravascular staining for ICAM-1 (red) in cLN and TdLN of CT26-bearing mice at 6 hours following sham or RFA treatment. LN were counterstained with anti-PNAd Ab (green) to identify HEV. (A–C) Scale bars, 100 μm; numbers in photomicrographs and histograms indicate MFI for quantification of all pixels analyzed within the HEV for ≥10 fields in non-sequential cryosections for individual mice. Data are representative of 2 independent experiments.
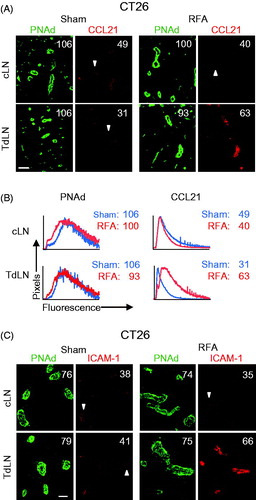
Baseline intravascular ICAM-1 expression was equivalent in TdLN and cLN of CT26-bearing mice whereas ICAM-1 was preferentially increased by RFA treatment in TdLN but not cLN HEV (. We further examined the kinetics of induction of intravascular ICAM-1 by RFA in HEV using B16F10-bearing C57BL/6 mice. Data shown in indicate that intravascular expression of ICAM-1 was maximally enhanced in TdLN HEV within 6 h after RFA treatment while intravascular ICAM-1 expression declined on HEV at 12 and 24 h after treatment. These results suggest there is a finite window to maximize trafficking of adoptively transferred T cells in HEV post-RFA.
Figure 3. RFA increases homing of lymphocytes to TdLN by enhancing secondary firm adhesion in HEV. (A) Kinetics of intravascular ICAM-1 induction by RFA in cLN and TdLN in C57BL/6 mice bearing B16F10 tumors. The position of HEV is demarked by PNAd staining of LN. Numbers indicate MFI for total pixels analyzed within area designated as HEV (i.e. ≥10 fields for non-sequential cryosections were analyzed per treatment group). Data are representative of 2 independent experiments. Scale bars, 100 μm. (B,C) Calcein-labeled lymphocytes were adoptively transferred into recipient C57BL/6 mice bearing B16-OVA tumors at 6 hours after sham or RFA treatment and their interactions within the LN venular tree were analyzed by IVM. Mean rolling fractions (B) and sticking fractions (C) ± SEM in TdLN are shown. Data are from three independent experiments (n = 3 mice/group). *p < 0.05 and **p < 0.01 by unpaired, two-tailed Student’s t-test.
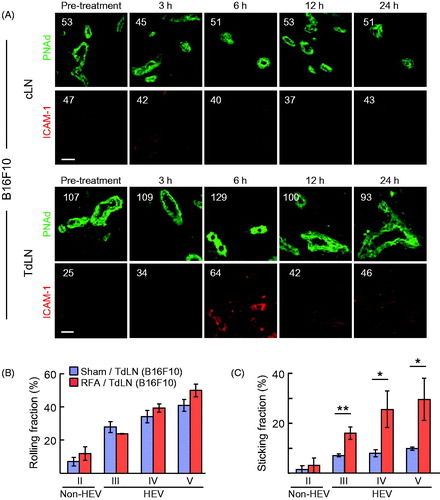
These observations prompted us to use IVM to pinpoint the nature of the adhesive interactions regulated by RFA that result in increased CD8+ T cell trafficking at the lumenal surface of HEV in TdLN. The anatomy of the vascular tree is organized into vessels of differing order such that capillaries collect into high order venules, which subsequently drain into low order venules and collecting veins [Citation15,Citation54]. In LN, high-order (III–V) postcapillary venules constitute HEV that are typified by a cuboidal endothelial morphology, expression of PNAd and CCL21, and high constitutive rates of lymphocyte trafficking [Citation15,Citation54]. Conversely, low-order venules (II) are comprised of flat-walled non-HEV vessels that lack PNAd and CCL21 and do not support lymphocyte trafficking [Citation15,Citation50]. Consistent with evidence that RFA did not induce PNAd expression in cuboidal HEV (), we found that RFA did not affect the frequency of rolling interactions throughout the venular tree (. In contrast, RFA significantly increased the fraction of cells that transitioned from primary rolling to secondary firm sticking exclusively in order III–V venules (i.e., HEV) without altering lymphocyte adhesion in order II venules (i.e., non-HEV, . Altogether, these data support a model in which increased T cell homing to TdLN after RFA is mediated by enhanced secondary firm adhesive interactions as a result of selective induction of CCL21 and ICAM-1 on the lumenal surface of HEV.
Temporal coordination of intravascular ICAM-1 induction and trafficking of adoptively transferred antigen-specific effector T cells in response to RFA in the tumor microenvironment
We previously reported that RFA increases the accumulation of CD8+ cytotoxic T cells in the tumor microenvironment 7 days after treatment [Citation32]. However, it remains unknown whether this increase in CD8+ T cell infiltration by in situ thermal ablation therapy involves rapid stimulation of T cell trafficking to the tumor microenvironment or if it is solely due to late events that occur over days that stem from antigen-driven activation and expansion of T cells in TdLN and their subsequent migration to tumors. To address this question, we examined the relative intravascular density of ICAM-1 on tumor vessels identified by expression of the pan-endothelial adhesion molecule, CD31, in tumor tissue at 6 h after RFA treatment. We found that RFA substantially increased the intravascular expression of ICAM-1 in CD31+ vessels of both CT26-HA and B16-OVA tumors when compared to sham-treated tumors (. In contrast, the density of CD31 was unchanged on tumor vascular endothelium in both tumor models.
Figure 4. RFA augments ICAM-1 and trafficking of adoptively transferred in vitro-activated CD8+ T cells in tumor vessels. (A, B) Intravascular staining of ICAM-1 in CT26-HA tumor (A) or B16-OVA tumor (B). Tumor-bearing mice were injected iv with ICAM-1 Ab 6 hours after RFA or sham treatment. Twenty minutes after injection, tumors were harvested and cryosections were counterstained with TRITC-conjugated secondary Ab (red) and anti-CD31 Ab (green) to identify vessel position. Numbers indicate MFI determined by quantification of all pixels analyzed within CD31+ vessels in ≥10 fields of non-sequential cryosections for each treatment group. (C) Schematic illustration investigating immunomodulatory effect of RFA in BALB/c mice bearing CT26-HA tumors. Representative photomicrographs and quantification of TRITC-labeled activated Clone 4 splenocytes (red) in the indicated organs (CT26-HA tumor, pancreas, and spleen) counterstained for CD31+ vessels (green) 1 hour or 12 hours after sham procedure or RFA. (D) Schematic of experimental design to investigate leakage of cells after RFA in BALB/c mice bearing CT26-HA tumor. Representative photomicrographs and quantification of TRITC-labeled RBC (red) in CT26-HA tumors or TdLN 2 hours after sham procedure or RFA; tumor tissues counterstained for CD31+ vessels and LN were stained for PNAd+ HEV (green). ***p < 0.001; ns: not significant as determined by unpaired, two-tailed Student’s t-test. Scale bars, 100 μm; data are mean ± SEM of ≥10 fields analyzed from independent mice (n = 3 mice per group) and are representative of 2 experiments.
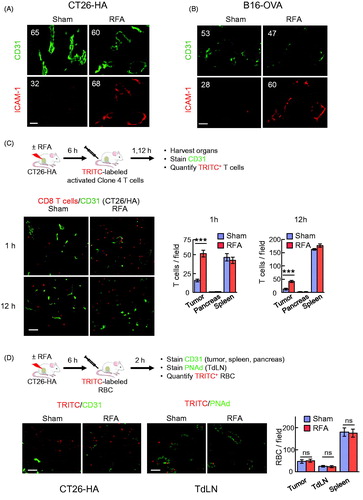
Strong induction of intravascular ICAM-1 in tumor vessels after RFA prompted us to hypothesize that trafficking of adoptively transferred CD8+ T cells to the tumor site would also be enhanced by RFA. To this end, in vitro activated Clone 4 CD8+ T cells were labeled with fluorescent tracking dye prior to iv adoptive transfer into sham- or RFA-treated CT26-HA tumor-bearing mice and tumors were harvested 1 h or 12 h after adoptive transfer (. We detected a significant increase in intratumoral CD8+ T cell infiltration as early as 1 h after RFA. Moreover, elevated numbers of Clone 4 T cells were maintained in the tumor for at least 12 h. In sharp contrast, CD8+ T cell infiltration into healthy organs outside the treatment field (i.e., pancreas and spleen) was unchanged following RFA treatment ( and Supplemental Figure 1), suggesting that RFA exerts local/regional and not systemic effects on T cell homing.
We further considered whether improved trafficking of adoptively transferred T cells in response to RFA could be due to passive leakage across disrupted tumor vasculature rather than active trafficking/infiltration. We formally addressed this possibility by iv adoptive transfer of fluorescence-tagged RBC that lack trafficking proteins necessary for active homing to tissues into CT26-HA tumor-bearing mice (. We then assessed the frequency of RBC in TdLN and tumors at 2 h post-adoptive transfer to allow sufficient time to detect passive leakage in these tissues without overloading the spleen which was used as control tissue outside the RFA treatment region. As expected, we found that tumor vessels were more leaky than LN vessels in treated and sham-treated mice as indicated by higher numbers of blood-borne fluorescent tagged RBC that reached the tumor interstitium (i.e., located outside CD31+ vessels). However, RFA did not alter the frequency of transferred RBC recovered in tumor, TdLN, or spleen (. Thus, the enhanced T cell infiltration observed in tumors or LN in response to RFA cannot be explained by passive leakage of T cells across damaged blood vessels.
RFA augments the therapeutic efficacy of adoptive CD8+ T cell therapy
We next examined whether early trafficking of adoptively transferred T cells in response to RFA was associated with enhanced killing of target tumor cells in established tumors and whether T cell-mediated cytolysis was antigen-restricted. For these studies, we quantified TUNEL+ apoptotic cells in B16-OVA versus parental B16F10 tumors after adoptive transfer of in vitro activated OVA-specific TcR-transgenic OT-I CD8+ T cells using approaches we previously described [Citation12,Citation18]. OT-I T cells were transferred 6 h after RFA treatment to exploit the window of ICAM-1 induction in tumor vessels and tumor-infiltrating OT-I T cells and intratumoral apoptotic cells were quantified 12 h later (). RFA alone had a relatively modest impact on apoptosis of tumor cells in the local microenvironment regardless of expression of surrogate tumor antigen by tumor cells (. Moreover, the low level of OT-I infiltration detected post-ACT in sham-treated B16-OVA tumors was associated with minimal apoptosis (). These data are consistent with a previous report that entry of OT-I T cells is limited in B16-OVA melanoma after ACT [Citation12]. In contrast, combined RFA and OT-I T cell ACT significantly increased the extent of apoptosis detected in B16-OVA tumors within this short window (i.e., 18 h) after RFA treatment (. Tumor cell apoptosis induced by RFA preconditioning and OT-I T cell ACT reflected antigen-specific cytolysis as evidenced by findings that OT-I ACT had no benefit over RFA alone in parental B16F10 tumors that lack OVA antigen (. Increased OT-I T cell accumulation detected in B16-OVA compared to B16F10 tumors 12 h after adoptive transfer is consistent with the active role of cognate antigen recognition in retention of T cells within tumors, as described in a prior report [Citation12].
Figure 5. RFA augments antigen-restricted killing of tumor targets by adoptively transferred CD8+ T cells. (A) Schematic for experimental design to investigate RFA augmentation of antigen-directed killing and representative photomicrographs of B16-OVA tumors at 12 hours after ACT using TRITC-labeled in vitro-activated OT-I cells (red). Apoptotic cells were detected by TUNEL assay (green) and nucleated cells were stained with DAPI (blue). Scale bar: 100 μm. (B, C) Quantification of adoptively transferred TRITC-labeled in vitro-activated OT-I cells (B) and TUNEL+ apoptotic cells (C) in B16-OVA and parental B16F10 tumors. Data are mean ± SEM of 5–10 fields analyzed from non-sequential cryosections of independent mice (n = 3 mice per group) and are representative of 2 experiments. ***p < 0.001 and **p < 0.01 by unpaired two-tailed Student’s t-test.
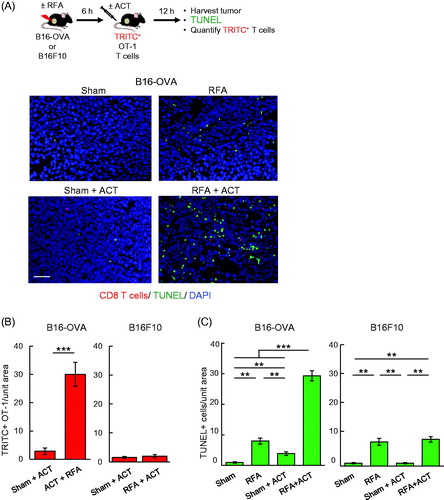
Evidence that RFA augments the antitumor activity of cytotoxic T cells during ACT prompted us to test whether RFA improves the efficacy of therapeutic T cell ACT in both the B16-OVA and CT26-HA tumor models. Mice bearing B16-OVA or CT26-HA tumors were treated with sham procedure or RFA followed 6 h later by adoptive transfer of in vitro activated OVA-specific OT-I CD8+ T cells or HA-specific Clone 4 CD8+ T cells. Treatment with either RFA or ACT alone modestly delayed the growth of B16-OVA or CT26-HA tumors compared to the sham-treatment group ( and Supplemental Tables 1 and 2). However, combined RFA and ACT substantially delayed tumor growth and prolonged survival in both the B16-OVA and CT26-HA models. A secondary objective was to confirm that the improved efficacy of RFA depended on antigen-specific recognition of tumors by transferred T cell therapeutics. This was accomplished by adoptively transferring OT-I CD8+ T cells into mice bearing parental B16 tumors ( and Supplemental Table 1). These data showed that the combination of RFA and ACT had no benefit over RFA alone, establishing that the tumor growth control and improved survival observed during combination therapy in the B16-OVA model was antigen-restricted. Pilot studies further suggested that combined RFA and T cell ACT induces long-term T cell memory based on findings that tumor rejection was observed in 100% of RFA and ACT-treated B16-OVA mice when rechallenged at 150 days with B16-OVA whereas 0% rejection was observed in mice rechallenged with parental B16F10 tumor (n = 4 mice per group, data not shown).
Figure 6. In situ immune modulation with RFA augments antitumor efficacy of adoptively transferred CD8+ T cells. Tumor growth curves and survival curves for mice bearing B16-OVA and parental B16 tumors (A) or CT26-HA tumors (B) in different treatment groups (n = 5 mice per group). Tumor-bearing mice were treated with sham procedure or RFA. Six hours after the procedure, ACT was administered by iv transfer of 1 × 106 in vitro-activated OT-I (A) or Clone 4 (B) CD8+ T cells. (A, B) For survival data, **p < 0.01 for RFA + ACT group compared to all other groups as determined using log-rank (Mantel–Cox) test.
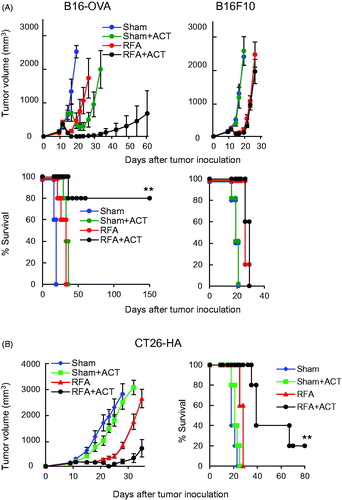
Discussion
Thermal ablative techniques such as RFA and cryoablation are minimally invasive therapeutic modalities for the local destruction of primary and metastatic tumors that are performed with an image guided-percutaneous approach under local anesthesia. While these techniques are best suited for patients who are not candidates for surgical resection, a key distinction from surgical resection is that ablation causes tumor cell death that remains in situ, in what is essentially a self-contained vaccine that leads to exposure of hidden antigenic epitopes to the immune system that trigger systemic antitumor immune responses [Citation24,Citation25,Citation33,Citation34,Citation37]. Furthermore, these endogenous antitumor immune responses can be augmented by various immunomodulatory regimens such as adoptive transfer of tumor-specific CAR T cells or TcR-transgenic T cells or immune checkpoint inhibitor therapy directed against CTLA-4 and the PD-1/PD-L1 axis [Citation25,Citation34,Citation35]. The broad immunomodulatory activities of RFA prompted us to investigate the therapeutic benefit of combining RFA with CD8+ T cell ACT in the current study. ACT is attractive, in this regard, since it provides an opportunity to massively boost the frequency of tumor-reactive T cells by bolus infusion at the same time that tumor antigens are being released from the tumor mass via ablation. Here, we provide the first evidence that RFA enhances the efficacy of CD8+ T cell ACT in two preclinical murine cancer models. The therapeutic benefit of RFA in conjunction with ACT is further correlated with improved early access of CD8+ T cells to tumor antigens at two anatomically distinct sites; i.e., TdLN and the tumor microenvironment.
Our study expands on the immunoregulatory mechanisms known to underlie RFA activation of T cell immunity by establishing that homing of CD8+ T cells is improved in TdLN in direct response to in situ thermal ablation. These findings support the premise that RFA creates a more favorable immune microenvironment in TdLN which can otherwise be dominated by tolerogenic DC and immunosuppressive regulatory T cells as previously documented in the B16F10 model [Citation58]. Ablation-induced immunogenic death of tumor cells releases tumor antigens as well as proinflammatory mediators such as the heat shock proteins Hsp70 and gp96 [Citation41,Citation59,Citation60], which in turn stimulate migration of antigen-loaded DC into TdLN via the afferent lymphatics [Citation25,Citation28,Citation43,Citation61,Citation62]. RFA-induced antigen cross-priming of CD8+ T cells by DC is accompanied by substantial expansion of tumor-reactive CD8+ T cells in TdLN [Citation32,Citation34,Citation36]. Improved T cell homing via gatekeeper HEV within 6 h of RFA administration coincides with these early immune-activating events of RFA. Increased localization of tumor-reactive naïve or memory CD8+ T cells (i.e., TCM and TSCM) in LN effectively increases the probability that individual T cells will encounter DC presenting cognate tumor antigen. Our findings that CD8+ T cells remain elevated in TdLN 12 h after RFA is consistent with the time-frame documented for initial activation of T cells by antigen-loaded DC in LN in a non-cancerous setting [Citation63]. This timing is also relevant to observations that the first 12 h of antigen exposure are the most critical for generating intranodal T cell immunity [Citation64].
Complementary findings showed that RFA also improves direct access of tumor-specific cytotoxic CD8+ T cells to the viable non-ablated zone within the tumor microenvironment during ACT in both B16F10 and CT26 models. Improved homing provides an explanation for the increased infiltration of endogenous T cells in ablated tumor tissue that has been reported in preclinical models and in patients [Citation26,Citation27,Citation32]. An immediate consequence of RFA-boosted homing is the increased apoptosis of target tumor cells by antigen-restricted CD8+ T cell therapeutics that occurs within 12 h of ACT. This initial killing event is likely due to direct homing of adoptively transferred CD8+ effector T cells to tumor sites to the tumor microenvironment since it takes ≥3 days for naïve or memory T cells to undergo priming and activation in TdLN prior to their trafficking from TdLN to tumor destinations.
Multiple lines of evidence definitively establish that RFA improves T cell homing by modifying the vascular function in TdLN and tumor sites. Firstly, we found that RFA increases the intravascular density of the β2 integrin ligand, ICAM-1, in tumor vessels as well as TdLN HEV that are the major portals for extravasation of blood-borne cytotoxic effector T cells to tumor sites or naive, TSCM, and TCM cells to LN. Of note, we found baseline T cell homing to be reduced in TdLN compared to cLN in both B16F10 and CT26 tumor systems. These data confirm and extend prior results in the B16F10 model in which poor T cell homing in TdLN was attributed to tumor-induced reduction in CCL21 presentation on TdLN HEV [Citation52]. Results of the current study suggest that RFA overrides this homing deficit by increasing the intravascular presentation of CCL21 and ICAM-1 on the lumenal surface of HEV.
Secondly, we demonstrated that the RFA-induced increase in CD8+ T cell homing in TdLN and tumor sites was detectable within 1 h after adoptive transfer of T cells. Thus, improved trafficking is attributed to vascular events since 1 h is sufficient for blood-borne T cells to complete extravasation across vascular barriers, but not enough time for other processes to occur that also influence the extent of tissue infiltration such as retention, proliferation and egress. T cell homing at vascular surfaces generally does not depend on antigen; however, in the absence of cognate antigen recognition T cells exit from LN and extralymphoid tissues after ∼12–15 h [Citation12,Citation65]. Consistent with this paradigm, our data in the current study and a prior report showed diminished infiltration OT-I T cells at 12 h after ACT within B16F10 tumors that do not express OVA antigen [Citation12].
Thirdly, we obtained direct evidence for enhanced vascular function in IVM studies showing that RFA substantially increased firm arrest of lymphocytes selectively in HEV without altering their rolling frequency. These data are consistent with the observed selective induction by RFA of CCL21 and ICAM-1 molecules that mediate the transition of T cells from primary rolling interactions to secondary arrest in HEV whereas expression of PNAd is unchanged in HEV [Citation53,Citation54]. Findings for high-temperature RFA parallel reports that fever-range (38–40 °C) systemic thermal therapy augments ICAM-1 or CCL21-dependent T cell trafficking in tumor vessels and LN [Citation12,Citation15,Citation18,Citation44,Citation45,Citation48,Citation66,Citation67]. However, a key distinction is that while fever-range systemic thermal therapy augments T cell homing in all LN located throughout the body [Citation15,Citation44,Citation45,Citation48,Citation66,Citation67], the impact of RFA is focal, influencing ICAM-1 and CCL21-dependent homing solely in TdLN. This would be expected to be an advantage during ACT since it maximizes the chances that therapeutic CD8+ T cell products will encounter tumor antigens that are enriched within the parenchyma of TdLN but not non-draining LN. It is envisioned that local ablation could be delivered in a minimally invasive fashion to augment site-specific induction of trafficking molecules and homing of infused antigen-specific T cells during clinical ACT.
The local nature of the vascular response in TdLN and tumor vessels is in line with a scenario in which soluble factors act on vessels within the tumor milieu or are delivered to TdLN HEV via afferent lymphatic vessels. It is tempting to speculate that inflammatory cytokines are direct mediators of the vascular effects of RFA. Indeed, catastrophic cell damage induced by ablation is characterized by local upregulation of multiple inflammatory cytokines including tumor necrosis factor (TNF), IL-1, and IL-6, together with activation of the downstream NFκB transcription factor [Citation68–70]. These inflammatory mediators are well-known stimulators of ICAM-1 synthesis in endothelial cells [Citation71,Citation72]. Moreover, local IL-6 is necessary and sufficient for ICAM-1 induction in LN HEV and tumor vessels in response to fever-range systemic thermal therapy [Citation12,Citation15,Citation67]. The failure to broadly detect T cell homing or ICAM-1 changes in tissues outside the treatment field (i.e., cLN, pancreas) suggests that systemic cytokines are unlikely to be involved even though circulating levels of TNF, IL-1, and IL-6 are reportedly elevated in rodent cancer models and cancer patients following RFA [Citation70,Citation73–76]. Given the complex inflammatory nature of RFA, it will be of interest in future studies to determine if the observed vascular response involves a cocktail of pro-adhesive cytokines or if a single cytokine is responsible as previously shown for the non-redundant role of IL-6 during fever-range systemic thermal therapy [Citation12,Citation15,Citation67].
The current study demonstrates that acute inflammatory cues triggered by RFA combined with CD8+ T cell ACT results in superior in vivo antitumor efficacy compared to either single modality, as indicated by improved tumor growth delay and survival in B16F10 melanoma and CT26 colon adenocarcinoma models. Observations for long-term tumor control extending up to 150 days post-implantation of highly metastatic OVA-expressing B16F10 but not parental B16F10 highlight the durability of the response that is mediated by adoptively transferred OVA-specific CD8+ T cells. These results point to the requirement for systemic immune responses activated in TdLN in the context of combined RFA and ACT. Systemic CD8+ T cell immunity has also been implicated in the abscopal effects previously attributed to RFA during tumor rechallenge at distant sites outside the ablation zone in the CT26 tumor model [Citation32]. The impact of RFA on the persistence of adoptively transferred T cells in peripheral blood remains to be evaluated and could be an important determinant of systemic antitumor immunity, given evidence in patients that maintenance of the circulating pool of transferred T cell therapeutics correlates with tumor regression [Citation77–82].
Findings that RFA rapidly improves CD8+ T cell homing in TdLN are particularly notable given the emergence of the systemic immune system as a critical component for long-term tumor control during T cell-based immunotherapy. In this regard, preclinical studies in B16F10 melanoma showed that interference with L-selectin-dependent homing of adoptively transferred naïve, TCM or TSCM T cell therapeutics to LN substantially reduces the efficacy of ACT [Citation19,Citation20]. Pharmacologic blockade of CD8+ T cell egress from lymphoid organs including LN by disrupting sphingosine-1-phosphate receptor 1 signaling using FTY720 similarly lowers therapeutic response to tumor vaccines, immune checkpoint inhibitors or ACT in murine MC38 colon adenocarcinoma and 4T1 mammary cancer models [Citation21]. Thus, continual maintenance of CD8+ T cell antitumor immunity in secondary lymphoid organs appears to be critical for immunosurveillance to limit outgrowth of newly arising tumors. Results that ICAM-1 induction on vessels is maximal at 6 h post-RFA in TdLN suggests there is a finite window in which to exploit the pro-migratory effects of ablative therapy during ACT. Our present findings provide a rationale for further investigation to formally test if administration of ACT within this window provides for optimal antitumor control, as predicted based on the kinetics of vascular responses to RFA.
In conclusion, our findings indicate that RFA dually enhances expression of homing molecules on TdLN HEV as well as in tumor vessels. This vascular response overcomes limitations in CD8+ T-cell trafficking across tumor vessels and HEV in TdLN, thus significantly improving the antitumor efficacy of ACT. Our results shed light on the acute immunomodulatory effects of RFA which can be exploited to develop more effective immunotherapy.
Supplemental Material
Download PDF (144.5 KB)Disclosure statement
No potential conflict of interest was reported by the authors.
Additional information
Funding
References
- Kochenderfer JN, Wilson WH, Janik JE, et al. Eradication of B-lineage cells and regression of lymphoma in a patient treated with autologous T cells genetically engineered to recognize CD19. Blood. 2010;116:4099–4102.
- Maus MV, Fraietta JA, Levine BL, et al. Adoptive immunotherapy for cancer or viruses. Annu Rev Immunol. 2014;32:189–225.
- Porter DL, Levine BL, Kalos M, et al. Chimeric antigen receptor-modified T cells in chronic lymphoid leukemia. N Engl J Med. 2011;365:725–733.
- Grupp SA, Kalos M, Barrett D, et al. Chimeric antigen receptor-modified T cells for acute lymphoid leukemia. N Engl J Med. 2013;368:1509–1518.
- Maude SL, Frey N, Shaw PA, et al. Chimeric antigen receptor T cells for sustained remissions in leukemia. N Engl J Med. 2014;371:1507–1517.
- Morgan RA, Dudley ME, Wunderlich JR, et al. Cancer regression in patients after transfer of genetically engineered lymphocytes. Science. 2006;314:126–129.
- Rapoport AP, Stadtmauer EA, Binder-Scholl GK, et al. NY-ESO-1-specific TCR-engineered T cells mediate sustained antigen-specific antitumor effects in myeloma. Nat Med. 2015;21:914–921.
- Robbins PF, Morgan RA, Feldman SA, et al. Tumor regression in patients with metastatic synovial cell sarcoma and melanoma using genetically engineered lymphocytes reactive with NY-ESO-1. JCO. 2011;29:917–924.
- Robbins PF, Kassim SH, Tran TL, et al. A pilot trial using lymphocytes genetically engineered with an NY-ESO-1-reactive T-cell receptor: long-term follow-up and correlates with response. Clin Cancer Res. 2015;21:1019–1027.
- Rosenberg SA, Yang JC, Sherry RM, et al. Durable complete responses in heavily pretreated patients with metastatic melanoma using T-cell transfer immunotherapy. Clin Cancer Res. 2011;17:4550–4557.
- Rosenberg SA, Packard BS, Aebersold PM, et al. Use of tumor-infiltrating lymphocytes and interleukin-2 in the immunotherapy of patients with metastatic melanoma. A preliminary report. N Engl J Med. 1988;319:1676–1680.
- Fisher DT, Chen Q, Skitzki JJ, et al. IL-6 trans-signaling licenses mouse and human tumor microvascular gateways for trafficking of cytotoxic T cells. J Clin Invest. 2011;121:3846–3859.
- Joyce JA, Fearon DT. T cell exclusion, immune privilege, and the tumor microenvironment. Science. 2015;348:74–80.
- Pockaj BA, Sherry RM, Wei JP, et al. Localization of 111indium-labeled tumor infiltrating lymphocytes to tumor in patients receiving adoptive immunotherapy. Augmentation with cyclophosphamide and correlation with response. Cancer. 1994;73:1731–1737.
- Chen Q, Fisher DT, Clancy KA, et al. Fever-range thermal stress promotes lymphocyte trafficking across high endothelial venules via an interleukin 6 trans-signaling mechanism. Nat Immunol. 2006;7:1299–1308.
- Chung AS, Lee J, Ferrara N. Targeting the tumour vasculature: insights from physiological angiogenesis. Nat Rev Cancer. 2010;10:505–514.
- Slaney CY, Kershaw MH, Darcy PK. Trafficking of T Cells into Tumors. Cancer Res. 2014;74:7168–7174.
- Mikucki ME, Fisher DT, Matsuzaki J, et al. Non-redundant requirement for CXCR3 signalling during tumoricidal T-cell trafficking across tumour vascular checkpoints. Nat Commun. 2015;6:7458.
- Gattinoni L, Klebanoff CA, Palmer DC, et al. Acquisition of full effector function in vitro paradoxically impairs the in vivo antitumor efficacy of adoptively transferred CD8+ T cells. J Clin Invest. 2005;115:1616–1626.
- Gattinoni L, Zhong XS, Palmer DC, et al. Wnt signaling arrests effector T cell differentiation and generates CD8+ memory stem cells. Nat Med. 2009;15:808–813.
- Spitzer MH, Carmi Y, Reticker-Flynn NE, et al. Systemic immunity is required for effective cancer immunotherapy. Cell. 2017;168:487–502.e15.
- Wong SL, Mangu PB, Choti MA, et al. American Society of Clinical Oncology 2009 clinical evidence review on radiofrequency ablation of hepatic metastases from colorectal cancer. JCO. 2010;28:493–508.
- Ginzburg S, Tomaszewski JJ, Kutikov A. Focal ablation therapy for renal cancer in the era of active surveillance and minimally invasive partial nephrectomy. Nat Rev Urol. 2017;14:669–682.
- Chu KF, Dupuy DE. Thermal ablation of tumours: biological mechanisms and advances in therapy. Nat Rev Cancer. 2014;14:199–208.
- den Brok MH, Sutmuller RP, van der Voort R, et al. In situ tumor ablation creates an antigen source for the generation of antitumor immunity. Cancer Res. 2004;64:4024–4029.
- Wissniowski TT, Hansler J, Neureiter D, et al. Activation of tumor-specific T lymphocytes by radio-frequency ablation of the VX2 hepatoma in rabbits. Cancer research. 2003;63:6496–6500.
- Zerbini A, Pilli M, Penna A, et al. Radiofrequency thermal ablation of hepatocellular carcinoma liver nodules can activate and enhance tumor-specific T-cell responses. Cancer Res. 2006;66:1139–1146.
- Ali MY, Grimm CF, Ritter M, et al. Activation of dendritic cells by local ablation of hepatocellular carcinoma. J Hepatol. 2005;43:817–822.
- Schueller G, Kettenbach J, Sedivy R, et al. Heat shock protein expression induced by percutaneous radiofrequency ablation of hepatocellular carcinoma in vivo. Int J Oncol. 2004;24:609–613.
- Napoletano C, Taurino F, Biffoni M, et al. RFA strongly modulates the immune system and anti-tumor immune responses in metastatic liver patients. Int J Oncol. 2008;32:481–490.
- Fietta AM, Morosini M, Passadore I, et al. Systemic inflammatory response and downmodulation of peripheral CD25 + Foxp3+ T-regulatory cells in patients undergoing radiofrequency thermal ablation for lung cancer. Human immunology. 2009;70:477–486.
- Ito F, Ku AW, Bucsek MJ, et al. Immune adjuvant activity of pre-resectional radiofrequency ablation protects against local and systemic recurrence in aggressive murine colorectal cancer. PLOS One. 2015;10:e0143370.
- Dromi SA, Walsh MP, Herby S, et al. Radiofrequency ablation induces antigen-presenting cell infiltration and amplification of weak tumor-induced immunity. Radiology. 2009;251:58–66.
- den Brok MH, Sutmuller RP, Nierkens S, et al. Efficient loading of dendritic cells following cryo and radiofrequency ablation in combination with immune modulation induces anti-tumour immunity. Br J Cancer. 2006;95:896–905.
- Shi L, Chen L, Wu C, et al. PD-1 blockade boosts radiofrequency ablation-elicited adaptive immune responses against tumor. Clin Cancer Res. 2016;22:1173–1184.
- den Brok MH, Sutmuller RP, Nierkens S, et al. Synergy between in situ cryoablation and TLR9 stimulation results in a highly effective in vivo dendritic cell vaccine. Cancer Res. 2006;66:7285–7292.
- Nierkens S, den Brok MH, Ruers TJ, et al. Radiofrequency ablation in cancer therapy: tuning into in situ tumor vaccines. Volume 5. In: Keisari Y, editor. Tumor ablation. Springer; 2013. p. 39–60.
- Gameiro SR, Higgins JP, Dreher MR, et al. Combination therapy with local radiofrequency ablation and systemic vaccine enhances antitumor immunity and mediates local and distal tumor regression. PLOS One. 2013;8:e70417.
- Johnson EE, Yamane BH, Buhtoiarov IN, et al. Radiofrequency ablation combined with KS-IL2 immunocytokine (EMD 273066) results in an enhanced antitumor effect against murine colon adenocarcinoma. Clin Cancer Res. 2009;15:4875–4884.
- Thakur A, Littrup P, Paul EN, et al. Induction of specific cellular and humoral responses against renal cell carcinoma after combination therapy with cryoablation and granulocyte-macrophage colony stimulating factor: a pilot study. J Immunother. 2011;34:457–467.
- Liu Q, Zhai B, Yang W, et al. Abrogation of local cancer recurrence after radiofrequency ablation by dendritic cell-based hyperthermic tumor vaccine. Mol Ther. 2009;17:2049–2057.
- Waitz R, Solomon SB, Petre EN, et al. Potent induction of tumor immunity by combining tumor cryoablation with anti-CTLA-4 therapy. Cancer Res. 2012;72:430–439.
- Saji H, Kato H, Song W, et al. Therapeutic vaccination against murine colon carcinoma by radiofrequency ablation in combination with intratumoral naive dendritic cell injection. Cancer Res. 2007;67:1852–1852.
- Ku AW, Muhitch JB, Powers CA, et al. Tumor-induced MDSC act via remote control to inhibit L-selectin-dependent adaptive immunity in lymph nodes. eLife. 2016;5:e17375.
- Chen Q, Appenheimer MM, Muhitch JB, et al. Thermal facilitation of lymphocyte trafficking involves temporal induction of intravascular ICAM-1. Microcirculation. 2009;16:143–158.
- Weninger W, Crowley MA, Manjunath N, et al. Migratory properties of naive, effector, and memory CD8(+) T cells. J Exp Med. 2001;194:953–966.
- Chen Q, Fisher DT, Kucinska SA, et al. Dynamic control of lymphocyte trafficking by fever-range thermal stress. Cancer Immunol Immunother. 2006;55:299–311.
- Evans SS, Wang WC, Bain MD, et al. Fever-range hyperthermia dynamically regulates lymphocyte delivery to high endothelial venules. Blood. 2001;97:2727–2733.
- Abramoff MD, Magelhaes PJ, Ram SJ. Image processing with ImageJ. Biophotonics Int. 2004;11:36–42.
- von Andrian UH. Intravital microscopy of the peripheral lymph node microcirculation in mice. Microcirculation. 1996;3:287–300.
- Mandl JN, Liou R, Klauschen F, et al. Quantification of lymph node transit times reveals differences in antigen surveillance strategies of naive CD4+ and CD8+ T cells. Proc Natl Acad Sci USA. 2012;109:18036–18041.
- Carriere V, Colisson R, Jiguet-Jiglaire C, et al. Cancer cells regulate lymphocyte recruitment and leukocyte-endothelium interactions in the tumor-draining lymph node. Cancer Res. 2005;65:11639–11648.
- Butcher EC, Picker LJ. Lymphocyte homing and homeostasis. Science. 1996;272:60–66.
- von Andrian UH, Mempel TR. Homing and cellular traffic in lymph nodes. Nat Rev Immunol. 2003;3:867–878.
- Miyasaka M, Tanaka T. Lymphocyte trafficking across high endothelial venules: dogmas and enigmas. Nat Rev Immunol. 2004;4:360–370.
- Luo BH, Carman CV, Springer TA. Structural basis of integrin regulation and signaling. Annu Rev Immunol. 2007;25:619–647.
- Shamri R, Grabovsky V, Gauguet JM, et al. Lymphocyte arrest requires instantaneous induction of an extended LFA-1 conformation mediated by endothelium-bound chemokines. Nat Immunol. 2005;6:497–506.
- Sharma MD, Hou DY, Baban B, et al. Reprogrammed foxp3(+) regulatory T cells provide essential help to support cross-presentation and CD8(+) T cell priming in naive mice. Immunity. 2010;33:942–954.
- Yang WL, Nair DG, Makizumi R, et al. Heat shock protein 70 is induced in mouse human colon tumor xenografts after sublethal radiofrequency ablation. Ann Surg Oncol. 2004;11:399–406.
- Rai R, Richardson C, Flecknell P, et al. Study of apoptosis and heat shock protein (HSP) expression in hepatocytes following radiofrequency ablation (RFA). J Surg Res. 2005;129:147–151.
- Zerbini A, Pilli M, Fagnoni F, et al. Increased immunostimulatory activity conferred to antigen-presenting cells by exposure to antigen extract from hepatocellular carcinoma after radiofrequency thermal ablation. J Immunother. 2008;31:271–282.
- Blachere NE, Li Z, Chandawarkar RY, et al. Heat shock protein-peptide complexes, reconstituted in vitro, elicit peptide-specific cytotoxic T lymphocyte response and tumor immunity. J Exp Med. 1997;186:1315–1322.
- Mempel TR, Henrickson SE, Von Andrian UH. T-cell priming by dendritic cells in lymph nodes occurs in three distinct phases. Nature. 2004;427:154–159.
- Druzd D, Matveeva O, Ince L, et al. Lymphocyte circadian clocks control lymph node trafficking and adaptive immune responses. Immunity. 2017;46:120–132.
- Cyster JG, Schwab SR. Sphingosine-1-phosphate and lymphocyte egress from lymphoid organs. Annu Rev Immunol. 2012;30:69–94.
- Evans SS, Bain MD, Wang WC. Fever-range hyperthermia stimulates alpha4beta7 integrin-dependent lymphocyte-endothelial adhesion. Int J Hyperthermia. 2000;16:45–59.
- Evans SS, Repasky EA, Fisher DT. Fever and the thermal regulation of immunity: the immune system feels the heat. Nat Rev Immunol. 2015;15:335–349.
- Rozenblum N, Zeira E, Bulvik B, et al. Radiofrequency ablation: inflammatory changes in the periablative zone can induce global organ effects, including liver regeneration. Radiology. 2015;276:416–425.
- Nomoto Y, Yamamoto M, Fukushima T, et al. Expression of nuclear factor kappa B and tumor necrosis factor alpha in the mouse brain after experimental thermal ablation injury. Neurosurgery. 2001;48:158–166.
- Ahmed M, Kumar G, Navarro G, et al. Systemic siRNA nanoparticle-based drugs combined with radiofrequency ablation for cancer therapy. PLOS One. 2015;10:e0128910.
- Collins T, Read MA, Neish AS, et al. Transcriptional regulation of endothelial cell adhesion molecules: NF-kappa B and cytokine-inducible enhancers. FASEBJ. 1995;9:899–909.
- Ledebur HC, Parks TP. Transcriptional regulation of the intercellular adhesion molecule-1 gene by inflammatory cytokines in human endothelial cells. Essential roles of a variant NF-kappa B site and p65 homodimers. J Biol Chem. 1995;270:933–943.
- Schell SR, Wessels FJ, Abouhamze A, et al. Pro- and antiinflammatory cytokine production after radiofrequency ablation of unresectable hepatic tumors. J Am Coll Surg. 2002;195:774–781.
- Schneider T, Sevko A, Heussel CP, et al. Serum inflammatory factors and circulating immunosuppressive cells are predictive markers for efficacy of radiofrequency ablation in non-small-cell lung cancer. Clin Exp Immunol. 2015;180:467–474.
- Gu T, Ge Y, Song Y, et al. Hepatic radiofrequency ablation causes an increase of circulating histones in patients with hepatocellular carcinoma. Scand J Clin Lab Invest. 2015;75:621–627.
- Ypsilantis P, Lambropoulou M, Evagellou A, et al. Immune and inflammatory responses of the intestinal mucosa following extended liver radiofrequency ablation. Gastroenterol Res Pract. 2017;2017:3450635.
- Robbins PF, Dudley ME, Wunderlich J, et al. Cutting edge: persistence of transferred lymphocyte clonotypes correlates with cancer regression in patients receiving cell transfer therapy. J Immunol. 2004;173:7125–7130.
- D'Angelo SP, Melchiori L, Merchant MS, et al. Antitumor activity associated with prolonged persistence of adoptively transferred NY-ESO-1 (c259) T cells in synovial sarcoma. Cancer Discov. 2018;8:944–957.
- Huang J, Khong HT, Dudley ME, et al. Survival, persistence, and progressive differentiation of adoptively transferred tumor-reactive T cells associated with tumor regression. J Immunother. 2005;28:258–267.
- Brentjens RJ, Riviere I, Park JH, et al. Safety and persistence of adoptively transferred autologous CD19-targeted T cells in patients with relapsed or chemotherapy refractory B-cell leukemias. Blood. 2011;118:4817–4828.
- Zhou J, Dudley ME, Rosenberg SA, et al. Persistence of multiple tumor-specific T-cell clones is associated with complete tumor regression in a melanoma patient receiving adoptive cell transfer therapy. J Immunother. 2005;28:53–62.
- Park JR, Digiusto DL, Slovak M, et al. Adoptive transfer of chimeric antigen receptor re-directed cytolytic T lymphocyte clones in patients with neuroblastoma. Mol Ther. 2007;15:825–833.