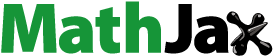
Abstract
Background: Because of the importance of adrenoreceptors in regulating the cardiovascular (CV) system and the role of the CV system in thermoregulation, understanding the response to these two stressors is of interest. The purpose of this study was to assess changes of arterial geometry and function in vivo during thermal and β-adrenergic stress induced in mice and quantified by MRI.
Methods: Male mice were anesthetized and imaged at 7 T. Anatomical and functional data were acquired from the neck (carotid artery), torso (suprarenal and infrarenal aorta and iliac artery) and periphery (femoral artery). Intravenous dobutamine (tail vein catheter, 40 µg/kg/min, 0.12 mL/h) was used as β-adrenergic stressor. Baseline and dobutamine data were acquired at minimally hypothermic (35 °C) and minimally hyperthermic (38 °C) core temperatures. Cross-sectional vessel area and maximum cyclic strain were measured across the cardiac cycle.
Results: Vascular response varied by location and by core temperature. For minimally hypothermic conditions (35 °C), average, maximum and minimum areas decreased with dobutamine only at the suprarenal aorta (avg: −17.9%, max: −13.5%, min: −21.4%). For minimally hyperthermic conditions (38 °C), vessel areas decreased between baseline and dobutamine at the carotid (avg: −19.6%, max: −15.5%, min: −19.3%) and suprarenal aorta (avg: −24.2%, max: −17.4%, min: −17.3%); whereas, only the minimum vessel area decreased for the iliac artery (min: −14.4%). Maximum cyclic strain increased between baseline and dobutamine at the iliac artery for both conditions and at the suprarenal aorta at hyperthermic conditions.
Conclusions: At hypothermic conditions, the vessel area response to dobutamine is diminished compared to hyperthermic conditions where the vessel area response mimics normothermic dobutamine conditions. The varied response emphasizes the need to monitor and control body temperature during medical conditions or treatments that may be accompanied by hypothermia, especially when vasoactive agents are used.
Introduction
The cardiovascular (CV) system plays a vital role in the human body’s ability to thermoregulate to withstand physiological states [Citation1–3]. Various pathological conditions and treatments, including sepsis [Citation4] and the treatment of cardiogenic shock [Citation5], can cause core body temperature to deviate from normothermia. Clinically, dobutamine is used in critical care for acute treatment of congestive heart failure, cardiogenic and septic shock, and as a pharmacological surrogate to exercise for stress tests [Citation6–8]. There is an interest in better understanding the combined effects of temperature and dobutamine because of the detrimental consequences of decreased peripheral resistance after dobutamine administration in hypothermic patients [Citation9,Citation10].
The primary mechanism of a racemic mixture of dobutamine is direct stimulation of β1-adrenergic receptors in the heart to increase cardiac contractility and output. However, one enantiomer is a β2-agonist and α1-antagonist while the other is an α1-agonist, which often leads to vasodilation [Citation11]. Conversely, temperature elicits a full-body autonomic response to maintain core body temperature. With increasing core temperature, regional changes in cerebral blood flow [Citation12–14], increased cardiac output [Citation15,Citation16] and decreased total peripheral resistance due to vasodilation [Citation15,Citation17] have been observed.
To improve parameterization and validation of mathematical and computational models [Citation18], recent work has used magnetic resonance imaging (MRI) to quantify geometric (cross-sectional area) and functional (Green-Lagrange circumferential strain) changes in core arteries and veins due to increases in core temperature [Citation19,Citation20]. Previous work by Crouch et al. [Citation20] showed that under hypothermic conditions (35 °C) the average area was significantly smaller in the infrarenal aorta and femoral artery by −12% and −72%, respectively, and larger for hyperthermic conditions (38 °C) by 1.3 and 6.0%, respectively, compared to normothermic conditions (37 °C). While cross-sectional area tended to increase with temperature, with larger changes occurring inferiorly, previous work in well-controlled and nearly identical conditions, Castle et al. [Citation21] showed that cross-sectional area at normothermic conditions decreased with the administration of dobutamine for the carotid, suprarenal and infrarenal aorta and iliac artery by −19%, −16%, −14% and −12%, respectively, with larger changes occurring superiorly in the body. For either increases in core temperature or dobutamine administration, the end-diastolic/minimum areas experienced larger changes than the peak-systolic/maximum areas, resulting in changes in maximum cyclic strain. Temperature’s effect on strain varied by location with a general overall decrease in strain from 35 to 38 °C [Citation19,Citation20], whereas dobutamine caused an increase in maximum cyclic strain for all vessels [Citation21]. Combining these two cardiac stressors is of interest because of the differential response of core vasculature to changing temperature or administration of dobutamine alone.
Preclinical animal models play a vital role in the advancement of therapeutic development and optimization of current treatment paradigms. It is important to understand the results of cardiac stressors in the healthy murine condition before studying their effects in preclinical CV disease models. With MRI, core vasculature geometry and function can be investigated noninvasively due to high spatial and temporal resolution. The purpose of this study was to assess physiological changes of arterial geometry and function in vivo during thermal and β-adrenergic stress via dobutamine using murine models and MRI. MRI data were acquired under hypothermic (35 °C) and hyperthermic (38 °C) conditions at the carotid artery, suprarenal and infrarenal aorta, iliac artery and femoral artery of C57BL/6 male mice, prior to and during dobutamine infusion.
We hypothesized that: (1) at hypothermic conditions (35 °C), dobutamine would not elicit changes in cross-sectional area and strain, due to temperature-induced vasoconstriction; and (2) at hyperthermic conditions (38 °C) cross-sectional area would decrease and strain would increase, mirroring dobutamine responses at normothermic conditions (37 °C). To our knowledge, these data are the first to empirically quantify the spatially and temporally resolved response of core vasculature to dobutamine at hypo- and hyper-thermic conditions in vivo from head-to-toe.
Methods
All experiments were carried out with local Institutional Animal Care and Use Committee approval. Animals were housed in a room with temperature (22 °C ± 2 °C) and humidity (∼27%) control with an alternate 12-h light/dark cycle.
Healthy adult male (13–15 weeks old, ∼20 human years [Citation22]) C57BL/6 mice, purchased from Charles River Laboratory, were used in this study. Male mice were chosen in this initial study combining hypo- and hyper-thermic states with dobutamine stimulation because this sex showed the smallest response to increases in core temperature in the aorta in previous work (male: 0.019 mm2/°C vs. female: 0.024 mm2/°C, [Citation19]). A dobutamine dosage of 40 µg/kg of body weight (Hospira, Inc., Lake Forest, IL) was prescribed at an infusion rate of 2 μL/min (Cole Parmer, Vernon Hills, IL) and pre-mixed assuming an average murine body weight of 25 g (actual mean and SEM of animals was 26.4 ± 0.7 g). Prior to imaging, a tail vein catheter was placed using a 30 gauge needle, connected to an ∼5 cm length of PE10 tubing prefilled with saline, followed by the dobutamine solution [Citation21]. Mice were anesthetized with 1.25–2% isoflurane in 1 L/min of oxygen [Citation23]. Animals were imaged in the supine position at 7 T field strength using a Direct Drive console (Agilent Technologies, Santa Clara, CA) and a 40 mm inner diameter transmit-receive volume coil (Morris Instruments, Ontario, Canada).
illustrates the locations investigated in this study and a protocol timeline. CINE data were acquired in the neck (carotid artery), torso (suprarenal and infrarenal aorta, iliac artery) and periphery (femoral artery). The two target core temperatures were minimally hypothermic (35 °C) and minimally hyperthermic (38 °C), controlled within ±0.2 °C using forced convection [Citation19]. The forced convection system includes a rectal temperature probe (core temperature), a heater blowing air through the bore of the magnet and over the animal, and a custom-built proportional-integral-derivative (PID) controller (Labview, National Instruments, Austin, TX). These temperatures were selected to avoid pathological changes [Citation24,Citation25]. Baseline and dobutamine data were acquired at all five locations for a given animal in two separate imaging sessions, corresponding to the two target core temperatures. Heart rate (HR) and respiration were monitored (SA Instruments, Stony Brook, NY). After acquiring baseline data at all five locations for the given target core temperature, dobutamine infusion was initiated. After a plateau in increased HR was achieved, slices planned at each of the five locations were acquired a second time during the infusion of dobutamine (referred to as ‘dobutamine’ in this paper). The total imaging time for each animal was approximately 90 min with approximately 60 min of dobutamine infusion (∼120 µL infused). Time to reach maximum HR plateau was 12 min of dobutamine infusion for hypothermic state compared to 19 min for hyperthermic state.
Figure 1. Coronal maximum intensity projection [Citation19] (left) and cross-sectional view of the arteries [Citation21] (right) illustrating arterial locations where imaging data were acquired and quantified. Schematic of protocol timeline (bottom).
![Figure 1. Coronal maximum intensity projection [Citation19] (left) and cross-sectional view of the arteries [Citation21] (right) illustrating arterial locations where imaging data were acquired and quantified. Schematic of protocol timeline (bottom).](/cms/asset/6ed6e5cc-e0ee-45c8-8385-811e87831981/ihyt_a_1684577_f0001_b.jpg)
Sagittal 2D and axial 3D acquisitions were used to plan slices perpendicular to the carotid artery. Coronal 2D and sagittal 3D acquisitions were used to plan slices perpendicular to the aorta, iliac and femoral arteries. A cardiac-gated and velocity compensated 2D CINE sequence with 16 frames was used to acquire data at each location. Parameters were TR/TE 120/4 ms, flip angle (α) 60°, field of view (20 mm)2, matrix 2562 zero-filled to 5122, zero-filled in-plane resolution (39 µm)2, slice thickness 1 mm, NEX 6.
The CINE images were analyzed for vessel cross-sectional area and circumferential cyclic strain using an in-house semi-automated process previously described [Citation19,Citation21,Citation26]. Circumferential cyclic strain is a measurement of the repetitive mechanical deformation of the vessel wall as it expands and constricts with the cardiac cycle. Green-Lagrange circumferential cyclic strain was calculated using the following equation:
(1)
(1)
where Pi is the perimeter at a given time frame and Pdias is the perimeter of the vessel at end-diastole.
Statistical analysis
Data are reported and plotted as mean ± standard error (SEM). To test if areas (average, maximum and minimum) and maximum cyclic strain differed significantly between baseline and dobutamine at a given target core temperature and location, a two-tailed paired t-test was used. To test if the response to dobutamine (average_areadobutamine – average_areabaseline) differed between the two temperatures for a given location, a two-tailed paired t-test was used. Two methods were used to compare the response at different locations. The response at different locations was compared using two-way ANOVA with Tukey’s post hoc test. The relative response ((average_areadobutamine – average_areabaseline)/average_areabaseline) was calculated to account for size-differences between vessels and was compared between locations using two-way ANOVA with Tukey’s post hoc test. Significance was set at p < .05.
Results
A summary of the main results is presented in .
Table 1. Summary of main findings of arterial vascular response to adrenergic and thermal stress by in vivo MRI investigation.
Heart rate (HR)
Dobutamine resulted in an elevated HR in all mice at 35 °C (baseline: 419 ± 6 to dobutamine: 541 ± 5 beats per minute, p < .0001) and at 38 °C (baseline: 482 ± 20 to dobutamine: 594 ± 9 beats per minute, p = .0003). HR was higher at 38 °C compared to 35 °C for both baseline (p = .002) and dobutamine (p = .007).
Effect of dobutamine at different core temperatures, within a location
Baseline vs. dobutamine during hypothermic or hyperthermic conditions
The first comparison was baseline versus dobutamine for vessel area (average, maximum and minimum) and maximum cyclic strain. Statistically significant results for the cross-sectional area across the cardiac cycle at baseline and during dobutamine for 35 and 38 °C are shown in . For the hypothermic condition (35 °C), dobutamine resulted in a decrease in vessel area in the suprarenal aorta by 17.9 ± 1.8% (p < .0001), 13.5 ± 3.3% (p = .005) and 21.4 ± 5.2% (p = .006) for average, maximum and minimum areas, respectively. For the hyperthermic condition (38 °C), dobutamine resulted in a decrease in vessel area in the carotid by 19.4 ± 5.7% (p = .006), 15.5 ± 6.7% (p = .04) and 19.6 ± 4.2% (p = .01) for average, maximum and minimum areas, respectively. In the suprarenal aorta, the vessel area decreased by 24.2 ± 2.8% (p = .0002), 17.4 ± 4.4% (p = .005) and 33.6 ± 2.2% (p < .0001) for average, maximum and minimum areas, respectively. In the iliac artery, the vessel area decreased by 14.4 ± 5.2% (p = .03) for the minimum area.
Figure 2. Cross-sectional area across the cardiac cycle for the (A) carotid artery, (B) suprarenal aorta, and (C) iliac artery at 35 and 38 °C (left and right) for baseline and dobutamine (n = 8 adult male mice). For the hypothermic condition (35 °C), area (avg: average, min: minimum/end-diastolic and max: maximum/peak-systolic) decreased with dobutamine for the suprarenal aorta (*). For the hyperthermic condition (38 °C), area decreased with dobutamine for the carotid (avg, min and max), suprarenal aorta (avg, min and max) and iliac artery (min). Diastolic peak at fraction of cardiac ∼1. Images are acquired for over one complete cardiac cycle to insure MRI data accurately captures systole and diastole. Error bars omitted for clarity.
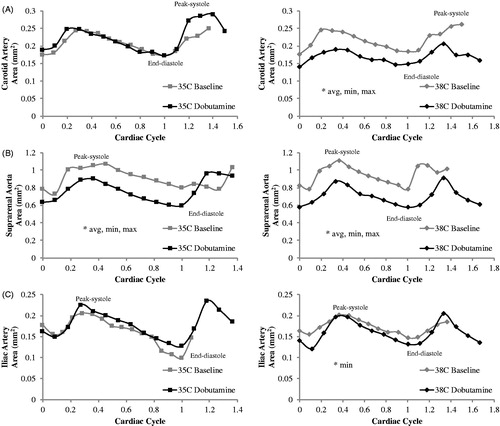
Statistically significant results for maximum cyclic strain at baseline and during dobutamine for 35 and 38 °C are shown in . For the hypothermic condition (35 °C), maximum cyclic strain increased with dobutamine in the iliac artery 20.3 ± 4.5% (p = .003). For the hyperthermic condition (38 °C), maximum cyclic strain increased with dobutamine in the suprarenal aorta by 21.0 ± 3.6% (p = .0006) and in the iliac artery by 17.3 ± 5.5% (p = .02).
Figure 3. Maximum cyclic strain across the cardiac cycle for suprarenal aorta (left) and iliac artery (right) at baseline and dobutamine for two core body temperatures 35 and 38 °C (n = 8 adult male mice). Strain increases from baseline to dobutamine for the iliac at 35 °C and both vessels at 38 °C. Significance set at p < .05. Comparing baseline to dobutamine at a given location and temperature (*).
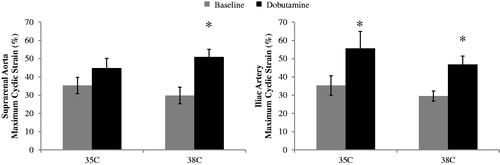
Comparison of the response during hypothermic vs. hyperthermic conditions
The response (dobutamine-baseline) at 35 °C was compared to the response at 38 °C for vessel areas and strain. The change in areas for all locations at 35 and 38 °C is shown from head-to-toe in . The response to dobutamine as measured by area varied between the temperatures. Statistically significant differences were seen at the carotid artery and suprarenal aorta. The change in carotid average area at 35 °C was an increase of 0.01 ± 0.01 mm2 compared to a decrease of −0.04 ± 0.01 mm2 (p = .005) at 38 °C. The change in carotid maximum area at 35 °C was an increase of 0.02±.01 mm2 compared to a decrease of −0.04 ± 0.01 mm2 (p = .004) at 38 °C. The change in suprarenal aorta minimum area at 35 °C was a decrease of −0.16 ± 0.04 mm2 compared to a decrease of −0.25 ± 0.03 mm2 (p = .03) at 38 °C.
Figure 4. The response to dobutamine (dobutamine – baseline) for average, minimum and maximum areas at 35 °C (left) and 38 °C (right) (n = 8 adult male mice). Comparing response between 35 and 38 °C at a given location, e.g., compare response at 35 to response at 38 for the suprarenal aorta (Δ). For a given temperature, the three responses at all other locations were significantly different from the corresponding values at the suprarenal aorta (#). Significance set at p < .05.
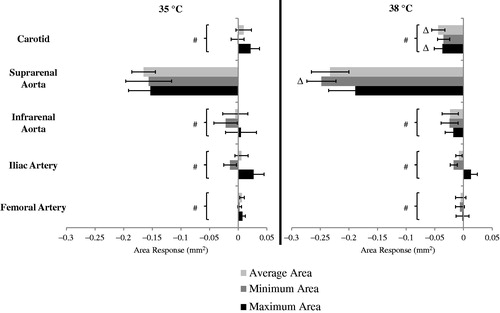
The maximum cyclic strain response for all locations at 35 and 38 °C is shown in . Maximum cyclic strain tended to increase with dobutamine at all locations and for both temperatures. However, there were no statistically significant differences when comparing the response at 35 to that at 38 °C.
Figure 5. The response to dobutamine (dobutamine – baseline) for maximum cyclic strain at 35 and 38 °C (n = 8 adult male mice). Strain tended to increase from baseline to dobutamine. Comparing response between 35 and 38 °C at a given location (NS); comparing response at 35 or 38 °C between locations (NS). Significance set at p<.05. NS: not significant.
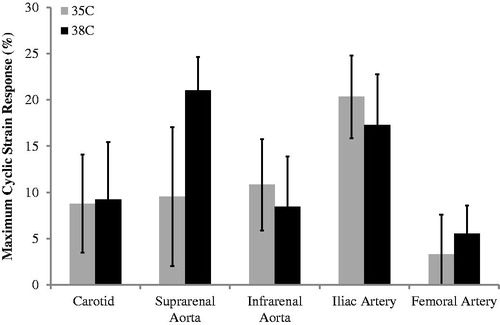
Effect of dobutamine at different core temperatures and across locations
To determine the effect of location on overall response, two comparisons were made: absolute response and relative response. For the absolute difference in response (), changes in area were dependent on location for average (p = .0001/0.005; location/temp), maximum (p = .0001/N.S.) and minimum (p = .0001/.007) areas. For the hypothermic condition (35 °C), pairwise comparisons were significantly different (p < .0001, all) between the suprarenal aorta (avg: −0.17 ± 0.02, max: −0.15 ± 0.04, min: −0.16 ± 0.04 mm2) and the carotid (0.01 ± 0.01, 0.02 ± 0.01, −0.001 ± 0.01 mm2), infrarenal aorta (−0.005 ± 0.02, 0.005 ± 0.03 and −0.02 ± 0.02 mm2), iliac artery (0.006 ± 0.01, 0.03 ± 0.01 and −0.01 ± 0.01 mm2) and femoral artery (0.006 ± 0.004, 0.007 ± 0.005 and −0.002 ± 0.003 mm2). For the hyperthermic condition (38 °C), pairwise comparisons were also significantly different (p < .001, all) between the suprarenal aorta (avg: −0.23 ± 0.03, max: −0.19 ± 0.04 and min: −0.25 ± 0.02 mm2) and the carotid (−0.04 ± 0.01, −0.04 ± 0.01 and −0.03 ± 0.01 mm2), infrarenal aorta (−0.023 ± 0.01, −0.02 ± 0.01 and −0.02 ± 0.01 mm2), iliac artery (−0.008 ± 0.006, 0.01 ± 0.01 and −0.02 ± 0.006 mm2) and femoral artery (−0.005 ± 0.009, −0.002 ± 0.01 and −0.006 ± 0.008 mm2).
Strain responses were not dependent on location (). However, the vessels varied in the magnitude of the change between 35 and 38 °C. For example, the carotid, suprarenal aorta and femoral artery had a larger increase during hyperthermic conditions, while the infrarenal aorta and iliac artery had a smaller increase.
Size-differences between the vessels were accounted for by calculating the relative response. The relative changes for average, maximum and minimum areas for all locations at 35 and 38 °C are shown in . All relative changes in area were dependent on location and temperature for average (p = .004/.003; location/temp), maximum (p = .02/.009) and minimum (p = .004/.01) areas. For average area, pairwise comparisons were significantly different at 35 °C between the suprarenal aorta and iliac artery (−0.18 vs. 0.07, p = .03) and the suprarenal aorta and femoral artery (−0.18 vs. −0.09, p = .003). For minimum area, significant differences occurred at 35 °C between the suprarenal aorta and femoral artery (−0.21 vs. 0.14, p = .02). For maximum area, significant differences occurred at 35 °C between the suprarenal aorta and iliac artery (−0.14 vs. 0.17, p = .03).
Discussion
Two CV stressors, deviations from normothermia and dobutamine administration, have been combined in this study. Because of the importance of adrenoreceptors in regulating the CV system and the role of the CV system in thermoregulation, understanding the response to these two stressors is of interest. To our knowledge, these data are the first to empirically quantify the spatially and temporally resolved response of arterial vasculature to a pharmacological stressor at different core body temperatures. The response to dobutamine, as measured by changes in vessel area, was diminished during hypothermic conditions while the response during hyperthermic conditions mimicked normothermia [Citation21].
For both temperature conditions, dobutamine resulted in elevated HR consistent with previous findings [Citation27,Citation28]. The hypothermic condition resulted in a slightly larger increase in HR of 29% compared to an increase of 23% in the hyperthermic condition, likely due to an increase in baseline HR at 38 °C [Citation19,Citation29]. Although not statistically significant for all vessels, dobutamine-induced decreases in area were more consistent across location during hyperthermic conditions. The suprarenal aorta exhibited distinct and greater decreases during both hypothermic and hyperthermic states. The unique response of the suprarenal aorta may be due to its position as the most superior vessel located below the heart. Another factor that may be influencing results at the suprarenal aorta is the influence of the gut. Interest in the brain-gut connection has grown after research has revealed that the enteric nervous system, coined the ‘second brain’, controls more than just digestion [Citation30,Citation31]. Due to the large nervous network, this location may be more susceptible to dobutamine. Further studies in the connection of the enteric nervous system and thermoregulation and dobutamine could provide interesting results.
Data presented here show that the vessel area response to dobutamine during minimally hyperthermic conditions mimics previously published data acquired during normothermic conditions. For the carotid artery, the relative area response was nearly identical (−20% at 38 °C vs. −19% at 37 °C, respectively). At the suprarenal location, the relative area response was larger during hyperthermic conditions (−24% vs. −16%, at 38 and 37 °C, respectively). For the infrarenal and iliac locations, the relative area response was smaller (infrarenal: −7% at 38 °C vs. −14% at 37 °C; iliac: −6% at 38 °C vs. −12% at 37 °C). Dobutamine-induced decreases in the average cross-sectional area of the core arteries during normothermic conditions are consistent with redistribution of blood to the skin/periphery due to dobutamine’s vasodilatory effects [Citation9,Citation21]. Conversely, cross-sectional area of the core arteries increases with increasing temperature, paralleling hyperthermia-induced vasodilation known to occur in the skin/periphery [Citation19,Citation20]. Comparing these recently published results with data presented here, one can begin to estimate the seemingly opposing factors of changes in core temperature and dobutamine administration on core arteries. For example, Crouch et al. [Citation20] quantified an ∼4%, 1% and 6% increase in area for the carotid artery, infrarenal aorta and femoral artery, respectively, when core temperature is increased from 37 to 38 °C. Here, for hyperthermia with dobutamine relative to hyperthermia alone we show a ∼20%, 7% and 5% decrease in area for the same vessels, respectively. This implies that the vasodilatory effects of dobutamine on downstream vasculature outweigh the local effects of temperature. This balance becomes less evident in the more superficial vessels (i.e., the femoral artery), which could be related to fewer vessels downstream to be dilated by dobutamine and/or the fact that these vessels, themselves, play a larger role in heat exchange with the environment.
Dobutamine administration during hypothermia resulted in smaller or no decreases in area for the carotid, suprarenal and infrarenal aorta compared to hyperthermic conditions. Crouch et al. [Citation20] also demonstrated that the cross-sectional area of some core arteries decreases due to decreases in temperature, paralleling hypothermia-induced vasoconstriction known to occur in the skin/periphery. For example, while there was a 7% increase for the carotid artery at 35 °C compared to 37 °C, conversely, the areas of the infrarenal aorta and femoral artery decreased by 11% and 72%, respectively. Here, we show a 5% increase in area at the carotid, no change at the infrarenal aorta and a 13% increase in the femoral artery when dobutamine is applied at 35 °C. This suggests that reductions in area of the core arteries due to hypothermia are mitigated by the application of dobutamine. Similarly, Oung et al. [Citation10] showed an increase in vasodilation from dobutamine during hypothermic conditions compared to normothermic conditions. Focusing on the femoral artery, the difference between a large reduction in area due to hypothermia, to minimize heat loss to the environment [Citation15,Citation17,Citation19], and an enlargement when dobutamine [Citation9] is applied during hypothermia would be hypothesized to cause further decreases in core temperature due to increased heat exchange with the environment. However, these structural changes are not necessarily indicative of blood flow changes. In addition to blood pressure measurements, laser Doppler imaging and phase contrast MRI could be used to determine whether changes in subcutaneous perfusion and blood flow velocity and volume, respectively, are accompanying geometric alterations.
Circumferential cyclic strain is a calculation of vessel deformation across the cardiac cycle [Citation32]. Reductions in strain can accompany the onset of pathology [Citation33–35]. Consistent with previous work [Citation20], baseline strain was qualitatively larger during hypothermic conditions compared to hyperthermic conditions. Like normothermia [Citation21], maximum cyclic strain increased with dobutamine during both hypothermic and hyperthermic conditions, reaching significance for some core arteries. Notably, the elastic arteries (carotid artery, aorta and iliac artery), which act to dampen the pulsatility of flow from the heart through expansion and elastic recoil [Citation36], had qualitatively greater increases in maximum cyclic strain (1.5–6 fold larger) compared to the only muscular artery studied in this work (femoral artery).
The adenosine A1 receptors which are involved in the regulation of body temperature, HR and locomotion activity [Citation37] are likely interacting with the adrenergic receptors stimulated by dobutamine in this work [Citation38] causing a varied vascular response during the hypo- and hyper- thermic conditions as well as differences across locations. Previous in vitro studies using isoproterenol, a β-adrenoreceptor specific agonist, show a vasodilatory effect in the core vessels. However, studies using isolated vessels cannot account for blood flow redistribution due to changes in downstream resistance resulting from vasodilation [Citation39]. Changes in downstream resistance may be caused by the response of the microcirculatory system to the administration of dobutamine, leading to increased pressure gradients/drops within the arterial system. Chruscinski et al. [Citation39] did show that the distribution of β1 and β2 receptors varied by location, and this could partially explain the location-dependent response seen in this study and may also affect microcirculatory flow. In future studies, the noninvasive methods established in this work can be combined with more specific reagents to determine the contribution of receptors and control mechanisms for both thermoregulation and β-adrenergic stimulation.
While hypo- and hyper-thermia have been used as therapeutic treatments, in relation to surgery, hypothermia due to anesthesia has been associated with increases in surgical site infection, transfusions, and length of hospital stays, as well as delayed wound healing [Citation40,Citation41]. A thorough review of more successfully managing body temperature intra- and peri-operatively during abdominal surgery revealed improved outcomes [Citation42]. However, there are no guidelines on when, where on the body, or how to monitor and control body temperature intraoperatively [Citation43]. Critical care treatment may require the use of anesthesia and/or dobutamine when the patient already has an altered core temperature. Here, our data suggest that the addition of vasoactive agents when the patient is already hypothermic likely compromises the ability of the patient to achieve normothermia due to the role the CV system plays in thermoregulation. Put more simplistically, a hypothermic patient given dobutamine will drop further into hypothermia, potentially exacerbating poor outcomes. Under such circumstances, core temperature monitoring is even more important and likely necessitates prolonged monitoring and supplementary temperature control.
Although we did not have saline control in this study; previous studies administering similar volumes of saline demonstrated minimal effect in the heart [Citation44] or the vasculature [Citation21] as compared to dobutamine. Regarding potential effects of isoflurane on vasculature, Crouch et al. [Citation20] showed minimal changes in vasculature or HR after a two-hour anesthesia exposure. Maximizing the number of anatomical locations, while minimizing blood volume increases due to infusion and time under anesthesia, necessitated the foci on hypo- and hyper-thermic conditions. Hence, normothermic data were not included in this study. However, the metrics of our study have been shown to be reproducible in the arteries of small-animal preclinical models during normothermic baseline conditions, even across laboratories, field strengths and modalities [Citation14,Citation31]. Dobutamine response also varies between mice and humans, particularly in the heart, with human studies showing stroke volume changes [Citation11] whereas these changes are not seen in the mouse [Citation27]. Human studies would be necessary to determine if dobutamine response in the vasculature is consistent across species.
In conclusion, this study provides quantitative insight into CV responses to two clinically relevant stressors, administration of dobutamine and variation of core temperature, while illustrating an innovative noninvasive approach using MRI. To our knowledge, these data are the first to empirically quantify the spatially and temporally resolved response of core vasculature to dobutamine at hypo- and hyper-thermic conditions in vivo from head-to-toe. The study was performed in healthy male mice and not a specific disease model. However, stressing the CV system can reveal deficits that otherwise remain undetectable at rest. Therefore, these initial data for healthy animals subjected to two cardiac stressors can be used to compare future measurements from disease models, such as cardiac failure or sepsis, to quantify early deficits, thereby helping to improve our understanding of how changes in core temperature interact with a clinical standard of treatment. Our data show that the response in core vasculature depends on anatomical location and varies for hypothermic and hyperthermic conditions. The results presented here also provide foundational data (geometry of the vessels) to begin coupling empirical values of the physiological response to temperature and dobutamine with computational fluid dynamics modeling to better understand how the CV system responds to stress. A better understanding of how the CV system responds to these two stressors, independently and combined, could provide motivation for further clinical studies on the effects of temperature and adrenergic stress on core vasculature.
Ethics approval and consent to participate
All experiments were carried out with University of Michigan Institutional Animal Care and Use Committee approval.
Author contributions
ACC and PEC conceived and designed the study; ACC, PEC, LNF collected and analyzed data; ACC and PEC performed statistical analysis and interpreted the data; ACC and JMG wrote the paper; ACC, PEC, UMS, and JMG critically revised the paper; all authors gave final approval of the paper.
Availability of data and material
The datasets used and/or analyzed during this study are available from the corresponding author upon reasonable request.
Disclosure statement
The authors report no conflicts of interest.
Additional information
Funding
References
- Tucker R, Rauch L, Harley YR, et al. Impaired exercise performance in the heat is associated with an anticipatory reduction in skeletal muscle recruitment. Pflugers Arch Eur J Physiol. 2004;448:422–430.
- Stewart IB, Rojek AM, Hunt AP. Heat strain during explosive ordnance disposal. Mil Med. 2011;176:959–963.
- Polderman KH. Application of therapeutic hypothermia in the intensive care unit. Intensive Care Med. 2004;30:757–769.
- Schortgen F. Fever in sepsis. Minerva Anestesiol. 2012;78:1254–1264.
- Schmidt-Schweda S, Ohler A, Post H, et al. Moderate hypothermia for severe cardiogenic shock (COOL shock study I & II). Resuscitation. 2013;84:319–325.
- Mauro VF, Mauro LS. Use of intermittent dobutamine infusion in congestive heart failure. Drug Intell Clin Pharm. 1986;20:919–924.
- Abram S, Arruda-Olson AM, Scott CG, et al. Typical blood pressure response during dobutamine stress echocardiography of patients without known cardiovascular disease who have normal stress echocardiograms. Eur Heart J Cardiovasc Imaging. 2016;17:557–563.
- Brink HL, Dickerson JA, Stephens JA, et al. Comparison of the safety of adenosine and regadenoson in patients undergoing outpatient cardiac stress testing. Pharmacotherapy. 2015;35:1117–1123.
- Shitara T, Wajima Z, Ogawa R. Dobutamine infusion modifies thermoregulation during general anesthesia. Anesth Analg. 1996;83:1154–1159.
- Oung CM, English M, Chiu RJ, et al. Effects of hypothermia on hemodynamic responses to dopamine and dobutamine. J Trauma. 1992;33:671–678.
- Ruffolo RR. Review: the pharmacology of dobutamine. Am J Med Sci. 1987;294:244–248.
- Nybo L, Møller K, Volianitis S, et al. Effects of hyperthermia on cerebral blood flow and metabolism during prolonged exercise in humans. J Appl Physiol. 2002;93:58–64.
- Bain AR, Nybo L, Ainslie PN. Cerebral vascular control and metabolism in heat stress. Compr Physiol. 2015;5:1345–1380.
- Qian S, Jiang Q, Liu K, et al. Effects of short-term environmental hyperthermia on patterns of cerebral blood flow. Physiol Behav. 2014;128:99–107.
- Crandall CG. Heat stress and baroreflex regulation of blood pressure. Med Sci Sports Exerc. 2008;40:2063–2070.
- Siddiqui A. Effects of vasodilation and arterial resistance on cardiac output. J Clin Exp Cardiolog. 2011;02:170.
- Kuhn LA, Turner JK. Alterations in pulmonary and peripheral vascular resistance in immersion hypothermia. Circ Res. 1959;7:366–374.
- Bhowmik A, Singh R, Repaka R, et al. Conventional and newly developed bioheat transport models in vascularized tissues: a review. J Therm Biol. 2013;38:107–125.
- Crouch AC, Manders AB, Cao AA, et al. Cross-sectional area of the murine aorta linearly increases with increasing core body temperature. Int J Hyperth. 2018;34:1121–1133.
- Crouch AC, Scheven UM, Greve JM. Cross-sectional areas of deep/core veins are smaller at lower core body temperatures. Physiol Rep. 2018;6:e13839.
- Castle PE, Scheven UM, Crouch AC, et al. Anatomical location, sex, and age influence murine arterial circumferential cyclic strain before and during dobutamine infusion. J Magn Reson Imaging. 2019;49:69–80.
- Flurkey K, Mcurrer J, Harrison D. Mouse models in aging research. In: Fox JG, Barthold SW, Davisson MT, et al., editors. The mouse in biomedical research. Vol. 3. New York: Elsevier; 2007. p. 637–672.
- Constantinides C, Mean R, Janssen BJ. Effects of isoflurane anesthesia on the cardiovascular function of the C57BL/6 mouse. ILAR J. 2011;52:e21–31.
- Teng D, Hornberger TA. Optimal temperature for hypothermia intervention in mouse model of skeletal muscle ischemia reperfusion injury. Cel Mol Bioeng. 2011;4:717–723.
- Duhan V, Joshi N, Nagarajan P, et al. Protocol for long duration whole body hyperthermia in mice. J Vis Exp. 2012;e3801.
- Palmer OR, Chiu CB, Cao A, et al. In vivo characterization of the murine venous system before and during dobutamine stimulation: implications for preclinical models of venous disease. Ann Anat Anat Anzeiger. 2017;214:43–52.
- Wiesmann F, Ruff J, Engelhardt S, et al. Dobutamine-stress magnetic resonance microimaging in mice: acute changes of cardiac geometry and function in normal and failing murine hearts. Circ Res. 2001;88:563–569.
- Ahonen J, Aranko K, Iivanainen A, et al. Pharmacokinetic-pharmacodynamic relationship of dobutamine and heart rate, stroke volume and cardiac output in healthy volunteers. Clin Drug Investig. 2008;28:121–127.
- Minson CT, Wladkowski SL, Cardell AF, et al. Age alters the cardiovascular response to direct passive heating. J Appl Physiol. 1998;84:1323–1332.
- Gershon M. The second brain: the scientific basis of gut instinct and a groundbreaking new understanding of nervous disorders of the stomach and intestines. New York: HarperCollins; 1998.
- Carabotti M, Scirocco A, Maselli MA, et al. The gut-brain axis: interactions between enteric microbiota, central and enteric nervous systems. Ann Gastroenterol. 2015;28:203–209.
- Humphrey JD, O’Rourke SL. An introduction to biomechanics: solids and fluids, analysis and design. Berlin (Germany): Springer-Verlag; 2004.
- Phillips EH, Di Achille P, Bersi MR, et al. Multi-modality imaging enables detailed hemodynamic simulations in dissecting aneurysms in mice. IEEE Trans Med Imaging. 2017;36:1297–1305.
- Phillips EH, Yrineo AA, Schroeder HD, et al. Morphological and biomechanical differences in the elastase and angII apoE(-/-) rodent models of abdominal aortic aneurysms. Biomed Res Int. 2015;2015:1.
- Goergen CJ, Azuma J, Barr KN, et al. Influences of aortic motion and curvature on vessel expansion in murine experimental aneurysms. Arterioscler Thromb Vasc Biol. 2011;31:270–279.
- Quinn U, Tomlinson LA, Cockcroft JR. Arterial stiffness. JRSM Cardiovasc Dis. 2012;1:1–8.
- Yang JN, Tiselius C, Daré E, et al. Sex differences in mouse heart rate and body temperature and in their regulation by adenosine alpha1 receptors. Acta Physiol. 2007;190:63–75.
- Schimmel RJ, Elliott ME, Dehmel VC. Interactions between adenosine and alpha 1-adrenergic agonists in regulation of respiration in hamster brown adipocytes. Mol Pharmacol. 1987;32:26–33.
- Chruscinski A, Brede ME, Meinel L, et al. Differential distribution of beta-adrenergic receptor subtypes in blood vessels of knockout mice lacking beta(1)- or beta(2)-adrenergic receptors. Mol Pharmacol. 2001;60:955–962.
- Sun Z, Honar H, Sessler DI, et al. Intraoperative core temperature patterns, transfusion requirement, and hospital duration in patients warmed with forced air. Anesthesiology. 2015;122:276–285.
- Wang H, Pei H, Chen M, et al. Incidence and predictors of surgical site infection after ORIF in calcaneus fractures, a retrospective cohort study. J Orthop Surg Res. 2018;13:293.
- Madrid E, Urrútia G, Roqué i Figuls M, et al. Active body surface warming systems for preventing complications caused by inadvertent perioperative hypothermia in adults. Cochrane Database Syst Rev. 2016;4:CD009016.
- Bindu B, Bindra A, Rath G. Temperature management under general anesthesia: compulsion or option. J Anaesthesiol Clin Pharmacol. 2017;33:306–316.
- Puhl SL, Weeks KL, Ranieri A, et al. Assessing structural and functional responses of murine hearts to acute and sustained β-adrenergic stimulation in vivo. J Pharmacol Toxicol Methods. 2016;79:60–71.