Abstract
Hyperthermia therapy (HT) of cancer is a well-known treatment approach. With the advent of new technologies, HT approaches are now important for the treatment of brain tumors. We review current clinical applications of HT in neuro-oncology and ongoing preclinical research aiming to advance HT approaches to clinical practice. Laser interstitial thermal therapy (LITT) is currently the most widely utilized thermal ablation approach in clinical practice mainly for the treatment of recurrent or deep-seated tumors in the brain. Magnetic hyperthermia therapy (MHT), which relies on the use of magnetic nanoparticles (MNPs) and alternating magnetic fields (AMFs), is a new quite promising HT treatment approach for brain tumors. Initial MHT clinical studies in combination with fractionated radiation therapy (RT) in patients have been completed in Europe with encouraging results. Another combination treatment with HT that warrants further investigation is immunotherapy. HT approaches for brain tumors will continue to a play an important role in neuro-oncology.
Introduction
Hyperthermia is a term derived from the Greek terms υπέρ/ypér, meaning ‘above’, ‘more’ or ‘over’ and θερμός/thermós meaning ‘hot’. Hyperthermia therapy (HT) is most often used to describe the therapeutic technique of induced temperature increase through thermal energy delivery [Citation1,Citation2]. Extreme hyperthermia, also known as thermal ablation, refers to the local application of thermal energy resulting in very high tissue temperatures which induces irreversible injury [Citation3]. Both hyperthermia and thermal ablation procedures have been used for the treatment of many different types of cancers including breast, melanoma, liver, kidney, lung, cervical, bone, lymph node metastasis, and head and neck tumors [Citation3,Citation4].
In this article, we review the literature on the different evolving thermal ablative procedures and HT modalities utilized for the treatment of brain tumors by discussing relevant preclinical and clinical data that have shaped the current state of HT in the field of neuro-oncology. We focus on the burgeoning clinical literature on thermal ablation laser interstitial therapy (LITT) and the rapidly growing literature on magnetic hyperthermia (MHT) and nanoparticle-mediated photothermal therapy (PTT). Finally, we review outcome data with other modes of HT utilizing microwaves, ultrasound, radiofrequency and light energy as well as novel HT devices employed in the clinical setting.
Physiological response to HT
HT is categorized into whole body HT (WHT), regional HT (RHT) and local HT (LHT) [Citation1]. WHT or total body hyperthermia (39.5–42 °C) causes systemic vasodilation, endocrine and immune changes [Citation5]. WHT leads to increased VEGF, IL1 and IL6 expression, neutrophil production and neutrophil tumor infiltration [Citation6]. HT should be distinguished from fever, as during HT any core body temperature increase will not affect the hypothalamic temperature set point [Citation4,Citation7]. RHT is achieved through an external heating device and refers to the heating of a localized tumor and its healthy surrounding tissue [Citation8]. RHT aims for a heterogeneous temperature distribution (39 − 43 °C) and leads to heat-dependent physiological changes implicating metabolic and tumor vasculature alterations [Citation6]. Tumor tissue with lower perfusion compared to the surrounding normal tissue is more sensitive than healthy tissue to increased temperatures, potentially due to the reduced heat-dissipating ability of tumor tissue [Citation9]. Moreover, the lower than normal pH of the interstitial space of tumors, renders tumor tissue more sensitive to HT when compared to healthy tissue leading to targeted tumor cell death. Nevertheless, to the best of our knowledge, data related to the underlying targeted cancer death have not been reported [Citation9]. For the treatment of brain tumors, HT is utilized locally (LHT) and the temperature increase is mainly confined to the target lesion whereas the core body temperature and the temperature of the surrounding brain parenchyma remain largely unchanged [Citation10]. LHT can display antineoplastic effects after a local temperature increase to 40–44 °C [Citation11].
Cellular response to HT
HT can lead to intracellular and extracellular heat related changes that may facilitate cell necrosis and/or apoptosis [Citation3,Citation12,Citation13]. Specifically, HT results in distortion of the cell cytoskeleton components, disruption of cell membrane permeability and increase in intracellular sodium levels [Citation11,Citation14]. Increased temperatures induce a number of mitochondrial structural alterations consistent with mitochondrial injury [Citation15,Citation16]. Heat cell injury following HT, may result in a significant decrease of intra- and extracellular pH [Citation14], and denaturation of histones, chromatin as well as intracellular enzymes implicated in the regulation of cell cycle and apoptosis [Citation17,Citation18]. Moreover, HT induces alterations on the expression level with irregular RNA synthesis and damage of the Golgi apparatus [Citation9,Citation11]. HT upregulates the expression of heat shock proteins (HSPs), a family of protein chaperons expressed in cancer cells and involved in the cellular DNA injury response [Citation19,Citation20]. Of note, HSPs seem to be involved in the resistance of cancer cells to therapies including HT. However, their specific role is yet to be unraveled [Citation7,Citation21].
HT and chemoradiosensitivity enhancement
Besides direct cellular injury, HT can lead to augmented antineoplastic effects by enhancing adjuvant therapies particularly radiation therapy (RT) and chemotherapy. A number of experimental studies have reported a synergistic interaction between HT, RT and chemotherapy [Citation22–24]. HT can enhance the effect of chemotherapy through increased substrate delivery and by rendering malignant cells more sensitive to chemotherapy. Increased local delivery of the chemotherapeutic substrate can be achieved as heat may increase regional cerebral blood flow (rCBF), enable disruption of the blood brain barrier (BBB), facilitate accumulation of carriers conjugated with chemotherapy in the tumor and modulate drug-carrier detachment [Citation25–31]. HT disrupts DNA repair mechanisms, damages ATP-binding cassette (ABC) transporters, increases intracellular drug metabolism and induces apoptotic pathways [Citation23,Citation24,Citation32–36]. When HT is applied, in addition to DNA repair disruption, the AKT signaling pathway is disrupted leading to radiosensitization. Particularly, after HT, phosphorylated AKT and kinases involved in the AKT pathway such as p70 S6K, RSK1/2 and pS6 have been found significantly reduced [Citation32,Citation37–41].
HT and immunomodulation
HT elicits innate and adaptive immune responses which may enhance its treatment effects [Citation3,Citation42,Citation43]. Studies have found that HT may increase the cytotoxic activity of natural killer (NK) and CD8+ T cells against tumor cells [Citation44,Citation45]. Preclinical studies have demonstrated that immunotherapy following HT may enhance antitumor immune responses [Citation46–49]. Check point inhibitor administration following MHT has been shown to enhance CD3+ T-cell recruitment and local tumor control in a metastatic lung tumor rodent model [Citation49]. The possibility of similar immune involvement in the central nervous system during HT has ignited an exciting new area of brain tumor research [Citation50,Citation51]. Initial preclinical data have shown that HT in combination with checkpoint inhibitors (anti-PD-L1 antibody) can enhance the antitumor effect against glioblastoma (GBM) [Citation52].
Extreme HT/thermal ablation
LITT
Magnetic resonance imaging (MRI)-guided LITT is now a standard alternative to surgical resection for certain patients with primary and metastatic brain tumors [Citation53]. Tumors that have recurred despite multiple therapies as well as radiation necrosis (RN) represent ideal candidates for LITT [Citation54,Citation55]. LITT was introduced in the late 1980s by Bown et al. [Citation56] while the first results of the application of LITT in neurological surgery where reported a few years later in 1990 [Citation57]. Early technologic constraints related to laser probe size and the inability to monitor and control thermal delivery limited the adaption of this technology for patients [Citation58,Citation59]. Recent technological advancements have led to the enthusiastic resurgence of LITT in the field of neurological surgery [Citation60–62]. Stereotactic platforms, robotic arms, neuro-navigation and optimized laser probes have allowed precise targeting of the lesion [Citation63–65]. In addition, thermal damage estimate (TDE) algorithms and models such as the Arrhenius model have successfully predicted the extent of ablation preoperatively [Citation66]. Intraoperative magnetic resonance thermometry has enabled real-time tissue damage visualization permitting accurate delivery of the prescribed thermal doses [Citation67,Citation68]. Currently, LITT is the most widely reported thermal ablation mode utilized for the treatment of brain tumors.
LITT can offer a survival benefit comparable to that of open surgery with possibly lower surgery related complications rates and decreased hospital stay [Citation69,Citation70]. LITT has been implemented in an awake setting without the requirement of general anesthesia [Citation71]. An initial stereotactic biopsy can be performed during the procedure for a tissue diagnosis. A number of studies have reported how safe and efficacious LITT is considered for both diagnosis and cytoreduction [Citation54,Citation72]. During LITT, a laser catheter probe is stereotactically inserted under image guidance into the tumor through a small burr hole () [Citation70]. Light energy is then administered to the tip and converted to heat within the tumor achieving tumor cell death due to local thermal ablation and HT of the surrounding tissues [Citation73,Citation74]. Two of the most commonly used LITT platforms include the NeuroBlate System (Monteris Medical, Inc.) and the Visualase Thermal Therapy System (Medtronic, Inc.) [Citation74–77]. The NeuroBlate platform utilizes a CO2 gas-cooled laser catheter to deliver laser energy in a temperature-controlled manner [Citation75]. The platform is connected to a MRI treatment planning software that provides real-time thermal data displaying the extent of thermal energy delivery as thermal-damage-threshold (TDT) lines [Citation75]. The target volume is heated to an equivalent of 43 °C for at least 60 min and is considered to sustain coagulation necrosis. This time period has been selected according to empirical data proposing that the degree of tissue injury after exposure to 43 °C for 10 min is halfway between that at exposures of 2 and 60 min [Citation78]. Tissue within this area may or may not undergo fatal thermal changes depending on a number of physiological factors [Citation78]. Others have reported that this area is considered to have severe damage [Citation74]. The outermost area corresponds to tissue exposed to 43 °C for at least 2 min, and is considered to have no permanent damage [Citation75]. The Visualase system utilizes a laser catheter that is connected to a pump that circulates sterile saline to prevent overheating of the catheter tip and surrounding tissues [Citation74]. Similar to the Neuroblate system, the Visualase platform is connected to MRI software displaying thermal data in real-time processed through Arrhenius model. Moreover, the platform has a safety temperature control system of automatic laser deactivation, based on predesignated temperature limits [Citation76]. In the case of infiltrative tumors, the heat energy administered through LITT induces histologic changes which can be classified in three concentrically distinct areas consisting of a central area of necrosis-ablation zone (Zone 1), and two surrounding HT zones namely an area of granulation tissue surrounding the necrosis area (Zone 2), and an outmost area with the presence of viable tumor cells (Zone 3) [Citation79]. Limitations of LITT include non-optimal treatment of tumors located adjacent to large blood vessels and/or cerebrospinal fluid (CSF) filled spaces such as ventricles or cisterns that may act as heat sinks [Citation80].
Figure 1. Brain tumor illustration demonstrating laser interstitial thermal therapy (LITT). (A) Schematic representation of intratumoral placement of laser catheter and brain tumor ablation. (B) Schematic representation of brain tumor ablation demonstrating post-LITT contrast enhancement consistent with LITT related blood brain barrier (BBB) disruption and LITT related perifocal edema.
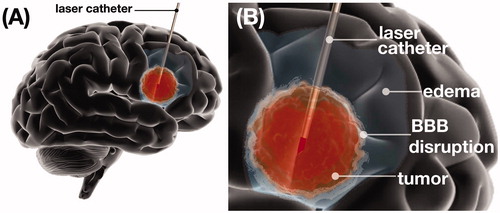
LITT and BBB
LITT can increase the permeability of the BBB, in the area surrounding the ablation zone (HT zone) (), providing a 4-week therapeutic window during which delivery of chemotherapeutic agents (CTAs) can be enhanced. Specifically, the highest permeability has been shown to take place within the first 2 weeks post-LITT while this effect wanes by weeks 4–6 [Citation81,Citation82]. It has been hypothesized that this LITT induced BBB disruption may facilitate immune cell migration through the BBB leading to an augmented immune response against tumors. Based on this finding, multiple clinical trials have been launched to investigate the potential therapeutic benefit of checkpoint kinase inhibitors (CKIs) for the treatment of high grade gliomas (HGGs) when administered during the therapeutic window of BBB disruption following LITT [Citation50,Citation51].
Brain metastasis and RN
Currently, brain metastases and RN comprise the most common pathologies LITT is used for in neuro-oncology. A literature search which was limited to the last 10 years, identified 35 original studies reporting outcome data for 646 LITT-treated patients harboring brain metastases or RN [Citation54,Citation63,Citation76,Citation77,Citation83–112]. LITT is most commonly employed for metastases that have recurred after surgical resection and RT. In one of the largest case series with LITT, brain metastases had the longest progression-free survival (PFS) compared to any other pathology (55.9 months) [Citation54]. Recurrent and incompletely ablated brain metastases have been reported as the major predicting factors for post-LITT local recurrence [Citation83]. Nevertheless, according to a recent systematic review, tumor control rates for recurrent brain metastases reach 80–100% at 3 months [Citation113]. For lesions consistent with RN, LITT is commonly employed for patients that may not be good surgical candidates (e.g. deep-seated lesion location, advanced patient age, poor patient functional status) and have failed to respond to conservative treatments with corticosteroids [Citation54,Citation70,Citation96,Citation97]. Reported outcome data for these patients are promising with local control rates reaching 75% recurrence free [Citation54].
Gliomas
LITT has been used for the treatment of HGGs in a number of studies [Citation60,Citation114–118]. A literature search over the last 10 years, identified 26 original studies reporting outcome data for 489 adult glioma patients treated with LITT [Citation54,Citation63,Citation71,Citation77–79,Citation84,Citation91,Citation95,Citation97–99,Citation101,Citation104,Citation119–131]. The most commonly reported indications included recurrent GBM (e.g. multiple craniotomies, previous RT, high risk of wound dehiscence) [Citation54,Citation63,Citation78,Citation99,Citation104,Citation122,Citation126,Citation127,Citation129,Citation130] and newly diagnosed GBM with unfavorable characteristics for surgical resection (e.g. deep seated, eloquent tumor location, advanced patient age, poor patient functional status) [Citation54,Citation63,Citation99,Citation104,Citation119,Citation120,Citation124,Citation125,Citation127,Citation129,Citation130]. For recurrent GBM tumors, a large clinical series report a median overall survival of 12 months after LITT treatment [Citation127,Citation130] while in the newly diagnosed GBM patients the median overall survival ranges between 8 and 9 months [Citation91,Citation127,Citation130]. Overall, the majority of the studies reporting data for recurrent GBM suggest that LITT is a safe treatment option which may provide a survival benefit with less operative morbidity and recovery time compared to conventional surgical resection [Citation54,Citation78,Citation104,Citation122,Citation125–127,Citation129,Citation130]. In terms of newly diagnosed GBM, most of the studies report that LITT can increase overall survival in patients that would have otherwise only be eligible for biopsy and chemoradiation [Citation54,Citation63,Citation99,Citation104,Citation119,Citation120,Citation124,Citation125,Citation127,Citation129,Citation130]. Two available meta-analyses underscore the role of LITT for HGGs located in eloquent or deep-seated areas [Citation132,Citation133]. One meta-analysis compared LITT with surgical resection outcomes of patients with newly diagnosed and recurrent HGGs located in eloquent areas [Citation132]. They reported that LITT-treated patients had increased cytoreduction rates and lower neurocognitive complication rates compared to patients who undergone surgical resection [Citation132]. Another meta-analysis included only newly diagnosed HGGs patients in their study and reported overall survival 14.2 months indicating a comparable advantage among a subset of patients who would otherwise receive biopsy and chemoradiation without any cytoreduction. Nevertheless, both studies highlight the need of clinical trials to evaluate the role of LITT in HGGs. LITT has been also employed for low grade gliomas (LGGs) with promising results [Citation63,Citation97,Citation119]. However, the lack of long-term follow-up data and controlled clinical trials precludes any assumptions regarding the role of LITT in this subset of patients.
Meningiomas
Similar to other recurrent brain tumors that have recurred despite multiple therapies, LITT has been described for patients with meningiomas that have failed surgery and RT [Citation54]. Nine original studies have been published in the literature reporting data for 29 patients harboring meningiomas [Citation54,Citation63,Citation71,Citation77,Citation84,Citation98,Citation131,Citation134,Citation135]. Although reported control rates are fairly promising for World Health Organization (WHO) grade I meningiomas, more aggressive (WHO grades II & III) meningiomas have higher recurrence rates [Citation54,Citation131,Citation135]. However, all the studies reporting outcome data for patients with LITT-treated dural-based lesions are very small with a limited number of patients.
Pediatric tumors
LITT has also been advocated for newly diagnosed and recurrent pediatric brain tumors [Citation136]. LITT-treated pathologies include: pilocytic astrocytomas [Citation136–138], ependymomas [Citation77,Citation103,Citation139], medulloblastomas [Citation136,Citation138], choroid plexus xanthogranulomas [Citation136,Citation138], subependymal giant cell astrocytomas [Citation136,Citation138,Citation140–142], gangliogliomas [Citation136,Citation138,Citation143], neuroectodermal tumors [Citation77,Citation103,Citation144], gliosis [Citation143], RN [Citation143] and hypothalamic hamartomas [Citation140]. Reported indications include deep-seated and recurrent, tumors [Citation136,Citation145]. The application of LITT for pediatric brain tumors has also shown promising outcomes, however, the number of patients treated and follow-up are limited. Futures studies are needed to further evaluate the role of LITT in pediatric neuro-oncology.
Hyperthermia modalities
MHT
MHT is a localized form of HT in which magnetic nanoparticles (MNPs) convert electromagnetic energy produced by an alternating magnetic field (AMF) into heat (). This energy conversion is thought to occur through hysteresis losses and Brownian relaxation, a process in which frictional heating is generated by the physical rotation of the magnetic particles [Citation146,Citation147]. Even though the AMF is applied nonspecifically to the body, localized heating of the region of interest can be achieved by delivering MNPs directly to the tumor area [Citation148]. This can be accomplished through targeted systemic delivery or by intratumoral administration. Newer modes of intratumoral delivery include the use of convection-enhanced delivery (CED) both in small and large animal models [Citation149–154]. Notably, CED clinical trials have reported leakage of the infused substrate in off-target areas such as the subarachnoid space, ventricles and tumor resection cavities [Citation155]. It has been shown that application of MNPs to different locations of the tumor may produce a more uniform temperature distribution across the lesion compared to laser catheter based treatment modalities such as LITT due to the distribution of MNPs which act as heat sources [Citation156]. The heating capabilities of MNPs are largely determined by their size, shape, coating/composition, magnetic properties (e.g. saturation magnetization) and their specific absorption rate (SAR) () [Citation147,Citation157]. In the context of MHT, SAR describes the power dissipation per unit of MNP in an applied AMF and largely defines their heating efficiency [Citation158]. Magnetic iron-oxide nanoparticles (MIONPs) have been studied the most for MHT due to their superior biocompatibility and heating abilities with AMF [Citation146,Citation159–162]. Other MNPs synthesized from highly magnetic metals such as manganese, cobalt, iron or nickel have been described for MHT [Citation163]. In addition to being excellent heating agents, multifunctional MIONPs also possess theranostic capabilities and can serve as contrast agents, drug-carriers and chemo-radiosensitizers [Citation24,Citation32,Citation163–169]. There are many advantages to using MHT as a treatment for brain tumors and particularly GBM. First, the penetration depth of the AMF exceeds that of other activation modalities commonly used in HT (e.g. light or acoustic waves) allowing for the heating of deeply seated tumors without having to perform skin incisions or bone removal [Citation163]. Coupled with the fact that MNPs have been shown to remain around the injection site for weeks, it is possible to perform multiple MHT sessions after single dosing [Citation153,Citation154,Citation170]. In short, MNPs are a locally confined, remotely controllable tool for performing repeated HT.
Figure 2. Schematic representation of magnetic hyperthermia therapy in the brain. (A) Alternating magnetic field is applied to the patient after local administration of magnetic nanoparticles (black spheres), generating highly localized hyperthermia. (B) Heat is produced via hysteresis losses and Brownian relaxation (a process in which frictional heating is generated by the physical rotation of the magnetic particle).
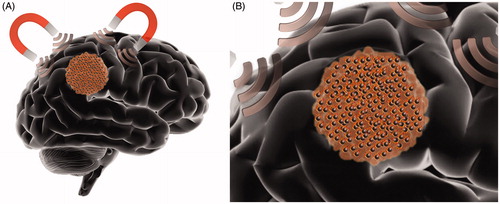
Table 1. Nanoparticle constructs utilized for magnetic hyperthermia therapy.
Advanced MNPs
The surface coating of MNPs can increase the saturation magnetization of MNPs and enhance their heating capacity. For example, aminosilane coated MNPs were reported to display HT antitumor effects after a single MHT session using iron concentrations as low as 50 μg/10 μL [Citation171]. Similarly, a stevioside coating can improve heating and enhance the antitumor effects when compared to noncoated MIONPs in vitro [Citation172]. Instead of coating the NPs, one group encapsulated magnetite (Fe3O4) NPs within copolymer-based micelles as a way of increasing the colloidal stability and controlling the size and shape of the contained MIONPs. When used to perform MHT, these magnetic polyion complex micelles reduced cell viability in glioma cells at a concentration of iron 3 orders of magnitude lower than concentrations used in previous clinical trials [Citation173]. Separately, a number of studies have used bacterial magnetosomes as an alternative for chemically synthesized MNPs. In comparison to conventional MIONPs, those studies report that bacterial magnetosomes can maintain tumor temperatures at 43–46 °C for longer, have higher antitumor efficacy in GBM cell lines, and require lower AMF amplitude to achieve target temperatures [Citation174–177]. One potential issue MHT is facing is that high concentrations of MNPs are often required to reach the clinically needed thermal dose. Silver nanocrystals (AgNPs), though not MNPs themselves, have been shown to enhance the thermo-sensitivity, radio-sensitivity and apoptosis rate of glioma cells when administered in combination with MNPs due to silver’s ability to cause reactive oxygen species (ROS) production and DNA damage. It has been proposed that using AgNPs and MNPs together may lower the concentration of MNPs necessary for effective MHT [Citation178,Citation179]. Another potential obstacle of MHT application is that internalization of MNPs has been reported to restrict Brownian motion and causes particle aggregation, both of which can lower the SAR and cause diminished heating [Citation180–182]. To counteract this phenomenon, others have designed flower-like manganese-doped superparamagnetic iron-oxide nanoparticles (SPIONPs) functionalized with glioma specific αvβ3-integrin-ligands. Due to the unique shape and the doped-manganese, they were able to maximize SAR and induce significant intracellular heating and cell death mainly through non-apoptotic pathways [Citation183]. However, other groups have reported that the cellular uptake of MNPs may be beneficial. In a recent study, MIONPs were conjugated with calcium phosphate and/or hydroxyapatite as a way of increasing their uptake by GBM cells. These internalized MIONPs reduced cell viability after MHT by a greater extent than their unconjugated counterparts that remained in the extracellular space [Citation184].
Targeted delivery
Conjugated MNPs with cancer-specific moieties for targeted heating are currently being studied. MIONPs conjugated with HSP 70 were shown to selectively target CD40 receptors and decrease tumor mass in rat C6 glioma tumors when given intravenously [Citation185]. Others have conjugated MNPs with anti-HER2 aptamers that required a 90-fold lower dose to achieve the same results as unconjugated NPs when performing MHT on HER-2 expressing cancer cells [Citation186]. Multiple other MNPs conjugated with compounds specific for glioma targeting, such as c(RGDyK), cetuximab and neuropilin-1-targeted peptide were also shown to enhance MHT effects in vivo [Citation154,Citation187–189]. Loading of MNPs in vehicles that may cross the BBB may permit better targeting of tumors after systemic administration. MIONPs loaded onto targeted exosomes have been shown to cross the BBB and provide potent antitumor effects [Citation189]. Others have demonstrated targeted delivery across the BBB by loading MIONPs into liposomes conjugated with the GBM specific cell-penetrating peptide, P1NS and the anti-GBM antibody, tenascin-C, to the liposomal surface [Citation190].
Chemotherapy
HT has been proposed as a potential chemosensitizer in the brain due to HT-induced disruption of the BBB, increased blood flow and interference with DNA repair mechanisms [Citation148]. The combination of MHT with chemotherapy may result in a more profound decrease in cell viability compared to chemotherapy or MHT monotherapy supporting the hypothesis of a synergistic effect between MHT and chemotherapy [Citation191–197]. MNPs and CTAs can be delivered together using polymers [Citation192,Citation193,Citation198], liposomes [Citation190,Citation191] or nanovectors made of both polymers and lipids [Citation194] to minimize off-target toxicity, facilitate their cellular internalization and ensure their simultaneous delivery to the target site. Polymeric conjugates and carriers (often made from chitosan, carboxymethyl cellulose, polyethylene glycol (PEG) and polyaniline derivatives) are highly biocompatible, can stabilize MNPs in aqueous solutions, increase the solubility of CTAs, and have been used for the delivery of doxorubicin, 5-fluorouracil and carmustine [Citation192,Citation193,Citation198–201]. Liposomal or lipid-based carriers can be designed to have phase transitions occur at specific temperatures (i.e. 43–46 °C) and have been used for heat-controlled release of doxorubicin or temozolomide (TMZ) [Citation190,Citation191,Citation194].
Magneto-PTT
MNPs that can additionally convert light energy into heat have been utilized for the combination of PTT and MHT. This approach involves administration of MNPs followed by application of AMF and delivery of near infrared (NIR) light. Groups have developed MNPs capable of PTT to overcome the diminished heating of MNPs caused by cellular internalization. MNPs designed for both MHT and PTT were shown to decrease cell viability both in vitro and in vivo to a greater extent than those used for MHT or PTT alone [Citation188]. In another study, novel FePt NPs were designed to perform magneto-PTT in combination with chemotherapy [Citation202]. Chemo-magneto-PTT had a more pronounced antitumor effect when compared to PTT monotherapy or PTT combined with chemotherapy.
Clinical studies
Two clinical trials within the past 10 years have evaluated the effects of MHT in combination with RT in humans. In the original study by Maier-Hauff et al. in 2011, 59 recurrent GBM patients underwent MHT in combination with fractionated RT [Citation203]. Aminosilane coated MIONPs were injected intratumorally at an iron concentration of 112 mg/mL prior to MHT treatments. The median volume of magnetic fluid injected was 4.5 mL (range 0.5–11.6 mL), translating to a median dose of 0.28 mL of magnetic fluid per cm3 of tumor volume. The thermotherapy generally consisted of six sessions with each session lasting 1 h. The median peak temperature measured within the tumor area during the sessions was 51.2 °C with a maximum of 82.0 °C. RT was performed immediately before or after thermotherapy and was fractionated (5 × 2 Gy per week) for a total dose of 30 Gy. As a first line therapy prior to MHT, 56/59 patients underwent surgical resection, 58/59 received RT and 51/59 chemotherapy. Patients were monitored at 3-month intervals with clinical follow up and CT scans (MRI was contraindicated due to the high concentration of MIONPs administered). The median overall survival (mOS) was 13.4 months for all patients suggesting that MHT in combination with fractionated stereotactic RT was clinically effective. Shortcomings reported in the study included the necessary removal of all metal implants within 40 cm of the treatment area (i.e. dental fillings) and the inability to use MRI for follow up due to MNP related artifacts.
In 2019, Grauer et al. reported on the effects of intracavitary MHT with RT on six recurrent GBM patients [Citation204]. Similar to the study by Maier-Hauff et al., aminosilane coated MIONPs were used at an iron concentration of 112 mg/mL. Two to three layers of the MIONPs were applied onto the cavity wall following 5-aminolevulinic acid (5-ALA) fluorescence-guided resection of the tumor. After the administration of the MIONPs, six 1 h sessions of MHT were performed. The first thermotherapy session was performed 3 days before the start of RT after which both treatments were performed on the same day. All patients included in the study had previously been irradiated with a median interval of 8.1 months and a standard dose of 60 Gy. Four out of six patients were re-irradiated in the study at a fractionated rate of 1.8 Gy 5×/week for a total dose of 39.6 Gy with two patients being excluded from re-irradiation due to maximum dose limitations. All patients had previously undergone surgical resection of their tumor. The mean follow-up time was 11.8 ± 9.3 months and the mOS and median PFS were 8.15 and 6.25 months, respectively. Patients treated at first recurrence had a mOS of 23.9 months whereas patients treated at second recurrence or later had a mOS of 7.1 months. One patient was still alive at the time of publication, 29 months after their last visit. However, 2–5 months post-MHT all patients experienced significant perifocal edema around the MNP deposits leading to clinical deterioration and prompting re-operation in four of the patients to remove NPs after which their condition improved. Further immunohistochemical analysis revealed significant infiltration of CD3+, CD8+ and CD68+ cells into the tumor sample after intracavitary thermotherapy. The reported survival benefits of MHT in combination with RT as well as the potential MHT-induced anti-tumor immune response are promising and warrant future investigation. The significant short-term cerebral edema related complications should be taken into consideration in the design of future trials, which should include closer monitoring and more comprehensive edema management.
Nanoparticle-mediated PTT
Nanoparticle-mediated PTT is a rapidly evolving platform for HT applications which relies on the thermal properties of nano-scale photothermal agents (PTAs) that can act as local heat sources, and the penetrating properties of NIR radiation, which can reach the tumor and excite the PTAs () [Citation205–207]. Nanoparticle-mediated PTT is a two-step therapy involving the local or systemic administration of PTAs followed by local application of light through NIR lasers. Light application to PTAs, results in localized surface plasmon resonance, through which PTAs absorb light at different wavelengths based on the oscillation of electrons on the surface and emit thermal energy increasing temperature in tumor tissue while preventing thermal damage to the surrounding normal brain [Citation208,Citation209]. PTT can be performed with pre-defined NIR light energy administration margins and temperature monitoring for further prevention of normal brain injury complications [Citation210,Citation211]. Different types of PTAs display different thermal properties according to their shapes, sizes and materials (). These characteristics reflect their heterogeneity on their electron number and configuration which in turn determine their light absorption and heat emission rates [Citation208]. PTAs with high NIR absorbance rate that have displayed antineoplastic effects in GBM experimental models include carbon nanotubes [Citation207,Citation212–214], carbon nanodots [Citation215], gold nanorods [Citation209,Citation216,Citation217], gold nanoshells [Citation217,Citation218], gold nanospheres [Citation219], gold nanostars [Citation52,Citation220], silk fibroin nanoparticles [Citation221], silicon based nanoparticles [Citation222] and iron-oxide carbon core-shell nanoparticles [Citation210].
Figure 3. Illustration of brain tumor nanoparticle-mediated photothermal therapy (PTT). (A) Schematic representation of local NIR light application to a brain tumor after photothermal agents (PTAs) administration (black spheres). (B) Schematic illustration demonstrating NIR light excitation of PTAs within the brain tumor. PTAs absorb NIR light and emit thermal energy acting as local heat sources.
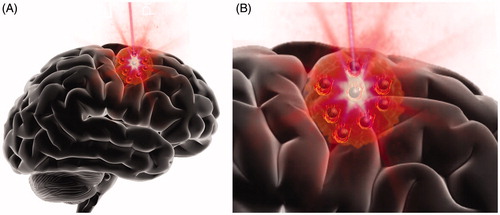
Table 2. Nanoparticle constructs utilized for photothermal therapy.
Targeted delivery of PTAs
In addition to their inherent heating properties, PTAs can be conjugated with antibodies, aptamers or other cell surface targeting compounds for their targeted delivery to the tumor [Citation223]. Conjugation of PTAs with PEG chains and folate, has been shown to enhance their uptake by GBM cells and increase the therapeutic effects of PTT in vitro [Citation224,Citation225]. PEG has been also conjugated with peptide 22 polypeptide targeting the low‐density lipoprotein receptor (LDLR). Peptide 22 polypeptide conjugation facilitated crossing of the BBB and delivery of the PTAs to the tumor in an orthotopic rodent glioma model [Citation226]. CD133 monoclonal antibodies have been conjugated with carbon and gold PTAs for the targeting of CD133+ glioma stem cells resulting in a profound decrease of tumor growth in both flank and orthotopic rodent glioma models [Citation214,Citation216]. Others have studied vascular endothelial growth factor (VEGF)-conjugated PTAs for targeting of the tumor vasculature in orthotopic GBM models [Citation227]. VEGF targeting doubled the concentration of PTAs bound to tumor vessels and induced significant vascular injury [Citation227]. Immune cells provide an additional method for the targeted delivery of PTAs. Monocytes and macrophages have been studied as potential vehicles, due to their capacity to phagocytize large doses of PTAs and their ability to cross the BBB and migrate toward tumor infiltrated tissues [Citation208,Citation228]. Nevertheless, this method of targeted delivery has been only studied in vitro [Citation217,Citation218].
Chemo-PTT
The coadministration of CTAs and PTAs can be achieved via their conjugation or loading in nanoplatforms to implement combined chemotherapy and PTT. Moreover, NIR radiation and heat can modulate the release of the CTAs from these compounds increasing the drug bioavailability and enhancing therapeutic effects [Citation219]. TMZ-loaded porous silicon nanoparticles (PSi NPs) have been studied in rodent GBM models [Citation222]. TMZ has been also conjugated with MIONPs and studied in vitro where apoptosis was induced with GBM cells after application of NIR light [Citation165]. Paclitaxel has been conjugated with gold nanospheres and studied in vitro and in vivo in GBM xenografts [Citation219]. Application of NIR light triggered its release and augmented antitumor effects [Citation219]. Curcin, a ribosome-inactivating protein has been conjugated with gold nanoparticles displaying high drug load capacity, pH-sensitive drug release and anti-proliferative effects in glioma cells lines in vitro [Citation225]. Docetaxel has been loaded on gold nanoparticles and showed therapeutic effects against GBM cells in vitro and in a flank rodent GBM model in vivo [Citation229]. Others have loaded campothecin (CPT), a topoisomerase inhibitor, on silica coated gold colloids which displayed cytotoxic effects in human glioma cells in vitro [Citation230]. Finally, doxycycline (DOX) loaded in carbon nanoparticles showed therapeutic effects in vivo, in an orthotopic rodent glioma model [Citation226].
Combined photodynamic/PTT
Photodynamic therapy (PDT) is a therapeutic approach involving the administration of a photosensitizer which upon the application of light, produces cytotoxic levels of ROS and triggers antitumor immune responses [Citation148,Citation231]. Most of the photosensitizers such as protoporphyrin IX (PpIX), 5,10,15,20-tetrakis(3-hydroxyphenyl) chlorin (mTHPC) and photofrin are excited with visible spectrum light which lacks the penetrating properties of NIR light [Citation148,Citation232,Citation233]. Therefore, the laser optical fiber needs to be inserted into the target lesion for potent excitation of the photosensitizer. Upconversion nanoparticles (UCNP) have been employed to overcome this limitation as they emit visible spectrum light under NIR excitation and convert part of the administered light energy into heat [Citation234]. Hence, UCNP act as both localized heaters and light sources enabling PTT and PDT effects [Citation234]. UCNP have been utilized for PTT and PDT of GBM after NIR irradiation exhibiting pronounced antitumor effects in vitro and in vivo [Citation206,Citation235]. Moreover, some researchers have utilized indocyanine (ICG) conjugated liposomal and silk fibroin nanoparticles that do not possess any light emitting properties coupled with NIR spectrum light and have reported substantial PDT/PTT effects in rodent GBM models without the use of UCNP or visible light application [Citation221,Citation231].
Understudied HT modalities
HT has been performed with a variety of modalities utilizing ultrasound (US), radiofrequency (RF), microwaves (MW) as well as with novel devices using more traditional forms of energy to induce HT (, ). Focused US has been utilized for HT for brain tumors in the clinical setting as a potential minimally invasive alternative for brain tumors; however, complications rate were high and reported data were not sufficient to characterize its safety and efficacy [Citation236–238]. Currently, US-based research paradigms are shifting toward bubble mediated US therapy or 5-ALA sonodynamic therapy (SDT) in which the effects of US ablation can be achieved with minimal temperature increases and thus prevent inadvertent thermal injury complications [Citation239–242]. HT can be also utilized through RF generators, a technique also known as loco-regional electro-hyperthermia (EHT). RF EHT has been demonstrated in experimental glioma models enabling tumor temperature increases from 42 to 45 °C [Citation14,Citation243]. RF EHT has been employed in a canine model that studied the heat mediated local release of vehicle conjugated chemotherapy. RF after administration of temperature sensitive liposomes conjugated with doxorubicin demonstrated heat mediated local release of the chemotherapeutic substrate [Citation244]. Furthermore, two studies have reported clinical EHT data. A Phase I clinical trial in patients with relapsed HGGs studied EHT with combined administration of nimustine. According to the reported data, the efficacy of EHT is not clear. Authors reported very high rates of edema related complications and thus suggested a phase II trial to further determine the effects of EHT [Citation245]. The second study reporting clinical data was a retrospective study comparing a cohort of 54 patients with recurrent GBM who received EHT and TMZ with a cohort of patients who only received TMZ. Authors reported that the combination of EHT and TMZ significantly improved mOS [Citation246]. HT has also been used with MW in combination with RT in an experimental rodent GBM model using patient-derived GBM cells showing a synergistic effect with an extended overall survival in animals [Citation32]. Recently, a pediatric brain MW applicator has been developed along with models that aim to optimize MW administration. However, MW HT has not been implemented to the clinical setting [Citation247].
Figure 4. Schematic illustration summarizing some of the less common HT modalities that have been employed for brain tumor treatment. HT for brain tumors has been performed with ultrasound (US), radiofrequency (RF) and microwaves (MW).
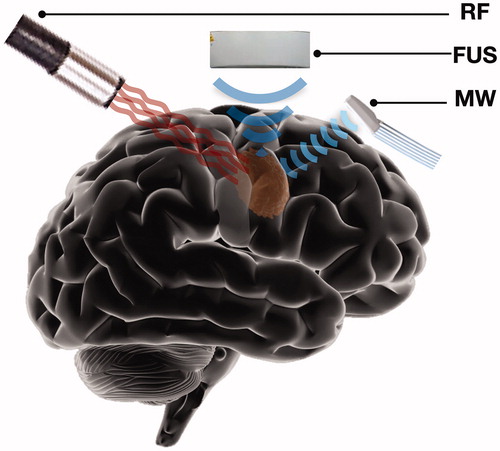
Table 3. Understudied HT modalities for the treatment of brain tumors.
Novel HT modalities in clinical settings
HT intracavitary devices have also been developed and reported in a phase I clinical trial [Citation248]. This devise is comprised of a water pump that circulates hot water to a cavitated gold coated sphere. The sphere can be inserted in the tumor cavity intraoperatively after the tumor has been resected for HT of the cavity. Authors utilized this novel HT devise in patients with brain metastases, and reported a significantly lower local recurrence rate among the HT treatment group compared to the group that received tumor resection without subsequent HT. An alternative novel technique, termed laser surface thermal therapy (LSTT) has been used in patients with HGGs [Citation249]. This technique involves thermal ablation of the post-resection tumor cavity through 808 and 1470 nm semiconductor lasers. The authors reported improved overall survival in HHG patients treated with LSTT in addition to microsurgical resection compared to patients who received only microsurgical resection. No LSTT-related adverse effects were reported.
Conclusion
HT is an important therapeutic modality for brain tumors that can be combined with other treatments. Multiple approaches exist for HT that include catheter implantation, MNPs and other agents. Currently, catheter-based LITT is the most common thermal ablation approach performed in brain tumor patients. LITT is a powerful, minimally invasive cytoreductive alternative that relies on MR imaging and real-time MR thermometry. The most rapidly evolving area in HT is the use of MNPs and AMF that can augment the effect of HT while sparing heat-related injury in the surrounding brain. More advanced MNPs with greater heating capacity and better tumor targeting schemes will provide the basis for future brain tumor treatments. Initial MHT clinical studies with early generation MNPs have been performed in Europe with encouraging results in combination with RT. The combination of thermal delivery with adjunct immunotherapeutic treatments is highly promising and warrants further investigation. Translational studies and carefully designed clinical trials are needed to continue the advancement of HT for treatment of brain tumors.
Disclosure statement
No potential conflict of interest was reported by the author(s).
Additional information
Funding
References
- Falk M, Issels R. Hyperthermia in oncology. Int J Hyperthermia. 2001;17(1):1–18.
- Hildebrandt B, Wust P, Ahlers O, et al. The cellular and molecular basis of hyperthermia. Crit Rev Oncol Hematol. 2002;43(1):33–56.
- Chu KF, Dupuy DE. Thermal ablation of tumours: biological mechanisms and advances in therapy. Nat Rev Cancer. 2014;14(3):199–208.
- Wust P, Hildebrandt B, Sreenivasa G, et al. Hyperthermia in combined treatment of cancer. Lancet Oncol. 2002;3(8):487–497.
- Bull JM, Lees DE, Schuette WH, et al. Immunological and physiological responses to whole-body hyperthermia. Natl Cancer Inst Monogr. 1982;61:177–181.
- Dewhirst MW, Lee C-T, Ashcraft KA. The future of biology in driving the field of hyperthermia. Int J Hyperthermia. 2016;32(1):4–13.
- Skitzki JJ, Repasky EA, Evans SS. Hyperthermia as an immunotherapy strategy for cancer. Curr Opin Invest Drugs (London, England: 2000). 2009;10(6):550.
- Issels R, Lindner LH. Regional hyperthermia for high-risk soft tissue sarcoma treatment: present status and next questions. Curr Opin Oncol. 2016;28(5):447–452.
- Nikfarjam M, Muralidharan V, Christophi C. Mechanisms of focal heat destruction of liver tumors. J Surg Res. 2005;127(2):208–223.
- Bing C, Nofiele J, Staruch R, et al. Localised hyperthermia in rodent models using an MRI-compatible high-intensity focused ultrasound system. Int J Hyperthermia. 2015;31(8):813–822.
- Fajardo LF, Egbert B, Marmor J, et al. Effects of hyperthermia in a malignant tumor. Cancer. 1980;45(3):613–623.
- Van der Zee J. Heating the patient: a promising approach? Ann Oncol. 2002;13(8):1173–1184.
- Kalamida D, Karagounis IV, Mitrakas A, et al. Fever-range hyperthermia vs. hypothermia effect on cancer cell viability, proliferation and HSP90 expression. PLoS One. 2015;10(1):e0116021.
- James JR, Gao Y, Soon VC, et al. Controlled radio-frequency hyperthermia using an MR scanner and simultaneous monitoring of temperature and therapy response by (1)H, (23)Na and (31)P magnetic resonance spectroscopy in subcutaneously implanted 9L-gliosarcoma. Int J Hyperthermia. 2010;26(1):79–90.
- Willis W, Jackman M, Bizeau M, et al. Hyperthermia impairs liver mitochondrial function in vitro. Am J Physiol Regul Integr Comp Physiol. 2000;278(5):R1240–R1246.
- Wheatley D, Kerr C, Gregory D. Heat-induced damage to HeLa-S3 cells: correlation of viability, permeability, osmosensitivity, phase-contrast light-, scanning electron- and transmission electron-microscopical findings . Int J Hyperthermia. 1989;5(2):145–162.
- Sakaguchi Y, Stephens LC, Makino M, et al. Apoptosis in tumors and normal tissues induced by whole body hyperthermia in rats. Cancer Res. 1995;55(22):5459–5464.
- Warters RL, Roti J. Hyperthermia and the cell nucleus. Radiat Res. 1982;92(3):458–462.
- Li GC, Mivechi NF, Weitzel G. Heat shock proteins, thermotolerance, and their relevance to clinical hyperthermia. Int J Hyperthermia. 1995;11(4):459–488.
- Ito A, Shinkai M, Honda H, et al. Heat shock protein 70 expression induces antitumor immunity during intracellular hyperthermia using magnetite nanoparticles. Cancer Immunol Immunother. 2003;52(2):80–88.
- Lianos GD, Alexiou GA, Mangano A, et al. The role of heat shock proteins in cancer. Cancer Lett. 2015;360(2):114–118.
- Neshasteh-Riz A, Eyvazzadeh N, Rostami A, et al. Cytogenetic damage from hyperthermia,6 MV X-rays, and topotecan in glioblastoma spheroids, simultaneously, and separately. J Cancer Res Ther. 2018;14(6):1273–1278.
- Kampinga HH. Cell biological effects of hyperthermia alone or combined with radiation or drugs: a short introduction to newcomers in the field. Int J Hyperthermia. 2006;22(3):191–196.
- Lee Titsworth W, Murad GJ, Hoh BL, et al. Fighting fire with fire: the revival of thermotherapy for gliomas. Anticancer Res. 2014;34(2):565–574.
- Kumar CS, Mohammad F. Magnetic nanomaterials for hyperthermia-based therapy and controlled drug delivery. Adv Drug Deliv Rev. 2011;63(9):789–808.
- Agarwal A, Mackey MA, El-Sayed MA, et al. Remote triggered release of doxorubicin in tumors by synergistic application of thermosensitive liposomes and gold nanorods. ACS Nano. 2011;5(6):4919–4926.
- Urakawa M, Yamaguchi K, Tsuchida E, et al. Blood–brain barrier disturbance following localized hyperthermia in rats. Int J Hyperthermia. 1995;11(5):709–718.
- Kong G, Braun RD, Dewhirst MW. Characterization of the effect of hyperthermia on nanoparticle extravasation from tumor vasculature. Cancer Res. 2001;61(7):3027–3032.
- Fike J, Gobbel G, Satoh T, et al. Normal brain response after interstitial microwave hyperthermia. Int J Hyperthermia. 1991;7(5):795–808.
- Gong W, Wang Z, Liu N, et al. Improving efficiency of adriamycin crossing blood brain barrier by combination of thermosensitive liposomes and hyperthermia. Biol Pharm Bull. 2011;34(7):1058–1064.
- Bidwell GL, 3rd, Perkins E, Hughes J, et al. Thermally targeted delivery of a c-Myc inhibitory polypeptide inhibits tumor progression and extends survival in a rat glioma model. PLoS One. 2013;8(1):e55104.
- Man J, Shoemake JD, Ma T, et al. Hyperthermia sensitizes glioma stem-like cells to radiation by inhibiting AKT signaling. Cancer Res. 2015;75(8):1760–1769.
- Watanabe M, Tanaka R, Hondo H, et al. Effects of antineoplastic agents and hyperthermia on cytotoxicity toward chronically hypoxic glioma cells. Int J Hyperthermia. 1992;8(1):131–138.
- Krawczyk PM, Eppink B, Essers J, et al. Mild hyperthermia inhibits homologous recombination, induces BRCA2 degradation, and sensitizes cancer cells to poly (ADP-ribose) polymerase-1 inhibition. Proc Natl Acad Sci USA. 2011;108(24):9851–9856.
- Hermisson M, Weller M. Hyperthermia enhanced chemosensitivity of human malignant glioma cells. Anticancer Res. 2000;20(3A):1819–1823.
- Raaphorst G, Chabot P, Doja S, et al. Effect of hyperthermia on cisplatin sensitivity in human glioma and ovarian carcinoma cell lines resistant and sensitive to cisplatin treatment. Int J Hyperthermia. 1996;12(2):211–222.
- Genet SC, Fujii Y, Maeda J, et al. Hyperthermia inhibits homologous recombination repair and sensitizes cells to ionizing radiation in a time- and temperature-dependent manner . J Cell Physiol. 2013;228(7):1473–1481.
- Atkinson RL, Zhang M, Diagaradjane P, et al. Thermal enhancement with optically activated gold nanoshells sensitizes breast cancer stem cells to radiation therapy. Sci Transl Med. 2010;2(55):55ra79.
- Horsman MR, Overgaard J. Hyperthermia: a potent enhancer of radiotherapy. Clin Oncol (R Coll Radiol). 2007;19(6):418–426.
- Neshasteh-Riz A, Rahdani R, Mostaar A. Evaluation of the combined effects of hyperthermia, cobalt-60 gamma rays and IUdR on cultured glioblastoma spheroid cells and dosimetry using TLD-100. Cell J. 2014;16(3):335–342.
- Neshastehriz A, Khosravi Z, Ghaznavi H, et al. Gold-coated iron oxide nanoparticles trigger apoptosis in the process of thermo-radiotherapy of U87-MG human glioma cells. Radiat Environ Biophys. 2018;57(4):405–418.
- Lee S, Son B, Park G, et al. Immunogenic effect of hyperthermia on enhancing radiotherapeutic efficacy. IJMS. 2018;19(9):2795.
- Haen SP, Pereira PL, Salih HR, et al. More than just tumor destruction: immunomodulation by thermal ablation of cancer. Clin Dev Immunol. 2011;2011:160250.
- Ando K, Suzuki Y, Kaminuma T, et al. Tumor-specific CD8-positive T cell-mediated antitumor immunity is implicated in the antitumor effect of local hyperthermia. Int J Hyperthermia. 2018;35(1):226–231.
- Dayanc BE, Beachy SH, Ostberg JR, et al. Dissecting the role of hyperthermia in natural killer cell mediated anti-tumor responses. Int J Hyperthermia. 2008;24(1):41–56.
- den Brok MH, Sutmuller RP, van der Voort R, et al. In situ tumor ablation creates an antigen source for the generation of antitumor immunity. Cancer Res. 2004;64(11):4024–4029.
- Johnson EE, Yamane BH, Buhtoiarov IN, et al. Radiofrequency ablation combined with KS-IL2 immunocytokine (EMD 273066) results in an enhanced antitumor effect against murine colon adenocarcinoma. Clin Cancer Res. 2009;15(15):4875–4884.
- Hamamoto S, Okuma T, Yamamoto A, et al. Radiofrequency ablation and immunostimulant OK-432: combination therapy enhances systemic antitumor immunity for treatment of VX2 lung tumors in rabbits. Radiology. 2013;267(2):405–413.
- Oei AL, Korangath P, Mulka K, et al. Enhancing the abscopal effect of radiation and immune checkpoint inhibitor therapies with magnetic nanoparticle hyperthermia in a model of metastatic breast cancer. Int J Hyperthermia. 2019;36(sup1):47–63.
- Hormigo A, Mandeli J, Hadjipanayis C, et al. Phase I study of PD-L1 inhibition with avelumab and laser interstitial thermal therapy in patients with recurrent glioblastoma. J Clin Oncol. 2019;37(15_suppl):TPS2074.
- Campian J, Ghiaseddin A, Rahman M, et al. ATIM-45. Long term follow-up of a phase I/II study testing the toxicities and efficacy of pembrolizumab in combination with mri-guided laser interstitial thermal therapy (LITT) in recurrent malignant gliomas. Neuro-Oncology. 2019;21(Supplement_6):vi11.
- Liu Y, Chongsathidkiet P, Crawford B, et al. Plasmonic gold nanostar-mediated photothermal immunotherapy for brain tumor ablation and immunologic memory. Immunotherapy. 2019;11(15):1293–1302.
- Kim St AH, Rao G, Prabhu S, et al. Laser ablation of abnormal neurological tissue using robotic neuroblate system (LAANTERN): 12-month outcomes and quality of life after brain tumor ablation. Neurosurgery. 2020. DOI: 10.1093/neuros/nyaa071
- Shah AH, Semonche A, Eichberg DG, et al. The role of laser interstitial thermal therapy in surgical neuro-oncology: series of 100 consecutive patients. Neurosurgery. 2019. DOI: 10.1093/neuros/nyz424
- Stafford RJ, Fuentes D, Elliott AA, et al. Laser-induced thermal therapy for tumor ablation. Crit Rev Biomed Eng. 2010;38(1):79–100.
- Bown S. Phototherapy in tumors. World J Surg. 1983;7(6):700–709.
- Sugiyama K, Sakai T, Fujishima I, et al. Stereotactic interstitial laser-hyperthermia using Nd-YAG laser. Stereotact Funct Neurosurg. 1990;54(1–8):501–505.
- Medvid R, Ruiz A, Komotar RJ, et al. Current applications of MRI-guided laser interstitial thermal therapy in the treatment of brain neoplasms and epilepsy: a radiologic and neurosurgical overview. AJNR Am J Neuroradiol. 2015;36(11):1998–2006.
- Belykh E, Yagmurlu K, Martirosyan NL, et al. Laser application in neurosurgery. Surg Neurol Int. 2017;8:274.
- Karampelas I, Sloan AE. Laser-induced interstitial thermotherapy of gliomas. Prog Neurol Surg. 2018;32:14–26.
- Sherman JH, Hoes K, Marcus J, et al. Neurosurgery for brain tumors: update on recent technical advances. Curr Neurol Neurosci Rep. 2011;11(3):313–319.
- Franzini A, Moosa S, Servello D, et al. Ablative brain surgery: an overview. Int J Hyperthermia. 2019;36(2):64–80.
- Rennert RC, Khan U, Tatter SB, et al. Patterns of clinical use of stereotactic laser ablation: analysis of a multicenter prospective registry. World Neurosurg. 2018;116:e566–e570.
- Rennert RC, Carroll KT, Ali MA, et al. Safety of stereotactic laser ablations performed as treatment for glioblastomas in a conventional magnetic resonance imaging suite. Neurosurg Focus. 2016;41(4):E7.
- Miller BA, Salehi A, Limbrick DD, Jr, et al. Applications of a robotic stereotactic arm for pediatric epilepsy and neurooncology surgery. J Neurosurg Pediatr. 2017;20(4):364–370.
- Patel NV, Jethwa PR, Shetty A, et al. Does the real-time thermal damage estimate allow for estimation of tumor control after MRI-guided laser-induced thermal therapy? Initial experience with recurrent intracranial ependymomas. J Neurosurg Pediatr. 2015;15(4):363–371.
- Diaz R, Ivan ME, Hanft S, et al. Laser interstitial thermal therapy: lighting the way to a new treatment option in neurosurgery. Neurosurgery. 2016;79(suppl_1):S3–S7.
- Quesson B, de Zwart JA, Moonen CT. Magnetic resonance temperature imaging for guidance of thermotherapy. J Magn Reson Imaging. 2000;12(4):525–533.
- Leuthardt EC, Voigt J, Kim AH, et al. A single-center cost analysis of treating primary and metastatic brain cancers with either brain laser interstitial thermal therapy (LITT) or craniotomy. Pharmacoecon Open. 2017;1(1):53–63.
- Ashraf O, Patel NV, Hanft S, et al. Laser-induced thermal therapy in neuro-oncology: a review. World Neurosurg. 2018;112:166–177.
- Laurent D, Oliveria SF, Shang M, et al. Techniques to ensure accurate targeting for delivery of awake laser interstitial thermotherapy. Oper Neurosurg (Hagerstown). 2018;15(4):454–460.
- Shah AH, Richardson AM, Burks JD, et al. Contemporaneous biopsy and laser interstitial thermal therapy for two treatment-refractory brain metastases. Neurosurg Focus. 2018;44(VideoSuppl2):V5.
- Patel NV, Mian M, Stafford RJ, et al. Laser interstitial thermal therapy technology, physics of magnetic resonance imaging thermometry, and technical considerations for proper catheter placement during magnetic resonance imaging-guided laser interstitial thermal therapy. Neurosurgery. 2016;79(Suppl 1):S8–S16.
- Missios S, Bekelis K, Barnett GH. Renaissance of laser interstitial thermal ablation. Neurosurg Focus. 2015;38(3):E13.
- Mohammadi AM, Schroeder JL. Laser interstitial thermal therapy in treatment of brain tumors – the NeuroBlate System. Expert Rev Med Devices. 2014;11(2):109–119.
- Torres-Reveron J, Tomasiewicz HC, Shetty A, et al. Stereotactic laser induced thermotherapy (LITT): a novel treatment for brain lesions regrowing after radiosurgery. J Neurooncol. 2013;113(3):495–503.
- Jethwa PR, Barrese JC, Gowda A, et al. Magnetic resonance thermometry-guided laser-induced thermal therapy for intracranial neoplasms: initial experience. Neurosurgery. 2012;71(1 Suppl Operative):133–144.
- Sloan AE, Ahluwalia MS, Valerio-Pascua J, et al. Results of the NeuroBlate system first-in-humans Phase I clinical trial for recurrent glioblastoma: clinical article. J Neurosurg. 2013;118(6):1202–1219.
- Elder JB, Huntoon K, Otero J, et al. Histologic findings associated with laser interstitial thermotherapy for glioblastoma multiforme. Diagn Pathol. 2019;14(1):19.
- Pruitt R, Gamble A, Black K, et al. Complication avoidance in laser interstitial thermal therapy: lessons learned. JNS. 2017;126(4):1238–1245.
- Leuthardt EC, Duan C, Kim MJ, et al. Hyperthermic laser ablation of recurrent glioblastoma leads to temporary disruption of the peritumoral blood brain barrier. PLoS One. 2016;11(2):e0148613.
- Tran D, Leuthardt E, Shimony J, et al. ACTR-82. Laser interstitial thermal therapy (LITT) of recurrent glioblastoma (GBM) induces temporary disruption of the peritumoral blood brain barrier (BBB) and may improve efficacy of chemotherapy with poor CNS penetration. Neuro-oncology. 2017;19(suppl_6):vi18.
- Bastos DCA, Rao G, Oliva ICG, et al. Predictors of local control of brain metastasis treated with laser interstitial thermal therapy. Neurosurgery. 2019. DOI: 10.1093/neuros/nyz357
- Patel P, Patel NV, Danish SF. Intracranial MR-guided laser-induced thermal therapy: single-center experience with the Visualase thermal therapy system. J Neurosurg. 2016;125(4):853–860.
- Carpentier A, McNichols RJ, Stafford RJ, et al. Laser thermal therapy: real-time MRI-guided and computer-controlled procedures for metastatic brain tumors. Lasers Surg Med. 2011;43(10):943–950.
- Smith CJ, Myers CS, Chapple KM, et al. Long-term follow-up of 25 cases of biopsy-proven radiation necrosis or post-radiation treatment effect treated with magnetic resonance-guided laser interstitial thermal therapy. Neurosurgery. 2016;79 Suppl 1(Suppl 1):S59–S72.
- Fabiano AJ, Qiu J. Delayed failure of laser-induced interstitial thermotherapy for postradiosurgery brain metastases. World Neurosurg. 2014;82(3–4):e559–e563.
- Rao MS, Hargreaves EL, Khan AJ, et al. Magnetic resonance-guided laser ablation improves local control for postradiosurgery recurrence and/or radiation necrosis. Neurosurgery. 2014;74(6):658–667. discussion 667.
- Eichberg DG, VanDenBerg R, Komotar RJ, et al. Quantitative volumetric analysis following magnetic resonance-guided laser interstitial thermal ablation of cerebellar metastases. World Neurosurg. 2018;110:e755–e765.
- Beechar VB, Prabhu SS, Bastos D, et al. Volumetric response of progressing post-SRS lesions treated with laser interstitial thermal therapy. J Neurooncol. 2018;137(1):57–65.
- Kamath AA, Friedman DD, Hacker CD, et al. MRI-guided interstitial laser ablation for intracranial lesions: a large single-institution experience of 133 cases. Stereotact Funct Neurosurg. 2017;95(6):417–428.
- Torcuator RG, Hulou MM, Chavakula V, et al. Intraoperative real-time MRI-guided stereotactic biopsy followed by laser thermal ablation for progressive brain metastases after radiosurgery. J Clin Neurosci. 2016;24:68–73.
- Rammo R, Asmaro K, Schultz L, et al. The safety of magnetic resonance imaging-guided laser interstitial thermal therapy for cerebral radiation necrosis. J Neurooncol. 2018;138(3):609–617.
- Sharma M, Krivosheya D, Borghei-Razavi H, et al. Laser interstitial thermal therapy for an eloquent region supratentorial brain lesion. Neurosurg Focus. 2018;44(VideoSuppl2):V4.
- Maraka S, Asmaro K, Walbert T, et al. Cerebral edema induced by laser interstitial thermal therapy and radiotherapy in close succession in patients with brain tumor. Lasers Surg Med. 2018;50(9):917–923.
- Hernandez RN, Carminucci A, Patel P, et al. Magnetic resonance-guided laser-induced thermal therapy for the treatment of progressive enhancing inflammatory reactions following stereotactic radiosurgery, or PEIRs, for metastatic brain disease. Neurosurgery. 2019;85(1):84–90.
- Borghei-Razavi H, Koech H, Sharma M, et al. Laser interstitial thermal therapy for posterior fossa lesions: an initial experience. World Neurosurg. 2018;117:e146–e153.
- Sharma M, Habboub G, Behbahani M, et al. Thermal injury to corticospinal tracts and postoperative motor deficits after laser interstitial thermal therapy. Neurosurg Focus. 2016;41(4):E6.
- Wright J, Chugh J, Wright CH, et al. Laser interstitial thermal therapy followed by minimal-access transsulcal resection for the treatment of large and difficult to access brain tumors. Neurosurg Focus. 2016;41(4):E14.
- Salehi A, Kamath AA, Leuthardt EC, et al. Management of intracranial metastatic disease with laser interstitial thermal therapy. Front Oncol. 2018;8:499.
- Munier SM, Hargreaves EL, Patel NV, et al. Ablation dynamics of subsequent thermal doses delivered to previously heat-damaged tissue during magnetic resonance-guided laser-induced thermal therapy. J Neurosurg. 2018;131:1683–1994.
- Hong CS, Deng D, Vera A, et al. Laser-interstitial thermal therapy compared to craniotomy for treatment of radiation necrosis or recurrent tumor in brain metastases failing radiosurgery. J Neurooncol. 2019;142(2):309–317.
- Patel NV, Jethwa PR, Barrese JC, et al. Volumetric trends associated with MRI-guided laser-induced thermal therapy (LITT) for intracranial tumors. Lasers Surg Med. 2013;45(6):362–369.
- Hawasli AH, Bagade S, Shimony JS, et al. Magnetic resonance imaging-guided focused laser interstitial thermal therapy for intracranial lesions: single-institution series. Neurosurgery. 2013;73(6):1007–1017.
- Fabiano AJ, Alberico RA. Laser-interstitial thermal therapy for refractory cerebral edema from post-radiosurgery metastasis. World Neurosurg. 2014;81(3–4):652.e1–652.e4.
- Swartz LK, Holste KG, Kim MM, et al. Outcomes in patients treated with laser interstitial thermal therapy for primary brain cancer and brain metastases. Oncologist. 2019;24(12):e1467–e1470.
- Traylor JI, Patel R, Habib A, et al. Laser interstitial thermal therapy to the posterior fossa: challenges and nuances. World Neurosurg. 2019;132:e124–e132.
- Ahluwalia M, Barnett GH, Deng D, et al. Laser ablation after stereotactic radiosurgery: a multicenter prospective study in patients with metastatic brain tumors and radiation necrosis. J Neurosurg. 2018;130(3):804–811.
- Qian JM, Yu JB, Mahajan A, et al. Frequent use of local therapy underscores need for multidisciplinary care in the management of patients with melanoma brain metastases treated with PD-1 inhibitors. Int J Radiat Oncol Biol Phys. 2019;105(5):1113–1118.
- Iyer A, Halpern CH, Grant GA, et al. Magnetic resonance-guided laser-induced thermal therapy for recurrent brain metastases in the motor strip after stereotactic radiosurgery. Cureus. 2016;8(12):e919.
- Hawasli AH, Ray WZ, Murphy RK, et al. Magnetic resonance imaging-guided focused laser interstitial thermal therapy for subinsular metastatic adenocarcinoma: technical case report. Neurosurgery. 2012;70(2 Suppl Operative):332–337. discussion 338.
- Chaunzwa TL, Deng D, Leuthardt EC, et al. Laser thermal ablation for metastases failing radiosurgery: a multicentered retrospective study. Neurosurgery. 2018;82(1):56–63.
- Alattar AA, Bartek J, Jr, Chiang VL, et al. Stereotactic laser ablation as treatment for brain metastases recurring after stereotactic radiosurgery: a systematic literature review. World Neurosurg. 2019;128:134–142.
- Lee I, Kalkanis S, Hadjipanayis CG. Stereotactic laser interstitial thermal therapy for recurrent high-grade gliomas. Neurosurgery. 2016;79(Suppl 1):S24–S34.
- Sanai N, Berger MS. Surgical oncology for gliomas: the state of the art. Nat Rev Clin Oncol. 2018;15(2):112–125.
- Hawasli AH, Kim AH, Dunn GP, et al. Stereotactic laser ablation of high-grade gliomas. Neurosurg Focus. 2014;37(6):E1.
- Rahmathulla G, Recinos PF, Kamian K, et al. MRI-guided laser interstitial thermal therapy in neuro-oncology: a review of its current clinical applications. Oncology. 2014;87(2):67–82.
- Norred SE, Johnson JA. Magnetic resonance-guided laser induced thermal therapy for glioblastoma multiforme: a review. Biomed Res Int. 2014;2014:761312.
- Hafez DM, Liekweg C, Leuthardt EC. Staged laser interstitial thermal therapy (LITT) treatments to left insular low-grade glioma. Neurosurgery. 2019;86(3):E337–E342.
- Bartek J, Jr, Alattar A, Jensdottir M, et al. Biopsy and ablation of H3K27 glioma using skull-mounted smartframe device: technical case report. World Neurosurg. 2019;127:436–441.
- Zervos TM, Robin AM, Lee I. Delirium and topographical disorientation associated with glioblastoma multiforme tumour progression into the isthmus of the cingulate gyrus. BMJ Case Rep. 2018;2018:bcr2018225473.
- Carpentier A, Chauvet D, Reina V, et al. MR-guided laser-induced thermal therapy (LITT) for recurrent glioblastomas. Lasers Surg Med. 2012;44(5):361–368.
- Pisipati S, Smith KA, Shah K, et al. Intracerebral laser interstitial thermal therapy followed by tumor resection to minimize cerebral edema. Neurosurg Focus. 2016;41(4):E13.
- Shah AH, Burks JD, Buttrick SS, et al. Laser interstitial thermal therapy as a primary treatment for deep inaccessible gliomas. Neurosurgery. 2019;84(3):768–777.
- Beaumont TL, Mohammadi AM, Kim AH, et al. Magnetic resonance imaging-guided laser interstitial thermal therapy for glioblastoma of the corpus callosum. Neurosurgery. 2018;83(3):556–565.
- Ali SC, Basil GW, Diaz RJ, et al. The safety of bevacizumab administered shortly after laser interstitial thermal therapy in glioblastoma: a case series. World Neurosurg. 2018;117:e588–e594.
- Kamath AA, Friedman DD, Akbari SHA, et al. Glioblastoma treated with magnetic resonance imaging-guided laser interstitial thermal therapy: safety, efficacy, and outcomes. Neurosurgery. 2019;84(4):836–843.
- Galldiks N, von Tempelhoff W, Kahraman D, et al. 11C-Methionine positron emission tomographic imaging of biologic activity of a recurrent glioblastoma treated with stereotaxy-guided laser-induced interstitial thermotherapy. Mol Imaging. 2012;11(4):265–271.
- Mohammadi AM, Hawasli AH, Rodriguez A, et al. The role of laser interstitial thermal therapy in enhancing progression-free survival of difficult-to-access high-grade gliomas: a multicenter study. Cancer Med. 2014;3(4):971–979.
- Thomas JG, Rao G, Kew Y, et al. Laser interstitial thermal therapy for newly diagnosed and recurrent glioblastoma. Neurosurg Focus. 2016;41(4):E12.
- Ivan ME, Diaz RJ, Berger MH, et al. Magnetic resonance-guided laser ablation for the treatment of recurrent dural-based lesions: a series of five cases. World Neurosurg. 2017;98:162–170.
- Barnett GH, Voigt JD, Alhuwalia MS. A systematic review and meta-analysis of studies examining the use of brain laser interstitial thermal therapy versus craniotomy for the treatment of high-grade tumors in or near areas of eloquence: an examination of the extent of resection and major complication rates associated with each type of surgery. Stereotact Funct Neurosurg. 2016;94(3):164–173.
- Ivan ME, Mohammadi AM, De Deugd N, et al. Laser ablation of newly diagnosed malignant gliomas: a meta-analysis. Neurosurgery. 2016;79 (Suppl 1):S17–S23.
- Ruiz A, Diaz RJ, Buttrick S, et al. Preliminary experience on laser interstitial thermal ablation therapy in the treatment of extra-axial masses: indications, imaging characterization and outcomes. Cureus. 2018;10(6):e2894.
- Rammo R, Scarpace L, Nagaraja T, et al. MR-guided laser interstitial thermal therapy in the treatment of recurrent intracranial meningiomas. Lasers Surg Med. 2019;51(3):245–250.
- Tovar-Spinoza Z, Choi H. Magnetic resonance-guided laser interstitial thermal therapy: report of a series of pediatric brain tumors. J Neurosurg Pediatr. 2016;17(6):723–733.
- Xu DS, Rosenfeld A, Ponce FA, et al. Cerebral peduncle tumor ablated by novel 3-mm laser tip. Stereotact Funct Neurosurg. 2015;93(1):38–41.
- Tovar-Spinoza Z, Choi H. MRI-guided laser interstitial thermal therapy for the treatment of low-grade gliomas in children: a case-series review, description of the current technologies and perspectives. Childs Nerv Syst. 2016;32(10):1947–1956.
- Riordan M, Tovar-Spinoza Z. Laser induced thermal therapy (LITT) for pediatric brain tumors: case-based review. Transl Pediatr. 2014;3(3):229–235.
- Buckley RT, Wang AC, Miller JW, et al. Stereotactic laser ablation for hypothalamic and deep intraventricular lesions. Neurosurg Focus. 2016;41(4):E10.
- Dadey DY, Kamath AA, Leuthardt EC, et al. Laser interstitial thermal therapy for subependymal giant cell astrocytoma: technical case report. Neurosurg Focus. 2016;41(4):E9.
- Karsy M, Patel DM, Bollo RJ. Trapped ventricle after laser ablation of a subependymal giant cell astrocytoma complicated by intraventricular gadolinium extravasation: case report. J Neurosurg Pediatr. 2018;21(5):523–527.
- Yang HW, Hua MY, Hwang TL, et al. Non-invasive synergistic treatment of brain tumors by targeted chemotherapeutic delivery and amplified focused ultrasound-hyperthermia using magnetic nanographene oxide. Adv Mater Weinheim. 2013;25(26):3605–3611.
- Jethwa PR, Lee JH, Assina R, et al. Treatment of a supratentorial primitive neuroectodermal tumor using magnetic resonance-guided laser-induced thermal therapy. J Neurosurg Pediatr. 2011;8(5):468–475.
- Kuo CH, Feroze AH, Poliachik SL, et al. Laser ablation therapy for pediatric patients with intracranial lesions in eloquent areas. World Neurosurg. 2019;121:e191–e199.
- Kozissnik B, Bohorquez AC, Dobson J, et al. Magnetic fluid hyperthermia: advances, challenges, and opportunity. Int J Hyperthermia. 2013;29(8):706–714.
- Dennis CL, Ivkov R. Physics of heat generation using magnetic nanoparticles for hyperthermia. Int J Hyperthermia. 2013;29(8):715–729.
- Mahmoudi K, Bouras A, Bozec D, et al. Magnetic hyperthermia therapy for the treatment of glioblastoma: a review of the therapy’s history, efficacy and application in humans. Int J Hyperthermia. 2018;34(8):1316–1328.
- Hadjipanayis CG, Machaidze R, Kaluzova M, et al. EGFRvIII antibody-conjugated iron oxide nanoparticles for magnetic resonance imaging-guided convection-enhanced delivery and targeted therapy of glioblastoma. Cancer Res. 2010;70(15):6303–6312.
- Grüttner C, Müller K, Teller J, et al. Synthesis and antibody conjugation of magnetic nanoparticles with improved specific power absorption rates for alternating magnetic field cancer therapy. J Magn Magn Mater. 2007;311(1):181–186.
- Yoo M-K, Park I-K, Lim H-T, et al. Folate–PEG–superparamagnetic iron oxide nanoparticles for lung cancer imaging. Acta Biomater. 2012;8(8):3005–3013.
- Freeman AC, Platt SR, Holmes S, et al. Convection-enhanced delivery of cetuximab conjugated iron-oxide nanoparticles for treatment of spontaneous canine intracranial gliomas. J Neurooncol. 2018;137(3):653–663.
- Platt S, Nduom E, Kent M, et al. Canine model of convection-enhanced delivery of cetuximab-conjugated iron-oxide nanoparticles monitored with magnetic resonance imaging. Clin Neurosurg. 2012;59:107–113.
- Kaluzova M, Bouras A, Machaidze R, et al. Targeted therapy of glioblastoma stem-like cells and tumor non-stem cells using cetuximab-conjugated iron-oxide nanoparticles. Oncotarget. 2015;6(11):8788–8806.
- Lidar Z, Mardor Y, Jonas T, et al. Convection-enhanced delivery of paclitaxel for the treatment of recurrent malignant glioma: a phase I/II clinical study. J Neurosurg. 2004;100(3):472–479.
- Liu L, Ni F, Zhang J, et al. Thermal analysis in the rat glioma model during directly multipoint injection hyperthermia incorporating magnetic nanoparticles. J Nanosci Nanotechnol. 2011;11(12):10333–10338.
- Tong S, Quinto CA, Zhang L, et al. Size-dependent heating of magnetic iron oxide nanoparticles. ACS Nano. 2017;11(7):6808–6816.
- Lanier OL, Korotych OI, Monsalve AG, et al. Evaluation of magnetic nanoparticles for magnetic fluid hyperthermia. Int J Hyperthermia. 2019;36(1):686–700.
- Wegscheid ML, Morshed RA, Cheng Y, et al. The art of attraction: applications of multifunctional magnetic nanomaterials for malignant glioma. Expert Opin Drug Deliv. 2014;11(6):957–975.
- Markides H, Rotherham M, Haj A. Biocompatibility and toxicity of magnetic nanoparticles in regenerative medicine. J Nanomater. 2012;2012. Article ID 614094.
- Dennis CL, Krycka KL, Borchers JA, et al. Internal magnetic structure of nanoparticles dominates time‐dependent relaxation processes in a magnetic field. Adv Funct Mater. 2015;25(27):4300–4311.
- Yi GQ, Gu B, Chen LK. The safety and efficacy of magnetic nano-iron hyperthermia therapy on rat brain glioma. Tumour Biol. 2014;35(3):2445–2449.
- Périgo EA, Hemery G, Sandre O, et al. Fundamentals and advances in magnetic hyperthermia. Appl Phys Rev. 2015;12(4):041302.
- Jingwen Y, Chao-Hsiung H, Zhao L, et al. Magnetic resonance nano-theranostics for glioblastoma multiforme. Curr Pharm Des. 2015;21(36):5256–5266.
- Kwon YM, Je J-Y, Cha SH, et al. Synergistic combination of chemo-phototherapy based on temozolomide/ICG-loaded iron oxide nanoparticles for brain cancer treatment. Oncol Rep. 2019;42(5):1709–1724.
- Hadjipanayis CG, Bonder MJ, Balakrishnan S, et al. Metallic iron nanoparticles for MRI contrast enhancement and local hyperthermia. Small. 2008;4(11):1925–1929.
- Wankhede M, Bouras A, Kaluzova M, et al. Magnetic nanoparticles: an emerging technology for malignant brain tumor imaging and therapy. Expert Rev Clin Pharmacol. 2012;5(2):173–186.
- Shirvalilou S, Khoei S, Khoee S, et al. Development of a magnetic nano-graphene oxide carrier for improved glioma-targeted drug delivery and imaging: In vitro and in vivo evaluations. Chem Biol Interact. 2018;295:97–108.
- Liu XL, Ng CT, Chandrasekharan P, et al. Synthesis of ferromagnetic Fe0.6 Mn0.4 O nanoflowers as a new class of magnetic theranostic platform for in vivo T1–T2 dual-mode magnetic resonance imaging and magnetic hyperthermia therapy. Adv Healthc Mater. 2016;5(16):2092–2104.
- Jordan A. Hyperthermia classic commentary: ‘Inductive heating of ferrimagnetic particles and magnetic fluids: physical evaluation of their potential for hyperthermia’ by Andreas Jordan et al., International Journal of Hyperthermia, 1993;9:51–68. Int J Hyperthermia. 2009;25(7):512–516.
- Rego G, Mamani JB, Souza TKF, et al. Therapeutic evaluation of magnetic hyperthermia using Fe3O4-aminosilane-coated iron oxide nanoparticles in glioblastoma animal model. Einstein (Sao Paulo, Brazil). 2019;17(4):eAO4786.
- Gupta R, Sharma D. Biofunctionalization of magnetite nanoparticles with stevioside: effect on the size and thermal behaviour for use in hyperthermia applications. Int J Hyperthermia. 2019;36(1):302–312.
- Nguyen VTA, De Pauw-Gillet M-C, Gauthier M, et al. Magnetic polyion complex micelles for cell toxicity induced by radiofrequency magnetic field hyperthermia. Nanomaterials (Basel, Switzerland). 2018;8(12):1014.
- Le Fèvre R, Durand-Dubief M, Chebbi I, et al. Enhanced antitumor efficacy of biocompatible magnetosomes for the magnetic hyperthermia treatment of glioblastoma. Theranostics. 2017;7(18):4618–4631.
- Alphandéry E, Idbaih A, Adam C, et al. Development of non-pyrogenic magnetosome minerals coated with poly-l-lysine leading to full disappearance of intracranial U87-Luc glioblastoma in 100% of treated mice using magnetic hyperthermia. Biomaterials. 2017;141:210–222.
- Alphandéry E, Idbaih A, Adam C, et al. Chains of magnetosomes with controlled endotoxin release and partial tumor occupation induce full destruction of intracranial U87-Luc glioma in mice under the application of an alternating magnetic field. J Control Release. 2017;262:259–272.
- Hamdous Y, Chebbi I, Mandawala C, et al. Biocompatible coated magnetosome minerals with various organization and cellular interaction properties induce cytotoxicity towards RG-2 and GL-261 glioma cells in the presence of an alternating magnetic field. J Nanobiotechnol. 2017;15(1):74–74.
- Jiang H, Wang C, Guo Z, et al. Silver nanocrystals mediated combination therapy of radiation with magnetic hyperthermia on glioma cells. J Nanosci Nanotechnol. 2012;12(11):8276–8281.
- Liu L, Ni F, Zhang J, et al. Silver nanocrystals sensitize magnetic-nanoparticle-mediated thermo-induced killing of cancer cells. Acta Biochim Biophys Sin (Shanghai). 2011;43(4):316–323.
- Di Corato R, Espinosa A, Lartigue L, et al. Magnetic hyperthermia efficiency in the cellular environment for different nanoparticle designs. Biomaterials. 2014;35(24):6400–6411.
- Guardia P, Di Corato R, Lartigue L, et al. Water-soluble iron oxide nanocubes with high values of specific absorption rate for cancer cell hyperthermia treatment. ACS Nano. 2012;6(4):3080–3091.
- Espinosa A, Kolosnjaj-Tabi J, Abou-Hassan A, et al. Magnetic (hyper)thermia or photothermia? Progressive comparison of iron oxide and gold nanoparticles heating in water, in cells, and in vivo. Adv Funct Mater. 2018;28(37):1803660.
- Del Sol-Fernández S, Portilla-Tundidor Y, Gutiérrez L, et al. Flower-like Mn-doped magnetic nanoparticles functionalized with αvβ3-integrin-ligand to efficiently induce intracellular heat after alternating magnetic field exposition, triggering glioma cell death. ACS Appl Mater Interfaces. 2019;11(30):26648–26663.
- Adamiano A, Wu VM, Carella F, et al. Magnetic calcium phosphates nanocomposites for the intracellular hyperthermia of cancers of bone and brain. Nanomedicine (Lond). 2019;14(10):1267–1289.
- Shevtsov MA, Yakovleva LY, Nikolaev BP, et al. Tumor targeting using magnetic nanoparticle Hsp70 conjugate in a model of C6 glioma. Neuro-oncology. 2014;16(1):38–49.
- Pala K, Serwotka A, Jeleń F, et al. Tumor-specific hyperthermia with aptamer-tagged superparamagnetic nanoparticles. Int J Nanomedicine. 2014;9:67–76.
- Zhou P, Zhao H, Wang Q, et al. Photoacoustic-enabled self-guidance in magnetic-hyperthermia Fe@Fe3O4 nanoparticles for theranostics in vivo. Adv Healthcare Mater. 2018;7(9):1701201.
- Lu Q, Dai X, Zhang P, et al. Fe(3)O(4)@Au composite magnetic nanoparticles modified with cetuximab for targeted magneto-photothermal therapy of glioma cells. Int J Nanomedicine. 2018;13:2491–2505.
- Jia G, Han Y, An Y, et al. NRP-1 targeted and cargo-loaded exosomes facilitate simultaneous imaging and therapy of glioma in vitro and in vivo. Biomaterials. 2018;178:302–316.
- Shi D, Mi G, Shen Y, et al. Glioma-targeted dual functionalized thermosensitive Ferri-liposomes for drug delivery through an in vitro blood–brain barrier. Nanoscale. 2019;11(32):15057–15071.
- Babincová N, Sourivong P, Babinec P, et al. Applications of magnetoliposomes with encapsulated doxorubicin for integrated chemotherapy and hyperthermia of rat C6 glioma. Z Naturforsch. 2018;73(7–8):265–271.
- Zamora-Mora V, Fernández-Gutiérrez M, González-Gómez Á, et al. Chitosan nanoparticles for combined drug delivery and magnetic hyperthermia: from preparation to in vitro studies. Carbohydr Polym. 2017;157:361–370.
- Carvalho SM, Leonel AG, Mansur AAP, et al. Bifunctional magnetopolymersomes of iron oxide nanoparticles and carboxymethylcellulose conjugated with doxorubicin for hyperthermo-chemotherapy of brain cancer cells. Biomater Sci. 2019;7(5):2102–2122.
- Tapeinos C, Marino A, Battaglini M, et al. Stimuli-responsive lipid-based magnetic nanovectors increase apoptosis in glioblastoma cells through synergic intracellular hyperthermia and chemotherapy. Nanoscale. 2018;11(1):72–88.
- Babincová M, Vrbovská H, Sourivong P, et al. Application of albumin-embedded magnetic nanoheaters for release of etoposide in integrated chemotherapy and hyperthermia of U87-MG glioma cells. Anticancer Res. 2018;38(5):2683–2690.
- Ohtake M, Umemura M, Sato I, et al. Hyperthermia and chemotherapy using Fe(Salen) nanoparticles might impact glioblastoma treatment. Sci Rep. 2017;7(1):42783.
- Zhao L, Yang B, Wang Y, et al. Thermochemotherapy mediated by novel solar-planet structured magnetic nanocomposites for glioma treatment. J Nanosci Nanotechnol. 2012;12(2):1024–1031.
- Hua M-Y, Liu H-L, Yang H-W, et al. The effectiveness of a magnetic nanoparticle-based delivery system for BCNU in the treatment of gliomas. Biomaterials. 2011;32(2):516–527.
- Mohammed MA, Syeda JTM, Wasan KM, et al. An overview of chitosan nanoparticles and its application in non-parenteral drug delivery. Pharmaceutics. 2017;9(4):53.
- Kuroiwa T, Noguchi Y, Nakajima M, et al. Production of chitosan oligosaccharides using chitosanase immobilized on amylose-coated magnetic nanoparticles. Process Biochem. 2008;43(1):62–69.
- Zhu L, Ma J, Jia N, et al. Chitosan-coated magnetic nanoparticles as carriers of 5-fluorouracil: preparation, characterization and cytotoxicity studies. Colloids Surf B Biointerfaces. 2009;68(1):1–6.
- Pandey A, Singh K, Patel S, et al. Hyaluronic acid tethered pH-responsive alloy–drug nanoconjugates for multimodal therapy of glioblastoma: an intranasal route approach. Mater Sci Eng C Mater Biol Appl. 2019;98:419–436.
- Maier-Hauff K, Ulrich F, Nestler D, et al. Efficacy and safety of intratumoral thermotherapy using magnetic iron-oxide nanoparticles combined with external beam radiotherapy on patients with recurrent glioblastoma multiforme. J Neurooncol. 2011;103(2):317–324.
- Grauer O, Jaber M, Hess K, et al. Combined intracavitary thermotherapy with iron oxide nanoparticles and radiotherapy as local treatment modality in recurrent glioblastoma patients. J Neurooncol. 2019;141(1):83–94.
- Iverson NM, Barone PW, Shandell M, et al. In vivo biosensing via tissue-localizable near-infrared-fluorescent single-walled carbon nanotubes. Nat Nanotechnol. 2013;8(11):873–880.
- Liu Y, Li L, Guo Q, et al. Novel Cs-based upconversion nanoparticles as dual-modal CT and UCL imaging agents for chemo-photothermal synergistic therapy. Theranostics. 2016;6(10):1491–1505.
- Kafa H, Wang J-W, Al-Jamal K. Current perspective of carbon nanotubes application in neurology. Int Rev Neurobiol. 2016;130:229–263.
- Hirschberg H, Madsen SJ. Cell mediated photothermal therapy of brain tumors. J Neuroimmune Pharmacol. 2017;12(1):99–106.
- Fernandez Cabada T, Sanchez Lopez de Pablo C, Martinez Serrano A, et al. Induction of cell death in a glioblastoma line by hyperthermic therapy based on gold nanorods. Int J Nanomedicine. 2012;7:1511–1523.
- Wang H, Mu Q, Revia R, et al. Iron oxide-carbon core-shell nanoparticles for dual-modal imaging-guided photothermal therapy. J Control Release. 2018;289:70–78.
- Landa FJO, Deán-Ben XL, Sroka R, et al. Volumetric optoacoustic temperature mapping in photothermal therapy. Sci Rep. 2017;7(1):9695.
- Eldridge BN, Bernish BW, Fahrenholtz CD, et al. Photothermal therapy of glioblastoma multiforme using multiwalled carbon nanotubes optimized for diffusion in extracellular space. ACS Biomater Sci Eng. 2016;2(6):963–976.
- Santos T, Fang X, Chen MT, et al. Sequential administration of carbon nanotubes and near-infrared radiation for the treatment of gliomas. Front Oncol. 2014;4:180.
- Wang C-H, Chiou S-H, Chou C-P, et al. Photothermolysis of glioblastoma stem-like cells targeted by carbon nanotubes conjugated with CD133 monoclonal antibody. Nanomedicine. 2011;7(1):69–79.
- Qian M, Du Y, Wang S, et al. Highly crystalline multicolor carbon nanodots for dual-modal imaging-guided photothermal therapy of glioma. ACS Appl Mater Interfaces. 2018;10(4):4031–4040.
- Casanova-Carvajal O, Urbano-Bojorge AL, Ramos M, et al. Slowdown intracranial glioma progression by optical hyperthermia therapy: study on a CT-2A mouse astrocytoma model. Nanotechnology. 2019;30(35):355101.
- Christie C, Madsen SJ, Peng Q, et al. Photothermal therapy employing gold nanoparticle-loaded macrophages as delivery vehicles: comparing the efficiency of nanoshells versus nanorods. J. Environ Pathol Toxicol Oncol. 2017;36(3):229–235.
- Baek SK, Makkouk AR, Krasieva T, et al. Photothermal treatment of glioma; an in vitro study of macrophage-mediated delivery of gold nanoshells. J Neurooncol. 2011;104(2):439–448.
- You J, Shao R, Wei X, et al. Near-infrared light triggers release of Paclitaxel from biodegradable microspheres: photothermal effect and enhanced antitumor activity. Small. 2010;6(9):1022–1031.
- Gherman A, Boca S, Vulpoi A, et al. Plasmonic photothermal heating of gold nanostars in a real-size container: multiscale modelling and experimental study. Nanotechnology. 2020;31(12):125701.
- Xu HL, ZhuGe DL, Chen PP, et al. Silk fibroin nanoparticles dyeing indocyanine green for imaging-guided photo-thermal therapy of glioblastoma. Drug Deliv. 2018;25(1):364–375.
- Zeng X, Wang Q, Tan X, et al. Mild thermotherapy and hyperbaric oxygen enhance sensitivity of TMZ/PSi nanoparticles via decreasing the stemness in glioma. J Nanobiotechnol. 2019;17(1):47.
- Chitgupi U, Qin Y, Lovell JF. Targeted nanomaterials for phototherapy. Nanotheranostics. 2017;1(1):38–58.
- Sheikh Mohamed M, Poulose AC, Veeranarayanan S, et al. Plasmonic fluorescent CdSe/Cu2S hybrid nanocrystals for multichannel imaging and cancer directed photo-thermal therapy. Nanoscale. 2016;8(15):7876–7888.
- Mohamed MS, Veeranarayanan S, Poulose AC, et al. Type 1 ribotoxin-curcin conjugated biogenic gold nanoparticles for a multimodal therapeutic approach towards brain cancer. Biochim Biophys Acta. 2014;1840(6):1657–1669.
- Qian W, Qian M, Wang Y, et al. Combination glioma therapy mediated by a dual-targeted delivery system constructed using OMCN-PEG-Pep22/DOX. Small. 2018;14(42):e1801905.
- Day ES, Zhang L, Thompson PA, et al. Vascular-targeted photothermal therapy of an orthotopic murine glioma model. Nanomedicine (Lond). 2012;7(8):1133–1148.
- Christie C, Madsen S, Peng Q, et al. Macrophages as nanoparticle delivery vectors for photothermal therapy of brain tumors. Ther Deliv. 2015;6:371–384.
- Hao Y, Zhang B, Zheng C, et al. The tumor-targeting core–shell structured DTX-loaded PLGA@Au nanoparticles for chemo-photothermal therapy and X-ray imaging. J Controlled Release. 2015;220:545–555.
- Botella P, Ortega Í, Quesada M, et al. Multifunctional hybrid materials for combined photo and chemotherapy of cancer. Dalton Trans. 2012;41(31):9286–9296.
- Shibata S, Shinozaki N, Suganami A, et al. Photo-immune therapy with liposomally formulated phospholipid-conjugated indocyanine green induces specific antitumor responses with heat shock protein-70 expression in a glioblastoma model. Oncotarget. 2019;10(2):175–183.
- Dysart JS, Singh G, Patterson MS. Calculation of singlet oxygen dose from photosensitizer fluorescence and photobleaching during mTHPC photodynamic therapy of MLL cells. Photochem Photobiol. 2005;81(1):196–205.
- Muller PJ, Wilson BC, Lilge LD, editors. et al. Photofrin photodynamic therapy for malignant brain tumors. Optical Methods for Tumor Treatment and Detection: Mechanisms and Techniques in Photodynamic Therapy X. Bellingham, WA: International Society for Optics and Photonics; 2001.
- Wang F, Banerjee D, Liu Y, et al. Upconversion nanoparticles in biological labeling, imaging, and therapy. Analyst. 2010;135(8):1839–1854.
- Tsai YC, Vijayaraghavan P, Chiang WH, et al. Targeted delivery of functionalized upconversion nanoparticles for externally triggered photothermal/photodynamic therapies of brain glioblastoma. Theranostics. 2018;8(5):1435–1448.
- McDannold N, Clement GT, Black P, et al. Transcranial magnetic resonance imaging-guided focused ultrasound surgery of brain tumors: initial findings in 3 patients. Neurosurgery. 2010;66(2):323–332. discussion 332.
- Coluccia D, Fandino J, Schwyzer L, et al. First noninvasive thermal ablation of a brain tumor with MR-guided focusedultrasound. J Ther Ultrasound. 2014;2(1):17.
- Park JW, Jung S, Jung TY, editors. et al. Focused ultrasound surgery for the treatment of recurrent anaplastic astrocytoma: A preliminary report. AIP Conference Proceedings, AIP; 2006.
- Wan Q, Zou C, Hu D, et al. Imaging-guided focused ultrasound-induced thermal and sonodynamic effects of nanosonosensitizers for synergistic enhancement of glioblastoma therapy. Biomater Sci. 2019;7(7):3007–3015.
- Yoshida M, Kobayashi H, Terasaka S, et al. Sonodynamic therapy for malignant glioma using 220-kHz transcranial magnetic resonance imaging-guided focused ultrasound and 5-aminolevulinic acid. Ultrasound Med Biol. 2019;45(2):526–538.
- Suehiro S, Ohnishi T, Yamashita D, et al. Enhancement of antitumor activity by using 5-ALA-mediated sonodynamic therapy to induce apoptosis in malignant gliomas: significance of high-intensity focused ultrasound on 5-ALA-SDT in a mouse glioma model. J Neurosurg. 2018;129(6):1416–1428.
- Ohmura T, Fukushima T, Shibaguchi H, et al. Sonodynamic therapy with 5-aminolevulinic acid and focused ultrasound for deep-seated intracranial glioma in rat. Anticancer Res. 2011;31(7):2527–2533.
- Cha J, Jeon TW, Lee CG, et al. Electro-hyperthermia inhibits glioma tumorigenicity through the induction of E2F1-mediated apoptosis. Int J Hyperthermia. 2015;31(7):784–792.
- Bredlau AL, Motamarry A, Chen C, et al. Localized delivery of therapeutic doxorubicin dose across the canine blood–brain barrier with hyperthermia and temperature sensitive liposomes. Drug Deliv. 2018;25(1):973–984.
- Wismeth C, Dudel C, Pascher C, et al. Transcranial electro-hyperthermia combined with alkylating chemotherapy in patients with relapsed high-grade gliomas: phase I clinical results. J Neurooncol. 2010;98(3):395–405.
- Roussakow SV. Clinical and economic evaluation of modulated electrohyperthermia concurrent to dose-dense temozolomide 21/28 days regimen in the treatment of recurrent glioblastoma: a retrospective analysis of a two-centre German cohort trial with systematic comparison and effect-to-treatment analysis. BMJ Open. 2017;7(11):e017387.
- Schooneveldt G, Dobsicek Trefna H, Persson M, et al. Hyperthermia treatment planning including convective flow in cerebrospinal fluid for brain tumour hyperthermia treatment using a novel dedicated paediatric brain applicator. Cancers (Basel). 2019;11(8):1183.
- Byun YH, Gwak HS, Kwon JW, et al. Local recurrence of brain metastasis reduced by intra-operative hyperthermia treatment. Int J Hyperthermia. 2019;35(1):168–175.
- Rozumenko A, Kliuchka V, Rozumenko V, et al. Image-guided resection of glioblastoma in eloquent brain areas facilitated by laser surface thermal therapy: clinical outcomes and long-term results. Neurosurg Rev. 2018;41(4):1045–1052.
- Meenach SA, Shapiro JM, Hilt JZ, et al. Characterization of PEG-iron oxide hydrogel nanocomposites for dual hyperthermia and paclitaxel delivery. J Biomater Sci Polym Ed. 2013;24(9):1112–1126.
- Han X, Jing X, Yang D, et al. Therapeutic mesopore construction on 2D Nb2C MXenes for targeted and enhanced chemo-photothermal cancer therapy in NIR-II biowindow. Theranostics. 2018;8(16):4491–4508.
- Yang Z, Song J, Dai Y, et al. Self-assembly of semiconducting-plasmonic gold nanoparticles with enhanced optical property for photoacoustic imaging and photothermal therapy. Theranostics. 2017;7(8):2177–2185.
- Zhu M, Sheng Z, Jia Y, et al. Indocyanine green-holo-transferrin nanoassemblies for tumor-targeted dual-modal imaging and photothermal therapy of glioma. ACS Appl Mater Interfaces. 2017;9(45):39249–39258.