Abstract
The emergence of antibiotic-resistant bacteria in the last century is alarming and calls for alternative, nonchemical treatment strategies. Thermal medicine uses heat for the treatment of infectious diseases but its use in facultative and obligate intracellular bacteria remains poorly studied. In this review, we summarize previous research on reducing the infectious burden of Mycobacterium ulcerans and Chlamydia trachomatis by using water-filtered infrared A-radiation (wIRA), a special form of heat radiation with high tissue penetration and low thermal load on the skin surface. Mycobacterium ulcerans is a thermosensitive bacterium causing chronic necrotizing skin disease. Therefore, previous data on wIRA-induced improvement of wound healing and reduction of wound infections is summarized first. Then, pathogenesis and treatment of infections with M. ulcerans causing Buruli ulcer and of those with C. trachomatis infecting the ocular conjunctiva and resulting in blinding trachoma are discussed. Both bacteria cause neglected tropical diseases and have similar geographical distributions. Results of previous in vitro and in vivo studies using wIRA on M. ulcerans and C. trachomatis infections are presented. Finally, technical aspects of using wIRA in patients are critically reviewed and open questions driving future research are highlighted. In conclusion, wIRA is a promising tool for reducing infectious burden due to intracellular bacteria such as M. ulcerans and C. trachomatis.
Introduction
Bacterial infections in human patients are considered an immediate threat to global health due to increasing antibiotic resistance and diminishing availability of antibiotics to treat these infections [Citation1]. The emergence of antibiotic-resistant gram-positive and gram-negative bacteria in the last century is alarming and calls for alternative, nonchemical treatment strategies. Thermal medicine uses heat for the treatment of infectious diseases and is attracting increasing interest as an alternative or combination treatment in medical microbiology. The use of thermal therapy to treat infectious diseases was highlighted in a recent special issue of the International Journal of Hyperthermia [Citation2] and again reviewed in a subsequent issue of this journal [Citation3].
Thermal therapy can be used to treat local or systemic infections and has significant advantages over antibiotic treatment regimens when ease of use, side effects, costs, and development of resistance are considered [Citation2–4]. Wardlow et al. [Citation5] combined heat with temperature-targeted delivery of antibiotics, using low temperature sensitive liposomes as a tool to enhance antimicrobial efficiency. Even though manifold thermal therapy-related research has been undertaken, thermal therapies mainly target extracellular bacteria and bacterial biofilms, e.g., Pseudomonas aeruginosa biofilms on implanted medical devices [Citation3,Citation4,Citation6] or Staphylococcus aureus-induced abscesses or biofilms in mouse or rat models [Citation5,Citation7].
Thermal modalities used to combat bacterial infections are manifold, including radiofrequency, ultrasound, high-intensity focused ultrasound, microwave therapy, and photothermal and magnetic nanoparticles [reviewed in Citation4]. Another source of thermal therapy is water-filtered infrared A (wIRA), a special form of heat radiation with high tissue penetration and a low thermal load on the skin surface [Citation8–10]. The high tissue penetration of wIRA is beneficial when treating deeper tissue layers of up to 20–30 mm depth containing resident extra- or intracellular pathogens. However, direct wIRA effects on pathogens in vitro and in particular on obligate intracellular infectious agents (such as chlamydiae) have not been studied before.
As mentioned above, most thermal therapies focus on extracellular bacteria whereas strict and facultative obligate intracellular bacteria remain poorly studied. In an effort to include intracellular bacteria, and possibly other intracellular pathogens, in the recent discussion of novel thermal therapy approaches, we summarize results of previous research on wIRA-induced hyperthermia as a treatment option, focusing on Mycobacterium ulcerans and Chlamydia trachomatis.
wIRA and hyperthermia
Water-filtered infrared A (wIRA) is infrared radiation with a spectrum of 780–1400 nm. It is produced by light from a halogen bulb passing through a water cuvette to abate the irradiances at 970 nm and 1160 nm, and to exclude wavelengths above 1400 nm. Major contributions of visible light (VIS) can be blocked by using an optical longpass filter with a cutoff wavelength at 590 nm [Citation11,Citation12], whereas black filters block VIS (380–780 nm) entirely [Citation13].
Clinical trials have shown that wIRA improves acute and chronic wound healing processes and prevents wound infection [Citation8,Citation14–16]. In two randomized controlled trials [Citation14,Citation16], the utilization of wIRA radiation and its impact on surgical site infection in abdominal surgery was studied. Since impaired wound healing caused by infections leads to pain, longer hospitalization, higher costs [Citation18], and poor cosmetic results [Citation8], several approaches were undertaken to reduce the incidence of these outcomes. Beside sterile working conditions and correct antibiotic prophylaxis [Citation19], heating of the incisional area [Citation18] has found application, since wound repair is influenced by tissue temperature, oxygen tension and perfusion [Citation21–24]. Whole body warming [Citation25] and heat packs have been used to reduce wound healing impairment but can also result in adverse effects—especially skin burns—since heat is absorbed by the outermost skin layer (epidermis). One major advantage of thermal wIRA application consists of its peak in warming the subcutaneous layer at about 20–30 mm depth, as demonstrated by [Citation14] in a double-blinded postoperative protocol of local abdominal wIRA treatment after laparotomies. The authors were able to show a significant difference in favor of the wIRA group in four out of five main study variables: subcutaneous oxygen partial pressure, subcutaneous temperature and evaluation of wound healing by the surgeon, and also a trend toward fewer wound infections [Citation14]. This positive outcome can be attributed to a combination of thermal, perfusion-increasing effects as well as nonthermal causes by direct stimulation of cellular immunomodulation and induction of protective proteins [Citation26,Citation27].
A second randomized control trial [Citation16] investigated the effect of a single, preoperative wIRA treatment in patients undergoing median laparotomies during visceral surgery. A total of 360 patients were randomly allocated to two groups—treated either by a wIRA radiator or a sham lamp for 20 min before surgery. A significant reduction in wound infection in combination with antibiotic prophylaxis could be demonstrated (5.1%, CI: 3–9% in wIRA irradiated group vs. 12.1%, CI: 8–18%, p = 0.018) over a 30-day period postoperatively. One hypothesis suggested that wIRA therapy improved perfusion in the subcutaneous layer—a region where antibiotics normally reach lower serum levels [Citation28]—and thus results in reduced, especially superficial, surgical site infections due to better perfusion and local distribution of antibiotic agents.
Another field of wIRA application [Citation29] includes the management of partial thickness skin graft transplantation. A transplant is commonly less perfused due to its dependence on local blood supply and also due to the lack of an intact skin barrier, making it more prone to infections.
Local hyperthermia facilitates the reduction of the radiation dose in the radiation therapy of cancer, which allows, e.g., re-irradiations of recurrent breast cancer [Citation30]. wIRA could be a suitable device for achieving a homogeneous irradiation of larger skin areas, as shown recently in a study performed in piglets [Citation31], while, e.g., heat packs lead to focal hyperthermia or even skin burns without reaching therapy-relevant temperature increases in larger skin areas or deeper skin layers. Piazena et al. [Citation31] allocated 11 piglets into three intervention groups and irradiated the skin of their thighs with different doses (irradiances) of wIRA. Skin temperatures were measured before, during and after the wIRA irradiation in a 1-min interval in tissue depths of 2, 4, 7, 8, 10, 12, 16, and 20 mm. The temperature measurement revealed (1) significant individual differences between temperature profiles and the thermoregulatory processes, (2) reproducible vertical temperature profiles through the skin layers and subcutis, showing the highest temperature in 4–6 mm depth of the tissue and (3) that the temperature measured at the skin surface is approximately 1–2 K lower than at 4 mm skin depth, allowing an approximate estimation of the temperature reached in deeper layers by temperature measurements at the skin surface [Citation31].
Further studies will be needed to assess the optimal time and dose exposure in thermal therapies, since the mean decay time (time after irradiation until the tissue reaches the target temperature (e.g., 39 °C) has been shown to be dependent on irradiation dose, depth of tissue, and individual factors [Citation31]. These are important parameters to consider for future wIRA use in human patients, e.g., when skin treatment of Buruli ulcer-affected patients is envisaged.
Buruli ulcer (Mycobacterium ulcerans disease)
Pathogenesis of Buruli ulcer
Buruli ulcer (BU), caused by infection with Mycobacterium ulcerans, is a chronic necrotizing skin disease that primarily affects the subcutaneous fat tissue, leading to ulceration of the overlying dermal and epidermal layers of the skin [Citation32]. The disease is reported from tropical regions worldwide, but has its highest prevalence in West Africa [Citation33], (). Three distinct non-ulcerative stages of the disease are described: (i) subcutaneous, painless and movable nodules or papules, (ii) edema, and (iii) plaques. All of them may progress to ulceration, once the destruction of the subcutis results in collapse of the overlying epidermis and dermis [Citation32]. The majority of BU patients have a single skin lesion located at the upper or lower extremities, but all body surfaces can be affected [Citation32]. Mycobacterium ulcerans has a low optimal growth temperature of 30–33 °C [Citation34,Citation35] and this temperature preference is considered a major factor for the explicit skin tropism and the limited systemic dissemination of M. ulcerans infections [Citation36].
Figure 1. World map showing the distribution of trachoma and Buruli ulcer (BU). Countries colored in red have reported cases of Buruli ulcer in the year 2018. Countries colored in blue have reported trachoma cases in the year 2019 and are still considered to require intervention programs to eliminate trachoma. The purple color represents the overlap of required trachoma elimination and reported BU cases.
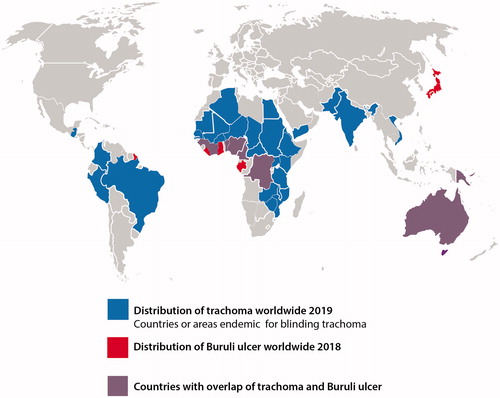
Mycobacterium ulcerans produces the polyketide exotoxin mycolactone, which plays a key role in the pathogenesis of BU and has cytotoxic, analgesic, and immunosuppressive properties [Citation37]. Exposure of different types of mammalian cells to low nanomolar concentrations of mycolactone A/B leads to cell death within 2–3 days [Citation37]. While mycolactone is produced locally by the extracellular M. ulcerans bacteria in the skin, there is evidence that nontoxic concentrations in the periphery induce systemic immunosuppressive activity [Citation38]. Macrophages and lymphocytes accumulate at the margin of the necrotic core of BU lesions, but infiltrating leukocytes seem to be killed by mycolactone surrounding the M. ulcerans clusters in the subcutaneous tissue [Citation39]. Interference with the production of mycolactone may thus be sufficient for achieving therapeutic efficacy.
Treatment of BU
Traditionally, surgical treatment with wide excision margins was the only therapeutic option for BU, as antibiotic therapy was considered ineffective [Citation40,Citation41]. In most rural BU-endemic regions of Africa, the available surgical capacity was, however, not sufficient to provide treatment for the majority of BU patients [Citation42]. Moreover, large excisions were not well accepted by the patients, but were necessary to avoid relapses [Citation43,Citation44]. In 2004, the World Health Organization (WHO) published ‘Provisional guidance on the role of specific antibiotics in the management of M. ulcerans disease’, recommending a combination therapy with oral rifampicin and intramuscular streptomycin for eight weeks [Citation45]. As treatment with streptomycin has been shown to frequently cause permanent ototoxicity and more occasionally transient nephrotoxicity, the WHO Technical Advisory Group on Buruli ulcer recommended replacing streptomycin with oral clarithromycin in 2017 [Citation46]. However, rifampicin is currently the only highly efficacious antibiotic against M. ulcerans, and no alternative drug treatment modality would currently be available in the case of the emergence of rifampicin-resistant strains [Citation40]. Repurposing of drugs under development for tuberculosis is the most promising approach for the identification of new antibiotics active against M. ulcerans [Citation40], and recent evidence indicates that the tuberculosis development candidate Q203, could both simplify and shorten BU treatment [Citation47].
Thermosensitivity of M. ulcerans
Mycobacterium ulcerans grows optimally on bacteriological media at 30–33 °C and the viability of cultures decreases after several days at 37 °C [Citation35]. Furthermore, it has been found that heat exposure of the bacilli to 37 °C for 1 day retards subsequent growth at 32 °C and that exposure to 41 °C for 1 day kills >90% of the bacilli [Citation48]. To gain more information on the exposure times required for thermotherapy, we have investigated the thermosensitivity of M. ulcerans strains freshly isolated from Cameroonian BU patients [Citation49] in more detail. An Alamar Blue-based growth inhibition assay adapted for the extremely slow-growing M. ulcerans [Citation50] was used to assess the temperature effects on metabolic activity of the bacilli (). Results demonstrated that daily temperature pulses at 42 °C for 20 min had no marked effect, while pulses of 1 h had moderate and 3 h had marked effects. Pulses of up to 3 h at 37 °C were ineffective. These data indicate that for the thermotherapy of BU, short temperature pulses may be ineffective.
Figure 2. Effect of temperature pulses of 37 °C (A) or 42 °C (B) or permanent exposure to 30°C (C) on the metabolic activity of M. ulcerans. Cultures of the three freshly isolated [Citation49] Cameroonian M. ulcerans strains S1012 (black bars), S1013 (empty bars), and S1047 (grey bars) were grown in Difco Middlebrook 7H9 medium (BD), supplemented with 10% (vol/vol) Middlebrook OADC enrichment medium (BD) and 0.2% (vol/vol) glycerol at 30 °C in triplicate in microtiter plates. The bacterial cultures were treated for 5 days with one temperature pulse of 20 min, one hour or three hours per day. After 2 days of recovery at 30 °C, a resazurin microtiter assay adapted for the extremely slow-growing M. ulcerans [Citation50] was used to determine the metabolic activity of the bacteria. After the addition of 10% (vol/vol) of a resazurin solution (0.125 mg/mL, Sigma) to the cultures and incubation for another 3 days at 30 °C, end-point fluorescence reading (Ex540nm/Em588nm) was performed. Values represent means of fluorescent units ± SD.
![Figure 2. Effect of temperature pulses of 37 °C (A) or 42 °C (B) or permanent exposure to 30°C (C) on the metabolic activity of M. ulcerans. Cultures of the three freshly isolated [Citation49] Cameroonian M. ulcerans strains S1012 (black bars), S1013 (empty bars), and S1047 (grey bars) were grown in Difco Middlebrook 7H9 medium (BD), supplemented with 10% (vol/vol) Middlebrook OADC enrichment medium (BD) and 0.2% (vol/vol) glycerol at 30 °C in triplicate in microtiter plates. The bacterial cultures were treated for 5 days with one temperature pulse of 20 min, one hour or three hours per day. After 2 days of recovery at 30 °C, a resazurin microtiter assay adapted for the extremely slow-growing M. ulcerans [Citation50] was used to determine the metabolic activity of the bacteria. After the addition of 10% (vol/vol) of a resazurin solution (0.125 mg/mL, Sigma) to the cultures and incubation for another 3 days at 30 °C, end-point fluorescence reading (Ex540nm/Em588nm) was performed. Values represent means of fluorescent units ± SD.](/cms/asset/1da920fe-c19d-4d5f-bf7c-ab89feec91e6/ihyt_a_1751312_f0002_b.jpg)
The effects of temperature pulses on the viability of the bacteria were analyzed by determination of the number of colony-forming units (CFUs). A nearly complete killing of the bacteria was achieved by five temperature pulses of three hours at 42 °C (). While five days of permanent incubation of the bacilli at 42 °C led to complete eradication, five days of incubation at 37 °C had only limited effect on viability.
Figure 3. Effects of five daily temperature pulses of 20 min or 3 h at 42 °C on the viability of M. ulcerans (shown by the mean number of colony-forming units, (CFUs) ± SD for the three tested strains). Liquid cultures of the three freshly isolated Cameroonian M. ulcerans strains S1012, S1013, and S1047 grown at 30 °C in Difco Middlebrook 7H9 medium (BD), supplemented with 10% (vol/vol) Middlebrook OADC enrichment medium (BD) and 0.2% (vol/vol) glycerol were exposed to temperature pulses at 42 °C on five consecutive days, then serially diluted and plated on Difco Middlebrook 7H10 agar plates (BD), supplemented with 10% (vol/vol) Middlebrook OADC enrichment medium (BD) and 0.2% (vol/vol) glycerol. The number of CFUs was determined after four months of incubation of the extremely slow growing bacilli at 30 °C. In addition, CFU counts of cultures incubated for 5 days at 37 °C or 42 °C were compared with a control remaining at 30 °C.
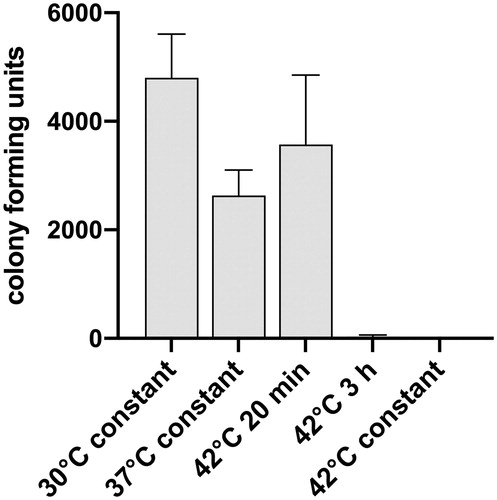
Taken together, these in vitro studies indicate that for thermotherapy of BU, heating the skin to elevated temperatures (e.g., 43 °C) for extended periods of time may be required to kill the bacilli. The safety aspects of increased tissue temperature, e.g., based on the CEM43 model, still require further investigations, since biological effects of heat therapy on normal human tissues are not entirely understood [Citation51]. However, even a reduction in the metabolic activity of the bacteria may lead to a drop in the production of mycolactone. This may allow phagocytes to reach the bacteria and to contribute to the elimination of the infection [Citation39,Citation52,Citation53].
Thermotherapy of BU
The thermosensitivity of M. ulcerans was first explored for therapeutic purposes by Meyers and colleagues in the 1970s [Citation51]. Eight patients from Zaire were successfully treated using circulating water jackets, maintaining a temperature of approximately 40 °C in the ulcerated area for a mean duration of 68 days. No local recurrences were observed during follow-up periods of up to 22 months [Citation54]. WHO guidelines subsequently listed the application of heat as a treatment option for BU (WHO 2000), but the heat application devices employed by Meyers were impractical in rural endemic areas of Africa and the heat treatment approach was not followed up for more than 30 years. However, in the early 2000s, use of commercially available silicone bags (210 × 155 × 20 mm) filled with the phase change material (PCM) sodium acetate trihydrate, which is also widely used in commercial pocket heat pads, was evaluated as a technical solution for thermotherapy of BU [Citation55]. After initiating crystallization, the fluid PCM maintains its melting temperature of 58 °C until it has completely solidified. For thermotherapy with the heat pads, BU lesions and the surrounding skin were covered with sterile pads, tube gauze, elastic bandage and cotton as protection and to bring the skin temperature within the therapeutic range of 39–42 °C. In a prospective observational trial, six BU patients from Cameroon were successfully treated for 28–55 days with the heat packs and remained relapse-free for one and a half years after completion of treatment [Citation56]. A subsequent phase II clinical trial in Cameroon under good clinical practice conditions confirmed that thermotherapy of BU with heat packs is well tolerated and effective [Citation57]. Patients were treated daily with two heat packs, with the first one being applied in the late afternoon. Packs were changed before patients went to bed and the second bag remained in place overnight. Heat treatment continued for 42 days; it was terminated earlier if a lesion had completely closed, and was extended for up to 14 days if induration had not fully subsided [Citation57]. In addition to the specific effects on M. ulcerans viability, heat treatment also appeared to promote wound healing in general.
Since wIRA enables heat application to deeper tissue layers of the skin [Citation14], where most M. ulcerans cells reside [Citation58], without overheating superficial skin layers, its use may have the potential to bring about further improvement in the thermotherapy of BU and might reduce the duration of treatment required.
Chlamydial infections
Pathogenesis and treatment of trachoma
Chlamydiae are gram-negative, obligate intracellular bacteria with a unique biphasic developmental cycle (). In humans, C. trachomatis is the worldwide leading cause of sexually-transmitted bacterial infections as well as of infectious blindness in developing countries. Blinding trachoma is a devastating neglected tropical disease (NTD) affecting the conjunctiva/cornea of human patients, especially children. Forty-one million people suffer from active ocular C. trachomatis infection. Trachoma has led to visual impairment in about 2.2 million people, of whom 1.2 million are irreversibly blind [Citation59].
Figure 4. Developmental cycle of Chlamydia including the extracellular, infectious elementary body (EB), the intracellular replicating reticulate body (RB) and the persistent aberrant body (AB) induced by stress conditions. wIRA treatment at different timepoints of the developmental cycle has shown reductive effects on chlamydial infectivity.
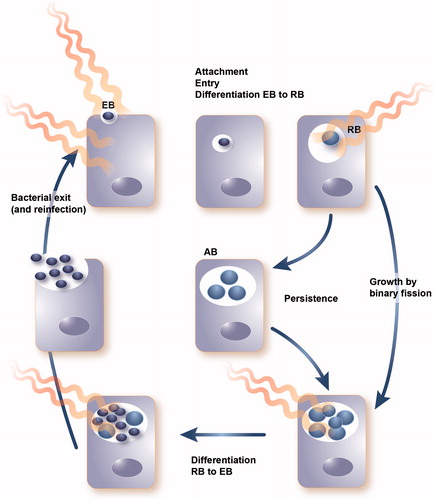
Globally, it is estimated that blinding trachoma is endemic in 50 countries, mainly in sub-Saharan Africa and the Middle East, as well as in parts of Asia and South and Central America [Citation60] (). Trachoma is associated with poor hygiene status and extreme poverty [Citation60]. The disease is spread by direct contact with ocular and nasal discharges, contact with fomites, or contact with eye-seeking flies (reviewed in [Citation61,Citation62]). One episode of infection results in self-limiting chlamydial conjunctivitis, an acute phase referred to as active trachoma [Citation63,Citation64]. Active trachoma is usually acquired in infancy in hyper-endemic regions and is mostly seen in children, progressing to eyelid scarring and blindness in adulthood [Citation65]. Repeated and/or persistent infections trigger sustained inflammation and scarring of the upper tarsal conjunctiva. Scarring and fibrosis, in turn, distort the upper eyelid and facilitate inturning of the eyelid (entropion) and eyelashes (trichiasis), causing irritation of the corneal surface and irreversible blindness due to corneal opacity (reviewed in [Citation61]). The immune response to C. trachomatis provides only partial protection and does not prevent re-infection [Citation64], which renders the development of a vaccine against trachoma unlikely.
A number of global health organizations have been working together to eliminate blinding trachoma by the year 2020 (WHO - SAFE strategy). The SAFE strategy includes: surgery for trichiasis, antibiotics for active trachoma, facial cleanliness, and environmental improvement [Citation62,Citation65]. The primary frontline antibiotic used to treat ocular chlamydial infection and to prevent trachoma is azithromycin. Current WHO recommendations constitute mass treatment with a single dose of azithromycin [Citation59]. Antibiotic treatment can cause unwanted side-effects, is expensive and can lead to antibiotic resistance [Citation64]. Although resistance to azithromycin in C. trachomatis has not been reported so far, resistance in Streptococcus pneumoniae, a major cause of morbidity and mortality worldwide, increased after azithromycin mass treatment [Citation66,Citation67]. Experiences from genital Chlamydia control programs suggest that early antimicrobial treatment interferes with the development of protective immune responses [Citation68].
Under adverse environmental conditions, developing chlamydiae may enter a state referred to as persistence, more recently named the chlamydial stress response or the aberrant (AB) RB phenotype [Citation61,Citation69,Citation70] (). The AB form is more resistant, or even refractory, to antibiotic treatment [Citation69]. Moreover, it is thought that persistence and AB formation during long-term infection also occur in vivo and that persistent infections could cause a cascade of ongoing inflammatory-induced sequelae resulting in scarring of the conjunctiva and trichiasis, which cannot be reversed by antibiotic treatment and are associated with a higher recurrent risk after ocular surgery [Citation65].
In summary, despite well-supported control programs, reported C. trachomatis cases have not reached expected declines, re-infections are a common problem and timely attainment of the SAFE strategy goal toward elimination of blinding trachoma remains questionable [Citation67,Citation71].
Basic biology and thermosensitivity of chlamydiae
The unique biphasic life cycle of chlamydiae, including the persistence state of development, is depicted in . The intracellular life cycle and the existence of two distinct development stages make it difficult to distinguish between chlamydial and host cell functions or structures, and limited knowledge is available on the temperature-sensitivity of chlamydiae. An increase in the incubation temperature to 42 °C can induce the so called heat shock or chlamydial stress response [Citation70] in C. trachomatis, which can result in complete loss of infectivity if maintained for up to 12 or 24 h [Citation72,Citation73]. Apart from these specific reports, it is unknown how chlamydiae react to hyperthermia. Thermal effects of wIRA on chlamydial infections in vitro and in vivo, in the guinea pig conjunctivitis model, have been investigated in recent studies and are summarized in sections below [Citation74–78].
wIRA and Chlamydia—in vitro investigations
In vitro studies investigating the effects of irradiation on chlamydial development usually distinguish between application of wIRA alone (780–1400 nm) or wIRA in combination with VIS ranging from 380 to 1400 nm [Citation74,Citation75,Citation76] or 595–1400 [Citation76]. For chlamydiae, wIRA/VIS combinations have shown additional beneficial effects compared to wIRA alone: VIS markedly contributes to temperature increases in the sample at high irradiation doses and extracellular chlamydial stages (EBs) are particularly sensitive to the VIS component, whereas mature chlamydial inclusions are not [Citation75]. In summarizing our previous study results, wIRA alone or wIRA/VIS combinations will be summarized as ‘wIRA’ in the following sections. For more detailed information, the reader is referred to the specific literature cited.
Initial studies [Citation74] investigated whether wIRA irradiation is able to reduce intracellular mature chlamydial inclusions and to diminish recovery of infectious EBs () in two different mammalian host cell lines using a human chlamydial strain. Since wIRA leads to temperature increase in tissue and chlamydiae might display thermosensitivity, a temperature-controlled setting in a water-bath set to 37 °C was chosen to avoid bacterial heat-shock responses or overheating of the host cells [Citation74]. In the latter study, a diminished recovery of chlamydia upon wIRA irradiation was demonstrated, without leading to the chlamydial stress response (persistence) or causing detrimental effects on host cells.
Using a temperature-controlled setting, Marti et al. [Citation74] determined a temperature rise of the intra-well temperature during the 20 min irradiation period at a dose of 370 mW/cm2. During the first 5 min of irradiation, a rise from 34.7 °C to 37.6 °C was measured, reaching a stationary phase after 10 min at 38.4 °C, and did not exceed 38.8 °C within the 20 min irradiation period. In nonirradiated controls, the temperature at the bottom of the well ranged between 35.1 and 35.9 °C within the 20 min time frame. Mimicking the temperature rise by setting the water bath to 41 °C for 20 min resulted in the same temperature profile as measured during irradiation. This temperature increase reduced the chlamydial inclusion frequency to a similar extent (0.41 inclusion per nucleus) as the single-dose irradiation (0.47 inclusion per nucleus). Therefore, Marti et al. [Citation74] concluded that thermal effects on Chlamydia due to irradiation are involved in the reduction of chlamydial infectivity.
A follow-up study [Citation75] aimed to investigate factors influencing the effects of wIRA on acute chlamydial infection, namely the impact of temperature, exposure intensity, infectious dose as well as the efficacy of the VIS component. Cultures were exposed to wIRA for 20 min in a temperature-controlled setting maintaining 37 °C. This study showed that not only thermal but also nonthermal effects may contribute to the inhibition of acute chlamydial infection. The water bath temperature was lowered to 34 °C resulting in an intra-well temperature on top of the coverslip of approximately 36 °C, which was identical to that of nonirradiated control wells at a water bath temperature of 37 °C. The reduction of IFU/mL following irradiation in a temperature-controlled setting (water bath at 34 °C) was similar to that of irradiation at a water bath temperature set at 37 °C. Regardless of the infectious dose, wIRA irradiation reduced the infectivity of EBs in an irradiation dose-dependent manner. Surprisingly, the reduction of infectivity of mature chlamydial inclusions was not only independent of the chlamydial infectious dose (as irradiation of EBs) but also remained the same regardless of the wIRA irradiation intensity [Citation75]. The latter finding suggests a contribution of host cell factors to the anti-chlamydial effect at a late stage of the chlamydial developmental cycle [Citation75].
These preliminary results encouraged us to further evaluate wIRA as a possible non-chemical treatment method for blinding trachoma by studying wIRA effects in an ocular in vitro infection model (ocular strain C. trachomatis serovar B and human conjunctival epithelial cells (HCjE)) and noninfected ocular structures in two ex vivo eye models [Citation76]. In vitro, wIRA was applied for 30 min in the temperature-controlled setting (37 °C) as described previously [Citation74,Citation75]. Irradiation with wIRA was carried out on infected HCjE cells at 40 h p.i. (mature chlamydial inclusions, first setting) or cell monolayers were irradiated at 8, 6, 3 h, and immediately before infection with EBs, which had also been either irradiated or not (second setting). A significant wIRA-induced reduction of C. trachomatis serovar B infectivity in HCjE could be observed: a single dose of wIRA reduced the number of mature chlamydial inclusions at 43 h p.i. by 35.2% (780–1400 nm) and by 53.8% (595–1400 nm). The combined irradiation of cells (four times) and EBs prior to infection had a strong synergistic effect, leading to a decrease in chlamydial infectivity of 73.5% (780–1400 nm) and 86.9% (595–1400 nm) of the control, respectively. Moreover, wIRA treatment of HCjE prior to chlamydial infection was sufficient to inhibit chlamydial infectivity, indicating the induction of potential protective effects in wIRA-treated cells possibly via increasing their cell metabolism, since wIRA neither reduced HCjE cell viability nor induced retinal damage post-treatment, thus excluding potential harmful effects. In addition, temperatures during wIRA exposure did not markedly exceed physiological eye temperatures at relevant doses (200 mW/cm2), suggesting that hyperthermia-related lesions are unlikely [Citation76].
Previous investigations [Citation74] using a triple dose irradiation of wIRA (370 mW/cm2, 380–1400 nm) for 20 min of C. trachomatis-infected HeLa cells revealed a release pattern of a subset of pro-inflammatory cytokines and chemokines after chlamydial infection, irradiation, and the combination of both, but not in a synergistic manner. These findings were further evaluated in a subsequent study [Citation77] showing that wIRA-induced reduction of chlamydial infectivity is independent of targeted cytokine inhibition of IL-6, IL-8, or RANTES/CCL5, either by pharmacological inhibition or by transfection with corresponding siRNAs. Conversely, wIRA acts reductively on chlamydial infection without influencing the host cytokine/humoral immune response, as recently confirmed by a guinea pig inclusion conjunctivitis study in vivo [Citation79].
In vivo data and translation to the clinical setting
Convincing in vitro results demonstrating that exposure of Chlamydia-infected cells to wIRA irradiation reduces: (1) the number of chlamydial inclusions that develop, and (2) the production of infectious chlamydiae without any negative impacts on host cell viability [Citation74,Citation75] prompted us to further investigate the effect of wIRA in an experimental animal model. The guinea pig inclusion conjunctivitis induced by experimental C. caviae infection is an established model of trachoma-like disease [Citation80,Citation81] and was applied to test the effect of wIRA in an ocular in vivo model [Citation79]. To study the acute course of a primary infection, Hartley strain guinea pigs were infected with 1 × 106 inclusion-forming units (IFUs) per eye of C. caviae directly into the conjunctival sac on day 0. In infected animals, wIRA was applied for 30 min on day 2 (single treatment) and on days 2 and 4 (double treatment) p.i. [Citation79]. In this model, wIRA reduced the pathology score on days 7 and 14 p.i. and the chlamydial load on days 2, 4, 7 and 14 p.i. in comparison with C. caviae-infected, nonirradiated control animals. On day 21 p.i., the number of chlamydial inclusions was reduced in singly and doubly treated animals, and PMNs were significantly reduced in the eyelids of animals treated on days 2 and 4 p.i. In general, a double treatment with wIRA/VIS (days 2 and 4 p.i.) was more efficient than a single treatment on day 2 p.i. The IFN-γ values in tear washes (days 0, 4, 7, 14, and 21 p.i.) and C. caviae-specific IgG antibodies in serum samples (days 0 and 21 p.i.) did not differ between infected, non-irradiated and infected, irradiated (single or double treatment) animals, but followed the course of C. caviae infection and the pathology scores. The latter is in line with recent in vitro findings [Citation77] showing that certain cytokines and chemokines are not involved in the wIRA working mechanism. As seen in vitro [Citation74,Citation76,Citation77], it is likely that nonthermal as well as thermal effects play a role in reducing chlamydial burden and infectivity. The latter would consist of a temperature rise in the conjunctival lid surfaces and on the cornea, possibly also affecting other ocular structures. Preliminary unpublished investigations of ocular wIRA application in anesthetized pigs were conducted, irradiating them under general anesthesia through closed eyelids with a temperature probe placed on the cornea. The temperatures measured on the cornea of these six pigs ranged from 36.8 to 38.6 °C with a mean value of 37.5 °C. In the guinea pig study [Citation79], probes were inserted between the conjunctiva and the cornea to measure the temperature during wIRA/VIS treatment (30 min, 210 mW/cm2). Temperatures ranged from 37.5 to 37.9 °C on day 2 and from 37.5 to 37.8 °C on day 4 of wIRA treatment, indicating that heat produced by wIRA was mainly absorbed by the guinea pig lid tissue.
wIRA-induced hyperthermia is of benefit in treating chlamydial infections in the conjunctiva, but might be critical when other ocular structures are reached. Recent data from our laboratory showed that wIRA (100 mW/cm2, 595–1400 nm for 30 min) does not activate markers of cytotoxicity such as phosphorylated p38, Akt, Erk, or SAPK/JNK in the highly photo- and heat-sensitive retinae of adult and postnatal (10 days old) mice at 0, 3, and 24 h after irradiation at 37 °C [Citation76]. The latter study also investigated the vitreal temperature, which can affect the neighboring thermo- and photosensitive retina, in an ex vivo perfused pig eye model in which the vitreal temperature was adjusted to 34 °C (physiological temperature). The temperature during irradiation was measured in the middle of the vitreous body or on top of the cornea during a 30 min irradiation by wIRA at different doses and wavelengths [Citation76]. The vitreal temperature increased to 38.4 °C after 30 min with an irradiation dose of 370 mW/cm2, which was, however, a higher dose than that used in the aforementioned guinea pig model (210 mW/cm2) [Citation79].
In human ophthalmology, temperatures of up to 40 °C are induced at the internal lid surface by commercial heat therapy devices (e.g., LipiFlow) for melting obstructed meibum in patients with recalcitrant Meibomian gland dysfunction [Citation82]. However, it is well known that the retina and the adjacent retinal pigment epithelium are sensitive to changes in temperature and intraocular pressure. Thus, the risk for damage to the neural visual system increases with long irradiation durations (e.g., 30 min). Therefore, ocular safety studies in a guinea pig or pig experimental model will be needed to exclude any deleterious effect of wIRA on sensitive ocular structures such as the cornea, lens, vitreous body, and retina. Moreover, short-term irradiation protocols (5 or 15 min) including multiple doses would be preferable to apply in the target population (mainly children).
Discussion, conclusion, future directions
Drawbacks in treatment of NTDs
Blinding trachoma and BU are devastating NTDs affecting the conjunctiva/cornea and skin/subcutaneous tissue of human patients, in particular children. Trachoma and BU have similar geographical distributions and are most prevalent in Sub-Saharan Africa (). Trachoma, caused by ocular serovars of C. trachomatis, and BU, induced by M. ulcerans, is commonly treated with antibiotics. The SAFE strategy to eradicate blinding trachoma by 2020 includes a single dose of oral azithromycin [Citation59]. To date, antibiotic treatment as part of the SAFE strategy has not produced the desired results, leaving a considerable number of people still infected despite therapy [Citation71,Citation83]. Antimicrobial treatment using rifampicin and streptomycin for eight weeks is the current WHO-recommended therapy for BU [Citation45]. BU treatment with rifampicin and streptomycin is associated with severe side effects and cannot be easily decentralized because streptomycin has to be injected daily. The drawbacks of antibiotic treatments are manifold, and growing antibiotic resistance is a worldwide threat [Citation84]. Development of a safe and effective C. trachomatis vaccine remains an ultimate goal, but short-term immunity and immunopathology induced by vaccination remains a critical hurdle [Citation85]. Vaccine development for BU is hampered by incomplete knowledge of the nature of immune defenses needed to control the infection, meaning that no vaccine candidate is currently in advanced development. Therefore, alternative nonchemical therapeutic strategies are urgently needed to combat these diseases caused by intracellular bacteria.
Thermotherapy using wIRA as promising treatment option
Thermotherapy has been shown to be clinically effective in several skin diseases, such as BU and leishmaniasis, but is not yet broadly applied. wIRA is a noninvasive source for safe thermal therapy and, in contrast to many drug treatments, is free of severe side effects requiring hospital-based treatment. Using wIRA would allow applying treatment at peripheral health centers close to patient communities, which would substantially lower the economic burden for the affected impoverished populations in the endemic trachoma and BU areas. wIRA radiators are easy to use devices that do not require a particular environment or skilled medical personnel and could be placed in remote areas (villages) without a power supply and used as an ambulant therapy. The irradiation device is affordable and does not need any expensive maintenance [Citation86]. Limited access to hospitals and lack of resources to finance transport and costs of hospital stays are a major cause of the delayed reporting of patients to the formal health systems. Therefore, there is an urgent requirement to bring treatments closer to the affected communities. wIRA treatment is highly suitable for achieving this goal: its application is not harmful, which renders it practical in settings where rapid laboratory reconfirmation of clinical diagnosis is not readily available. wIRA is applicable in areas without electricity through rechargeable batteries and a portable generator. The mobile wIRA irradiators are easily manageable and almost maintenance-free medical devices.
Previous in vitro, ex vivo, and animal model data have demonstrated the efficacy of wIRA against chlamydial and mycobacterial infections. Thus, the next logical step would be to implement wIRA as an innovative treatment for trachoma and BU in affected individuals. As a non-chemical treatment, wIRA does not interact with other therapeutic strategies such as antimicrobial substances or vaccines. Thus, it can be applied as an exclusive treatment, but also in combination with established antibiotic treatment strategies. The application of wIRA might thus reduce antibiotic use and the incidence of antimicrobial resistance. Inappropriate or inconsistent antibiotic therapy is a primary factor leading to resistance, which is particularly problematic in developing nations where antimicrobials can be acquired only periodically and where use of expired or counterfeit medications is common [Citation87]. wIRA could represent an important aid in overcoming such limitations. Moreover, wIRA might be suitable not only to treat existing infections but also to reduce re-infections, transmission of disease and disease progression.
Future directions
Chlamydia trachomatis infection occurs mainly in children aged 1–5 years, who act as a reservoir for the bacterium and will be the target population for treatment. In children, potential wIRA treatment is best applicable as a single short-term irradiation. Therefore, the efficiency of single short-term irradiations (5 or 15 min) needs to be evaluated in future experiments.
Chronic/persistent C. trachomatis infections may contribute to prolonged, chronic inflammation, fibrosis, and scarring through continuing stimulation of the host immune system, as seen in trachoma [Citation65]. The conjunctival epithelium is an immunocompetent tissue in which infection induces IFN-γ, increasing the likelihood that IFN-γ-mediated persistence/stress is also present in C. trachomatis-infected ocular tissues [Citation88,Citation89]. Previous in vitro studies have not addressed chronic/persistent chlamydial infection. Therefore, it remains to be investigated whether wIRA is effective against chronic/persistent C. trachomatis infection and if it is also able to reduce EB recovery after persistence.
In conclusion, once successfully implemented for BU and/or trachoma, it might be possible to extend the spectrum of wIRA-treatable tropical skin diseases, since there is evidence that other pathogens, such as the obligate intracellular protozoa of the genus Leishmania are sensitive to heat [Citation90]. Such an integrated approach is in line with the new WHO strategy to promote an integrated strategy for skin-related NTDs that require active detection, treatment and control [Citation91].
Disclosure statement
No potential conflict of interest was reported by the author(s).
Additional information
Funding
References
- MacLean RC, San Millan A. The evolution of antibiotic resistance. Science. 2019;365(6458):1082–1083.
- Chopra R, Levi-Polyachenko N, Smeltzer MS. Introduction to the special issue on thermal therapy and infectious diseases. Int J Hyperthermia. 2018;34(2):133–134.
- Gazel D, Yılmaz M. Are infectious disease and microbiology new fields for thermal therapy research? Int J Hyperthermia. 2018;34(7):918–924.
- Ibelli T, Templeton S, Levi-Polyachenko N. Progress in utilizing hyperthemia for mitigating bacterial infections. Int J Hyperthermia. 2018;34(2):144–156.
- Wardlow R, Bing C, VanOsdol J, et al. Targeted antibiotic delivery using low temperature-sensitive liposomes and magnetic resonance-guided high-intensity focused ultrasound hyperthermia. Int J Hyperthermia. 2016;32(3):254–264.
- Ricker EB, Aljaafari H, Bader TM, et al. Thermal shock susceptibility and regrowth of Pseudomonas aeruginosa biofilms. Int J Hyperthermia. 2018;34(2):168–176.
- Wardlow R, Sahoo K, Dugat D, et al. High intensity focused ultrasound (HIFU) heating improves perfusion and antimicrobial efficacy in mouse Staphylococcus abscess. Ultrasound Med. Biol. 2018; 44(4):909–914.
- Hartel M, Illing P, Mercer JB, et al. Therapy of acute wounds with water-filtered infrared-A (wIRA). GMS Krankenhhyg Interdiszip. 2007;2(2):Doc53.
- Hellige G, Becker G, Hahn G. Temperaturverteilung und Eindringtiefe wassergefilterter Infrarot-A-Strahlung. In Vaupel P, Krüger W, editors. Wärmetherapie mit wassergefilterter Infrarot-A-Strahlung. Grundlagen und Anwendungsmöglichkeiten. Stuttgart: Hippokrates Verlag; 1995.
- Vaupel P., Krüger W. (Eds.). Wärmetherapie mit wassergefilterter Infrarot-A-Strahlung. Stuttgart: Hippokrates; 1992.
- Vaupel P, Kelleher DK, Krüger W. Water-filtered infrared-A-radiation: a novel technique to heat superficial tumors. Strahlenther. Onkol. 1992;168:633–639.
- Vaupel P, Piazena H, Müller W, et al. Biophysical and photobiological basics of water-filtered infrared-A hyperthermia of superficial tumors. Int J Hyperthermia. 2018;35(1):26–36.
- Jung T, Höhn A, Lau AM, et al. An experimental setup for the measurement of nonthermal effects during water-filtered infrared a-irradiation of mammalian cell cultures. Photochem Photobiol. 2012;88:371–380 doi: 10.1111/j.1751-1097.2011.01072.x.
- Hartel M, Hoffmann G, Wente MN, et al. Randomized clinical trial of the influence of local water-filtered infrared A irradiation on wound healing after abdominal surgery. Br J Surg. 2006;93(8):952–960.
- Hoffmann G. Principles and working mechanisms of water-filtered infrared-A (wIRA) in relation to wound healing. GMS Krankenhhyg. Interdiszip. 2007;2(2):Doc54.
- Künzli BM, Liebl F, Liebl P, et al. Impact of preoperative local water-filtered infrared a irradiation on postoperative wound healing: a randomized patient-and observer- blinded controlled clinical trial). Ann Surg. 2013;258:887–894 doi: 10.1097/SLA.0000000000000235.
- Von Felbert V, Schumann H, Mercer JB, et al. Therapy of chronic wounds with water-filtered infrared-A (wIRA). GMS Krankenhyg. Interdiszip. 2007;2(2):Doc52.
- Reilly J, Twaddle S, McIntosh J, et al. An economic analysis of surgical wound infection. J Hosp Infect. 2001;49(4):245–249.
- Weber WP, Marti WR, Zwahlen M, et al. The timing of surgical antimicrobial prophylaxis. Ann Surg. 2008;247(6):918–926.
- Plattner O, Akça O, Herbst F, et al. The influence of 2 surgical bandage systems on wound tissue oxygen tension. Arch Surg. 2000;135(7):818–822.
- Buggy DJ, Doherty WL, Hart EM, et al. Postoperative wound oxygen tension with epidural or intravenous analgesia: a prospective, randomized, single-blind clinical trial. Anesthesiology. 2002;97(4):952–958.
- Hopf HW, Hunt TK, West JM, et al. Wound tissue oxygen tension predicts the risk of wound infection in surgical patients. Arch Surg. 1997;132(9):997–1004.
- Khan AA, Banwell PE, Bakker MC, et al. Topical radiant heating in wound healing: an experimental study in a donor site wound model. Int Wound J. 2004;1(4):233–240.
- Hoffmann G, Hartel M, Mercer JB. Heat for wounds - water-filtered infrared-A (wIRA) for wound healing - a review. Ger Med Sci. 2016;14:Doc08.
- Melling AC, Ali B, Scott EM, et al. Effects of preoperative warming on the incidence of wound infection after clean surgery: a randomised controlled trial. Lancet. 2001;358(9285):876–880.
- Applegate LA, Scaletta C, Panizzon R, et al. Induction of the putative protection protein ferritin by infrared radiation: implications in skin repair. Int J Mol Med. 2000;5:247–251.
- Danno K, Mori N, Toda K, et al. Near-infrared irradiation stimulates cutaneous wound repair: laboratory experiments on possible mechanisms. Photoderm Photoimm Photomed. 2001;17(6):261–265.
- Wysocki S. Gewebespiegel verschiedener Antibiotika beim Menschen. Infection. 1976;4(S2):S115–S122.
- Aljasir A, Pierson T, Hoffmann G, et al. Management of donor site infections in split-thickness skin graft with water-filtered infrared-A (wIRA). GMS Interdiscip Plast Reconstr Surg DGPW. 2018;7:Doc03.
- Notter M, Piazena H, Vaupel P. Hypofractionated re-irradiation of large-sized recurrent breast cancer with thermography-controlled, contact-free water-filtered infra-red-A hyperthermia: a retrospective study of 73 patients. Int J Hyperth. 2017;33:227–236. doi: 10.1080/02656736.2016.1235731
- Piazena H, Müller W, Pendl W, et al. Thermal field formation during wIRA-hyperthermia: temperature measurements in skin and subcutis of piglets as a basis for thermotherapy of superficial tumors and local skin infections caused by thermosensitive microbial pathogens. Int J Hyperthermia. 2019;36(1):937–951.
- Junghanss T, Johnson RC, Pluschke G, Mycobacterium ulcerans disease. In Farrar J, Hotez PJ, Junghanss T, Kang G, Lalloo D, White NJ, editors. Manson’s tropical diseases. 23rd ed. Edinburgh: Saunders, 519–531; 2014.
- Röltgen K, Pluschke G. Buruli ulcer: history and disease burden. In Röltgen K, Pluschke G, editors. Buruli ulcer. Cham, Switzerland: Springer Nature. 2019.
- MacCallum P, Tolhurst JC, Buckle G, et al. A new mycobacterial infection in man. J Pathol. 1948;60(1):93–122.
- Eddyani M, Portaels F. Survival of Mycobacterium ulcerans at 37 degrees C. Clin Microbiol Infect. 2007;13(10):1033–1035.
- Feldman WH, Karlson AG, Herrick JF. Mycobacterium ulcerans; pathogenesis of infection in mice, including determinations of dermal temperatures. Am J Pathol. 1957;33(6):1163–1179.
- Guenin-Macé L, Ruf MT, Pluschke G, et al. Mycolactone: more than just a cytotoxin. In Röltgen K, Pluschke G, editors. Buruli ulcer. Springer Nature; 2019.
- Hall B, Simmonds R. Pleiotropic molecular effects of the Mycobacterium ulcerans virulence factor mycolactone underlying the cell death and immunosuppression seen in Buruli ulcer. Biochem Soc Trans. 2014;42(1):177–183.
- Ruf MT, Steffen C, Bolz M, et al. Infiltrating leukocytes surround early Buruli ulcer lesions, but are unable to reach the mycolactone producing mycobacteria. Virulence. 2017;8(8):1918–1926.
- Scherr N, Pluschke G. Buruli ulcer. In Swinney D, Pollastri M, editors. Neglected tropical diseases: drug discovery and development. Weinheim, Germany: Wiley‐VCH Verlag GmbH; 2019.
- Omansen TF, van der Werf TS, Phillips RO. Antimicrobial treatment of Mycobacterium ulcerans infection. In Röltgen K, Pluschke G, editors. Buruli ulcer. Cham, Switzerland: Springer Nature; 2019.
- Cornet L, Richard-Kadio M, N’Guessan HA, et al. Treatment of Buruli’s ulcers by excision-graft. Bull Soc Pathol Exot. 1992;85(5):355–358.
- Teelken MA, Stienstra Y, Ellen DE, et al. Buruli ulcer: differences in treatment outcome between two centres in Ghana. Acta Trop. 2003;88(1):51–56.
- Ackumey MM, Gyapong M, Pappoe M, et al. Socio-cultural determinants of timely and delayed treatment of Buruli ulcer: implications for disease control. Infect Dis Poverty. 2012;1(1):6.
- WHO - Provisional guidance on the role of specific antibiotics in the management of Mycobacterium ulcerans disease (Buruli ulcer). 2004. Available from: https://apps.who.int/iris/handle/10665/68839.
- WHO - Report from the Meeting of the Buruli ulcer Technical Advisory Group. [cited Mar 2017]. Available from: http://www.who.int/neglected_diseases/events/WHO_BU_TAG_2017_report.pdf.
- Scherr N, Bieri R, Thomas SS, et al. Targeting the Mycobacterium ulcerans cytochrome bc1:aa3 for the treatment of Buruli ulcer. Nat Commun. 2018;9(1):5370.
- Asiedu K, Scherpbier R, Raviglione M. (Eds.). Buruli ulcer: Mycobacterium ulcerans infection. Yamoussoukro Declaration of Buruli Ulcer. WHO – Department of disease control, prevention and eradication. 2000.
- Bratschi MW, Bolz M, Grize L, et al. Primary cultivation: factors affecting contamination and Mycobacterium ulcerans growth after long turnover time and clinical specimens. BMC Infect Dis. 2014;14(1):636.
- Scherr N, Röltgen K, Witschel M, et al. Screening of antifungal azole drugs and agrochemicals with an adapted Alamar Blue-based assay demonstrates antibacterial activity of croconazole against Mycobacterium ulcerans. Antimicrob Agents Chemother. 2012;56(12):6410–6413.
- Van Rhoon G. Is CEM43 still a relevant thermal dose parameter for hyperthermia treatment monitoring?. Int J Hyperthermia. 2016;32(1):50–62.
- Schütte D, Pluschke G. Immunosuppression and treatment-associated inflammatory response in patients with Mycobacterium ulcerans infection (Buruli ulcer). Expert Opin Biol Ther. 2009;9(2):187–200.
- Schütte D, Umboock A, Pluschke G. Phagocytosis of Mycobacterium ulcerans in the course of rifampicin and streptomycin chemotherapy in Buruli ulcer lesions. Br J Dermatol. 2009;160(2):273–283.
- Meyers WM, Shelly WM, Connor DH. Heat treatment of Mycobacterium ulcerans infections without surgical excision. Am J Trop Med Hyg. 1974;23(5):924–929.
- Braxmeier S, Hellmann M, Beck A, et al. Phase change material for thermotherapy of Buruli ulcer: modelling as an aid to implementation. J Med Eng Technol. 2009;33(7):559–566.
- Junghanss T, Um Boock A, Vogel M, et al. Phase change material for thermotherapy of Buruli ulcer: a prospective observational single centre proof-of-principle trial. PLoS Negl Trop Dis. 2009;3(2):e380.
- Vogel M, Bayi PF, Bratschi MW, et al. Local heat application for the treatment of Buruli ulcer: results of a phase II open label single Center non comparative clinical trial. Clin Infect Dis. 2016;62(3) :342–350.
- Ruf MT, Bolz M, Vogel M, et al. Spatial distribution of Mycobacterium ulcerans in Buruli ulcer lesions: implications for laboratory diagnosis. PLoS Negl Trop Dis. 2016;10(6):e0004767.
- WHO - Trachoma. [cited 2017 Mar 31]. Available from: http://www.who.int/topics/trachoma/en/
- Wright HR, Turner A, Taylor HR. Trachoma. Lancet. 2008;371(9628):1945–1954.
- Leonard CL, Borel N. Chronic chlamydial diseases: from atherosclerosis to urogenital infections. Curr Clin Micro Rpt. 2014;1(3-4):61–72.
- Wolle MA, West SK. Ocular Chlamydia trachomatis infection: elimination with mass drug administration. Expert Rev Anti Infect Ther. 2019;17(3):189–200.
- Burton MJ, Mabey D. The global burden of trachoma: a review. PLoS Negl Trop Dis. 2009;3(10):e460.
- Hu VH, Holland MJ, Burton MJ. Trachoma: protective and pathogenic ocular immune responses to Chlamydia trachomatis. PLoS Negl Trop Dis. 2013;7(2):e2020.
- Taylor HR, Burton MJ, Haddad D, et al. Trachoma. Lancet. 2014;384(9960):2142–2152.
- Ho DK, Sawicki C, Grassly N. Antibiotic resistance in Streptococcus pneumoniae after azithromycin distribution for trachoma. J Trop Med. 2015;2015:1–8.
- O'Brien KS, Emerson P, Hooper PJ, et al. Antimicrobial resistance following mass azithromycin distribution for trachoma: a systematic review. Lancet Infect Dis. 2019;19(1):e14–e25.
- Brunham RC, Rekart ML. The arrested immunity hypothesis and the epidemiology of Chlamydia control. Sex Transm Dis. 2008;35(1):53–54.
- Wyrick PB. Chlamydia trachomatis persistence in vitro: an overview. J Infect Dis. 2010;201(S2):88–95.
- Bavoil PM. What’s in a word: the use, misuse, and abuse of the word “persistence” in Chlamydia biology. Front Cell Infect Microbiol. 2014;4:27.
- Lavett DK, Lansingh VC, Carter MJ, et al. Will the SAFE strategy be sufficient to eliminate trachoma by 2020? Puzzlements and possible solutions. Sci World J. 2013;2013:1–18.
- Mukhopadhyay S, Miller RD, Sullivan ED, et al. Protein expression profiles of Chlamydia pneumoniae in models of persistence versus those of heat shock stress response. Infect Immun. 2006;74(7):3853–3863.
- Hogan RJ, Mathews SA, Mukhopadhyay S, et al. Chlamydial persistence: beyond the biphasic paradigm. Infect Immun. 2004;72(4):1843–1855.
- Marti H, Koschwanez M, Pesch T, et al. Water-filtered infrared A irradiation in combination with visible light inhibits acute chlamydial infection. PLoS One. 2014;9(7):e10223.
- Marti H, Blenn C, Borel N. The contribution of temperature, exposure intensity and visible light to the inhibitory effect of irradiation on acute chlamydial infection. J Photochem Photobiol B. 2015;153:324–333.
- Rahn C, Marti H, Frohns A, et al. Water-filtered infrared A reduces chlamydial infectivity in vitro without causing ex vivo eye damage in pig and mouse models. J Photochem Photobiol B. 2016;165:340–350.
- Kuratli J, Pesch T, Marti H, et al. Water filtered infrared A and visible light (wIRA/VIS) irradiation reduces Chlamydia trachomatis infectivity independent of targeted cytokine inhibition. Front Microbiol. 2018;9:2757.
- Kuratli J, Borel N. Perspective: Water-filtered infrared-A-radiation (wIRA) - Novel treatment options for chlamydial infections? Front Microbiol. 2019;10:1053.
- Inic-Kanada A, Stojanovic M, Barisani-Asenbauer T, et al. Water-filtered Infrared A and visible light (wIRA/VIS) treatment reduces chlamydial load and pathology score in a guinea pig model of inclusion conjunctivitis. Swiss Society for Microbiology (SGM, SSM) Annual Meeting 2019, Zurich, 2019.
- Belij-Rammerstorfer S, Inic-Kanada A, Stojanovic M, et al. Infectious dose and repeated infections are key factors influencing immune response characteristics in guinea pig ocular chlamydial infection. Microbes Infect. 2016;18(4):254–262.
- Rank RG, Whittum-Hudson JA. Animal models for ocular infections. Methods Enzymol. 1994;235:69–83.
- Kenrick CJ, Alloo SS. The limitation of applying heat to the external lid surface: a case of recalcitrant meibomian gland dysfunction. Case Rep Ophthalmol. 2017;8(1):7–12.
- West SK, Moncada J, Munoz B, et al. Is there evidence for resistance of ocular Chlamydia trachomatis to azithromycin after mass treatment for trachoma control?. J Infect Dis. 2014;210(1):65–71.
- Nadimpalli M, Delarocque-Astagneau E, Love DC, et al.,; Bacterial Infections and antibiotic-Resistant Diseases among Young children in low-income countries (BIRDY) Study Group. Combating global antibiotic resistance: emerging one health concerns in lower- and middle-income countries. Clin Infect Dis. 2018;66(6):963–969.
- Mabey D, Hu V, Bailey RL, et al. Towards a safe and effective chlamydial vaccine: lessons from the eye. Vaccine. 2014;32(14):1572–1578.
- Hydrosun – Healing derived from nature. [cited 2019 Dec 12]. Available from: https://www.hydrosun.de/en
- Ouedraogo AS, Jean Pierre H, Bañuls AL, et al. Emergence and spread of antibiotic resistance in West Africa: contributing factors and threat assessment. Med Sante Trop. 2017;27(2):147–154.
- Rapoza PA, Tahija SG, Carlin JP, et al. Effect of interferon on a primary conjunctival epithelial cell model of trachoma. Invest Ophthalmol Vis Sci. 1991;32(11):2919–2923.
- Bobo L, Novak N, Mkocha H, et al. Evidence for a predominant proinflammatory conjunctival cytokine response in individuals with trachoma. Infect Immun. 1996;64(8):3273–3279.
- Aronson NE, Wortmann GW, Byrne WR, et al. randomized controlled trial of local heat therapy versus intravenous sodium stibogluconate for the treatment of cutaneous Leishmania major infection. PLoS Negl Trop Dis. 2010;94(3):e628.
- WHO - Skin diseases: integrated treatment to promote public health benefits. [cited 2017 Mar 31]. Available from: http://www.who.int/neglected_diseases/news/Skin_diseases_integrated_treatment/en/.