Abstract
The therapeutic application of heat is very effective in cancer treatment. Both hyperthermia, i.e., heating to 39–45 °C to induce sensitization to radiotherapy and chemotherapy, and thermal ablation, where temperatures beyond 50 °C destroy tumor cells directly are frequently applied in the clinic. Achievement of an effective treatment requires high quality heating equipment, precise thermal dosimetry, and adequate quality assurance. Several types of devices, antennas and heating or power delivery systems have been proposed and developed in recent decades. These vary considerably in technique, heating depth, ability to focus, and in the size of the heating focus. Clinically used heating techniques involve electromagnetic and ultrasonic heating, hyperthermic perfusion and conductive heating. Depending on clinical objectives and available technology, thermal therapies can be subdivided into three broad categories: local, locoregional, or whole body heating. Clinically used local heating techniques include interstitial hyperthermia and ablation, high intensity focused ultrasound (HIFU), scanned focused ultrasound (SFUS), electroporation, nanoparticle heating, intraluminal heating and superficial heating. Locoregional heating techniques include phased array systems, capacitive systems and isolated perfusion. Whole body techniques focus on prevention of heat loss supplemented with energy deposition in the body, e.g., by infrared radiation. This review presents an overview of clinical hyperthermia and ablation devices used for local, locoregional, and whole body therapy. Proven and experimental clinical applications of thermal ablation and hyperthermia are listed. Methods for temperature measurement and the role of treatment planning to control treatments are discussed briefly, as well as future perspectives for heating technology for the treatment of tumors.
Graphical Abstract
Schematic overview of clinically proven heating techniques used for various indications and tumor locations.
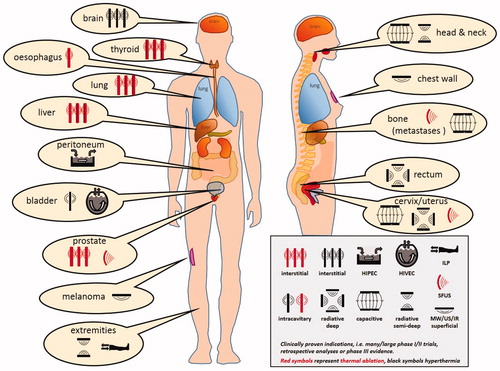
1. Introduction
Thermal medicine involves the manipulation of body or tissue temperatures for the treatment of diseases. The application of heat has a long history in the treatment of malignant tumors and was already used by the ancient Greeks. In the late nineteenth century, spontaneous regression of malignant tumors was reported in patients suffering from high fevers due to bacterial infections [Citation1]. Since then, heating techniques have been developed to apply more controlled heating to maximize the therapeutic effect while minimizing unwanted side effects. Therapeutic heating of tumor tissue can be roughly categorized as hyperthermia or thermal ablation.
Hyperthermia treatments aim to induce relatively mild tumor heating up to a maximum temperature of 45 °C. The therapeutic effect depends on the temperature and the duration of heating (thermal dose) [Citation2–4]. This is often expressed as the cumulative equivalent minutes at 43 °C (CEM43) [Citation5,Citation6], since the widely accepted target temperature is 43 °C for up to 1 h [Citation6]. The use of CEM43 is based on the Arrhenius relationship, describing the time–temperature dependent cytotoxic effect of hyperthermia [Citation7]. For short exposure times at temperatures below 43 °C, the cell survival versus exposure time curve shows a shoulder, which is indicative of accumulation of sub-lethal thermal damage. For increased exposure time, this shoulder turns into a constant rate of cell death, followed after several hours by a lower rate of cell death due to the development of thermotolerance. For heat exposure at temperatures above 43 °C, cell death occurs at a constant rate. Although the CEM43 thermal iso-effect dose concept has some limitations, it is commonly used as a practical normalization to convert a given time–temperature exposure to an equivalent exposure time at 43 °C [Citation5]. Hyperthermia is typically applied once or twice a week in combination with radiotherapy and/or chemotherapy schedules and has been shown to enhance the therapeutic effect of these adjuvant treatment modalities. Known mechanisms for this enhancement include inhibition of DNA damage repair, increased blood flow and reoxygenation [Citation8–12]. Furthermore, hyperthermia stimulates the immune response [Citation13]. Optimal dose differs for these mechanisms. Increased blood flow and reoxygenation become active at relatively low temperatures of ∼39 °C, peak around 41–42 °C, and reverse when temperatures exceed 44 °C [Citation14]. Effective inhibition of DNA damage repair requires higher temperatures (exceeding ∼41 °C) and strongly increases with increasing thermal dose [Citation15]. The combination of all mechanisms above effectively displays a dose–effect relationship within the hyperthermia temperature range. The applied timing of delivery is either simultaneous (often for chemotherapy) or sequential with a relatively short time interval between hyperthermia and radiotherapy/chemotherapy.
Thermal ablation aims at generation of temperatures over 50 °C for a few minutes in a single session to destroy tumor cells by heat alone. These excessive temperatures cause very rapid cell death by coagulation and protein denaturation, leading to both necrosis and apoptosis, depending on the dose. As explained in the previous paragraph, Arrhenius relationships can also be applied to thermal ablation to predict thermal damage and physical changes associated with thermal exposure in several tissue types [Citation16]. Depending on the mode of energy delivery, thermal ablation can be used as a minimally invasive alternative to surgical resection. However, in the tumor periphery beyond the border of immediate tissue coagulation, non-lethal, but hyperthermic temperatures are achieved and therefore the combination of thermal ablation with chemotherapy, radiotherapy, or immunotherapy, is also attracting growing clinical interest for effectively treating both the tumor and its periphery [Citation17–19]. represents the characteristics of hyperthermia and ablation in terms of temperature levels. Panel B shows a schematic representation of the clear division between the different realms of hyperthermia versus thermal ablation based on data presented in Dewhirst et al. [Citation20]. The dividing line shows that the exposure time needed to achieve cell death decreases with increasing temperatures.
Figure 1. (A) Characteristics of the three main treatment approaches in terms of temperature level. (B) Time to yield muscle fibrosis (continuous curve) or necrosis (dotted curve) at specific temperatures for hyperthermia and ablation (based on Dewhirst et al. [Citation20]).
![Figure 1. (A) Characteristics of the three main treatment approaches in terms of temperature level. (B) Time to yield muscle fibrosis (continuous curve) or necrosis (dotted curve) at specific temperatures for hyperthermia and ablation (based on Dewhirst et al. [Citation20]).](/cms/asset/a654b620-b27f-4c4b-9139-b6a0a74c1433/ihyt_a_1779357_f0001_c.jpg)
Over the past several decades increasing knowledge gained about the mechanisms of action of both hyperthermia and thermal ablation have had positive effects on treatment outcome in several randomized clinical trials for both hyperthermia and thermal ablation [Citation21]. Based on these positive clinical trials, hyperthermia and thermal ablation are recognized in clinical oncology for a number of tumor indications. Hyperthermia is routinely used for locally advanced cervical cancer patients unfit for chemotherapy, for recurrent breast cancer, non-muscle invasive bladder cancer and soft tissue sarcoma. Thermal ablation is currently in routine clinical use for a large number of indications, including hepatocellular carcinoma, renal cell carcinoma (the most common type of kidney cancer), primary and secondary lung tumors, prostate cancer, non-surgical liver metastases and adrenal metastases.
The realization of good clinical results requires high quality heating equipment, and thus a significant amount of research has been undertaken toward the development of robust and reliable heating devices. The tumor location is often decisive in selecting whether invasive or external heating is preferred. Several types of instruments, antennas and systems have been proposed. These vary widely in physical mechanism of energy transduction, techniques for energy delivery, heating depth and directional control of the delivered energy, as well as in the size of the volume that can be heated [Citation22]. These developments have resulted in dedicated heating equipment for specific tumor sites and anatomical locations, driving significant progress in both the control and technology of heating.
In this review we present an overview of equipment used clinically for hyperthermia and thermal ablation. Different techniques of heating are first explained, followed by an overview of equipment for local, locoregional and whole body heating with a description of tumor sites and clinical applications. Since monitoring is important to ensure treatment quality and thermal dose delivery, control methods involving thermometry and treatment planning are also briefly summarized. Finally, future perspectives are discussed.
2. Methods of heating
Hyperthermia and thermal ablation can be produced using a variety of techniques. Clinically used heating techniques are electromagnetic heating, ultrasound, hyperthermic perfusion and conductive heating. The principles of these techniques are discussed below and a schematic representation of the heating mechanisms is shown in .
Figure 2. Schematic representation of the heating mechanisms of different clinical heating techniques: Electromagnetic (i.e., capacitive, radiofrequency, microwave, infrared and laser heating), focused ultrasound, hyperthermic perfusion and conductive heating.

2.1. Electromagnetic heating
Electromagnetic heating techniques apply a high frequency alternating sinusoidal electromagnetic (EM) field generated using one or more antennas. These EM fields cause dielectric heating by molecular dipole rotation/polarization/vibration in the MHz range and ionic conduction in the kHz range. Polar molecules (e.g., water) have an electric dipole moment and therefore these molecules align continuously with the alternating field. Electric forces cause rotating molecules to push, pull, and collide with other molecules, thereby distributing the energy to adjacent molecules and atoms, causing dielectric heating. In conduction, ions in tissue oscillate due to the forces exerted by the electric current. This current faces internal resistance because of the collisions of charged particles with neighboring molecules or atoms, causing dielectric heating. Tissue heating is dominated by ionic conduction in the extracellular fluid for lower frequencies (<1 MHz) with the cell membranes acting as isolators, and by dipole polarization at higher frequencies (>1 MHz). For frequencies >1 MHz the cell membranes become permeable to the E-fields and the microscopic structure of tissues can be neglected [Citation23].
Electromagnetic heating techniques can be subdivided into categories differing in frequency, wavelength and penetration depth. These include in ascending order of frequency (and descending penetration depth) radiofrequency (either capacitive or radiative), microwave heating, infrared and laser heating. Many microwave systems operate at an industrial, scientific and medical (ISM)-frequency which permits the use outside a Faraday cage for shielding.
2.1.1. Capacitive radiofrequency heating
Capacitive heating is achieved using metal electrodes that are coupled to a high power RF generator, operating at frequencies of 8, 13.56 or 27.12 MHz. Electrodes are usually applied in pairs with the target region positioned between the electrodes, coupled to water bolus bags or other suitable media to transfer the current into the body. These boluses are also applied for skin cooling. An electric field is applied between the electrodes and power is deposited in the tissue as a result of the alternating voltage field [Citation24]. Power can be steered by using different sized electrodes, which concentrate current fields under the smallest electrode, such that both superficial and deep-seated tumor locations can be treated. The energy absorption in tissue by dielectric heating depends on the electrical conductivity and permittivity [Citation25], which vary between different tissue types [Citation26], resulting in an inhomogeneous power distribution. Therefore, excessive temperatures (hot spots) can occur at tissue interfaces, and due to the orientation of the main electric field component, which is perpendicular to fat layers, especially excessive heating of fat tissue can be treatment limiting when using this technique [Citation27–29]. Therefore, capacitive heating becomes less effective with increasing thickness of the fat layer and heating of deep-seated tumors can be challenging [Citation30–32].
Some variants of this heating technique exist, including coplanar electrodes and intraluminal/intracavitary electrodes placed in catheters, which create a capacitor between the electrode and tissue, with electrodes operating as balanced pairs or as individual applicators coupled to an external ground plane.
2.1.2. Radiative radiofrequency and microwave heating
Clinical radiative radiofrequency (RF) hyperthermia is applied using extracorporally placed antennas with operating frequencies ranging from 60 MHz to 150 MHz. RF hyperthermia is used for deep-seated tumor locations (e.g., pelvic tumors) because in this frequency range the wavelength is 25–60 cm in muscle tissue, yielding a large penetration depth of the electromagnetic energy. Microwave (MW) hyperthermia is usually applied for superficial or intraluminal/intracavitary tumor sites. Typical free ISM frequencies applied for microwave hyperthermia antennas are 433 MHz (Europe), 915 MHz (USA) and 2450 MHz. To couple the energy into tissue, a water bolus is usually applied between the antenna(s) and the tissue, for both radiofrequency and microwave heating; this water bolus can simultaneously be used for skin cooling when treating deep-seated tumors, or heating in the case of superficial tumor locations. As a result of inhomogeneous energy absorption in different tissues hot spots can occur at tissue interfaces.
Interstitial RF or MW needles are used for minimally invasive ablation as an alternative to surgery, depending on the size and location of the tumor. Needle electrodes are inserted under image guidance (CT, ultrasound or MRI) into the target tissue. RF ablation applies an alternating current in the range of 350–500 kHz; MW ablation frequencies are typically 915 MHz or 2.45 GHz. The main difference between RF and MW ablation is that RF ablation induces areas of high current density mainly near the electrodes, while microwave ablation causes dielectric heating in a volume around the applicator. A rapid decrease of power density away from the applicator is a common trait for both RF and MW ablation techniques [Citation33].
Radiofrequency and microwave hyperthermia and ablation devices can use a single electrode or antenna, an array or a phased array of antennas. With a 2 D array of multiple antennas larger tumor areas can be heated. With a 3 D phased array, in which multiple antennas are positioned in one or more rings around the patient, power steering and focusing of the electromagnetic energy into the tumor is possible (also at deep-seated locations) by adequate selection of phases and amplitudes to create constructive interference between the electromagnetic fields. As a result of the locoregional heating induced by this technique, some temperature rise in normal tissues is inevitable. However, this is usually not a problem, as long as excessive heating (hot spots) is avoided. This can be realized by adequate (destructive) interference [Citation34].
2.1.3. Infrared and laser
Infrared (IR) heating applies infrared lamps (frequency >300 GHz) to heat tissues. IR energy is strongly absorbed due to the presence of O–H bonds in water. As the penetration depth of this technique is typically less than 1 cm, it is suitable for very superficial heating. Infrared heating is also applied for whole body hyperthermia, using an isolated cabin to avoid heat loss [Citation35]. Furthermore, optical fiber guided laser light is applied for local ablation of tissue. Interstitial laser ablation allows the delivery of laser energy directly into the tumor tissue, which maximizes penetration and effectiveness in the tumor, while minimizing damage to surrounding healthy tissues [Citation36].
2.2. Ultrasound
Ultrasound (US) heating employs acoustic energy at frequencies 0.5–10 MHz to heat tissues and can be used to induce either hyperthermia or thermal ablation [Citation37,Citation38]. In contrast to diagnostic US imaging, where fields are usually weakly focused, ultrasound transducers used for thermal therapy are often designed to focus pressure waves to converge on a specific focal zone. Heat is generated by frictional losses due to molecular collisions resulting from the applied US pressure wave. A variety of planar devices (with or without lenses), bowl-shaped sources, focused multi-transducer phased arrays and interstitial US applicators are used. For focused ultrasound (FUS), the ellipsoidal focal spot typically has dimensions of a few mm in diameter, up to ∼1 cm in length, which decreases with increasing frequency and aperture. US technology enables excellent spatial and dynamic control of the power and temperature distribution [Citation39] due to its relatively short wavelength (a few millimeters) and allows deep penetration in soft tissues, provided an US window is present. These properties enable conformal tumor heating for both ablation and hyperthermia by mechanically or electronically scanning a highly focused US beam through the target region [Citation40–42].
In addition to thermal effects HIFU also damages cellular structures by cavitation, i.e., implosion of microbubbles formed during HIFU [Citation43–45]. Due to the pressure waves, gas bubbles present in the blood stream start to oscillate in the sound field (stable cavitation) inducing mechanical effects such as the enhancement of the permeability of cell membranes or blood vessels. The latter can be used to open the blood brain barrier locally, allowing drug compounds to extravasate into the brain tissue [Citation46]. Ultrasound-induced cavitation can actively transport and improve the distribution of therapeutic agents in tumors. Injection of microbubbles, polymeric nanocups or other nanoparticles can be used to realize stable and inertial cavitation, thereby offering a considerable potential for enhanced drug delivery and treatment monitoring in oncological and other biomedical applications [Citation47–50].
Application of US for soft tissue heating requires clear acoustical access to the target tissue, which should not be obstructed by bone, air pockets or strongly absorbing material, such as, for example feces in the bowels. As the absorption coefficient for ultrasound in bones is about 50 times higher than in soft tissue, any bone in the near field would lead to off-target heating. The acoustic impedance mismatch at the air/tissue interface leads to strong reflection and scattering of ultrasound at the air pocket, which may cause local heating and unintentional ablation of adjacent tissue. On the other hand, the strong absorption of US in bone makes it a perfect modality for bone tumor ablation [Citation51,Citation52], but other lesions may not be accessible for FUS, or require carefully planned applicator positioning to avoid normal tissue hot spots during treatment of deep seated pelvic and abdominal tumor sites.
2.3. Hyperthermic perfusion
Selected anatomical sites are suitable for perfusional hyperthermia techniques, generally combined with chemotherapy. One approach is the direct infusion of heated perfusate into the vascular system, for example for hyperthermic isolated limb perfusion (ILP). ILP involves temporarily connecting the circulatory system in the limb of the patient to an external pump to distribute both heat and drugs to the tumor target region, achieving relatively uniform heating and drug delivery in that region. Another approach is to circulate a hyperthermic carrier solution combined with chemotherapeutic agents inside body cavities to provide heating and drug delivery to tumors in the surface of the cavity. An example is Hyperthermic IntraPeritoneal Chemotherapy (HIPEC) which involves circulation of a heated chemotherapeutic solution in the peritoneal cavity to eradicate any microscopic tumors left behind after surgical removal of macroscopic tumor mass [Citation53]. Hyperthermic intrathoracic chemotherapy (HITHOC) is a similar technique for intrathoracic lesions [Citation54]. Hyperthermic Intra-Vesical Chemotherapy (HIVEC®) is another perfusion technique in which the bladder wall is treated by circulating a heated chemotherapy solution inside the bladder [Citation55].
2.4. Conductive heating
Conductive heating was the earliest clinically applied hyperthermia technique, as it was used by ancient Greeks and Egyptians to treat superficial malignancies. One of the earliest post-industrial revolution hyperthermia techniques was developed by Westermark, using water heated metal coils at 42–44 °C to treat cervical carcinoma in the late nineteenth century [Citation56]. More recently, interstitial implants of metal needles with hot water and palladium–nickel thermoseeds have been developed and applied clinically [Citation57]. These conductive heating techniques became less popular because the thermal penetration depth is governed by heat diffusion and perfusion, and therefore small in comparison to other heating techniques.
Magnetic nanoparticles (MNPs) were first proposed by Gilchrist et al. [Citation58]. Further developments have resulted in intriguing materials for clinical conductive heating [Citation59–61]. When exposed to an external magnetic field, the magnetic materials will respond by aligning their atomic moments with the field, thus producing a magnetization that persists for a characteristic duration after the external magnetic field is removed [Citation62,Citation63]. Heat is generated via magnetic hysteresis, a loss mechanism for which the amount of energy released depends on the frequency and amplitude of the applied magnetic field, as well as on the intrinsic magnetic properties of the nanoparticle. This dependency is complex and methodology for characterizing the heating is not standardized, making prediction of the amount of heat generated by MNPs challenging [Citation64]. Generally, the relevant frequency range for MNP hysteresis heating is between 0.1 and 1 MHz. However, for clinical MNP hyperthermia a more limited range of about 0.1–0.2 MHz is used to limit excessive off-target tissue heating from induced eddy currents [Citation64]. MNPs are typically suspended in a biocompatible fluid, which enables direct heating of the target tissue, thereby limiting contact with normal tissues [Citation65,Citation66]. Due to their size (∼100 nm), MNPs are colloids, which can be made stable in aqueous suspensions suitable for injection by using appropriate coatings [Citation65–69].
3. Selection and use of equipment based on clinical requirements
Existing heat treatment approaches can be subdivided into three categories as noted earlier: local heating, locoregional heating and whole-body heating. The exposure time to achieve tumor cell death decreases with increasing temperatures (). Hyperthermia treatment aims to provide synergistic effects with chemotherapy and/or radiotherapy in a typical 1 h treatment, while thermal ablation is applied in a single short session lasting up to a few minutes. A general summary of heating techniques, goal temperatures, treatment scheme and duration is given in and in this section an overview of clinical equipment for treatment of local disease (i.e., local and locoregional heating) and treatment of wide-spread disease (i.e., whole-body heating) is presented. At the end of each subsection, a brief description of clinical applications is provided. These are summarized per tumor site in . presents a schematic overview of the heating depth from the skin and the tumor/target size that can be heated with various heating techniques. summarizes the principles of the different heating techniques graphically.
Figure 3. Left: schematic overview of the heating depth from the skin and the tumor/target size that can be heated with various noninvasive heating techniques. Right: Schematic overview of the tumor/target size that can be heated with various invasive heating techniques.
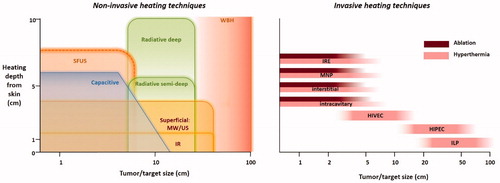
Table 1. General summary of heating techniques, typical goal temperatures, treatment scheme and duration, as well as experimental (italic) and clinically proven applications.
Table 2. Overview of clinical applications of local, locoregional and whole-body heating techniques.
3.1. Local heating techniques
Local heating aims at heating the tumor target volume itself, with no (or minimal) heating of normal tissue surrounding the target. Tumor selective heating can be achieved with invasive applicators for deep seated locations, as used for interstitial heating, electroporation and nanoparticles, or with external applicators for more superficially located target regions. Scanned focused ultrasound with external applicators is the only noninvasive method capable of achieving local and conformal heating at deep seated locations. The different techniques and guiding rationale in each case are discussed in the following subsections.
3.1.1. Interstitial heating
Equipment
Interstitial heating uses arrays of needle shaped applicators implanted into the target volume using catheters. Extensive research has been performed on interstitial heating techniques, resulting in several pre-clinical/research prototypes and commercially available products [Citation227–231]. Investigations include hot sources [Citation170,Citation232–234], laser sources [Citation203,Citation235], monopole, dipole, slot or helical coil microwave antennas [Citation171,Citation236–238], resistively-coupled radiofrequency local current field electrodes [Citation239], capacitively coupled radiofrequency catheter-based electrodes [Citation240–242] and ultrasound transducers [Citation243]. More detailed reviews of interstitial heating systems, including detailed system specifications, can be found in the literature [Citation227–231].
Currently, ablative applications are more common, although initially applications of interstitial heating mainly focused on hyperthermia combined with brachytherapy or a brachytherapy boost, where the catheters used to deliver the radiation source can also be used for hyperthermia applicators and thermometry. Energy deposition for all techniques is confined to the close proximity of the individual applicators due to the line/point source nature of interstitial sources. The clinical challenge for interstitial hyperthermia is thus ensuring homogeneous hyperthermic temperatures. This requires close spacing of multiple small applicators within 1–2 cm apart to counterbalance the impact of heat loss due to (high) perfusion. This constrains the application to heating of relatively small tumor volumes [Citation108].
When interstitial radiofrequency electrodes are used, the heating generated by the RF currents between electrodes is strongly influenced by local electrical tissue properties and the location and alignment of the other electrodes [Citation244]. These drawbacks can be overcome by using electrodes operating from within low-loss catheters (e.g., Nylon or Teflon), creating high impedance capacitive coupling across the catheter wall, as in the 27 MHz multiple electrode current source (MECS) system [Citation240–242]. A single probe consists of multiple independent electrodes, each 10–20 mm long. This enables 3 D steering of the power deposition. Treatment monitoring and feedback is provided by multi-sensor thermocouple probes, integrated into the electrodes. The power and polarity of the electrodes can be modified to optimize the heating pattern.
Interstitial microwave antennas provide a better penetration depth than radiofrequency electrodes. The most clinically used microwave interstitial hyperthermia system is the commercially available BSD-500 interstitial/superficial system, involving a 915 MHz modified dipole antenna system from BSD – Pyrexar [Citation245]. An 8-channel generator feeds into a 3- or 4-way power splitter to which up to 24 microwave antennas, inserted in standard Celcon or Nylon brachytherapy catheters, can be connected. Depending on the insertion depth, the effectively heated region can be up to ∼5 cm in axial length. The antennas can operate in a conservative asynchronous mode or in a synchronous mode. In synchronous mode, the phase of each antenna can be modified with respect to the others to optimize the homogeneity of the power distribution. Up to 8 channels can be used for temperature monitoring using thermistor sensors [Citation245].
The clinical challenge of interstitial ablation is to ensure complete tumor ablation, while avoiding thermal damage to surrounding healthy tissues. Catheter-mounted ultrasound transducers have been explored for interstitial ablation of tissue [Citation246]. The radiating transducer portion can be segmented into separate tubular elements along the catheter length and even into separate sectors around the tube surface. Each sector or segment has individual power control, which provides precise control over both the angular and axial specific absorption rate (SAR) distribution [Citation37,Citation229,Citation243]. This concept has been further explored to develop a probe for transurethral treatment of prostate cancer allowing full or focal gland ablation under MR-guidance [Citation247]. The imaging is used for treatment planning as well as for near-real time temperature mapping during therapy with temperature maps being fed back to the system control for modulating power and device rotation speed [Citation172]. The final CE and FDA approved device, TULSA-PRO was developed and marketed by Profound Medical (Mississauga, Canada). Transrectal US devices, which provide a high degree of spatial control have also been developed [Citation248–250]. However, dwell times between sonications are required to protect the rectal wall from thermal damage, resulting in a relatively long treatment time. The advantage of transurethral US devices is that US is delivered from within the prostate gland, enabling continuous sonication and the use of unfocused beams for reduced treatment times [Citation247].
Radiofrequency ablation (RFA) techniques have matured to facilitate ablation of larger tumors by introducing internal cooling, bipolar, straight and umbrella-shaped clustered probes and combinations of these design elements as strategies to maximize the size of the coagulation zone [Citation251]. However, RFA at high power settings can result in charring of tissue immediately surrounding the electrode. This charring forms an effective insulator, thus limiting the size of the ablated region. Available monopolar RFA devices include the Cool‐tip Tyco (Tyco‐Valleylab, Boulder, CO, USA) and Radionics (Radionics, Burlington, MA, USA), the multilined RITA StarBurst (RITA Medical Systems, Inc., Mountain View, CA, USA) and RF 3000 Boston LeVeen needles (Boston Scientific Corporate, Natick, MA, USA). Celon Olympus (Medical and Industrial Equipment, Southend‐on‐Sea, Essex, UK) is a well-known producer of bipolar and multipolar devices. The circumferential HALO360+ bipolar RFA system and focal HALO90 (Covidien GI Solutions, formerly BÂRRX Medical) were developed for ablating the esophagus.
Microwave ablation (MWA) is deemed capable of creating larger and more spherical ablation zones than RFA. Applicator cooling and an increase in the number of antennas are used to achieve a larger and a more spherical ablation zone [Citation252]. For ablation of tissues with low electrical conductivity and poor thermal conduction, such as lung tumors, MWA is preferred to RFA. These characteristics do not degrade the volume heating of microwaves, allowing for larger ablation zones than RFA. MWA also allows more energy to be deposited more quickly than with RFA. Although this allows ablation of larger targets, the shape of the coagulation zone becomes less predictable, which increases the risk of adverse effects as intravascular steam burns outside the target. MWA devices operating at 2450 MHz and above are applied to create an ablation zone that is smaller and more spherical than the zone with devices operating at 915 MHz [Citation220,Citation253,Citation254]. Available devices at 2450 MHz include the cooled 14 gauge mini-choked HS AMICA (HS Hospital Service, Rome, Italy) [Citation215,Citation255], the Acculis (AngioDynamics, NY, USA) [Citation216], the Solero (AngioDynamics, NY, USA), the Emprint Ablation System (Medtronic Inc, Minneapolis, MN, USA, formerly Covidien) [Citation217,Citation221] and the NeuWave Certus Microwave Ablation System (NeuWave Medical, Madison, Wisconsin) [Citation256]. For a more detailed overview of 915/2450 MHz devices the reader is referred to Lubner et al. [Citation142].
For both in RFA and MWA, the proximity of large blood vessels can cause cool tracks due to their long thermal equilibration length compared to the small lesion size [Citation257]. This convective cooling effect counters the heat generation effects of the ablation devices and requires a modified ablation strategy, such as multi-applicator techniques, to optimize clinical outcome [Citation218].
Besides RF, MW and US applicators, laser techniques are also used for thermal ablation. Laser Interstitial Thermal Therapy (LITT) uses laser light transmitted by an optical fiber which is converted into thermal energy and damages tissue in the target volume. The probe is often cooled to prevent tissue vaporization due to excessively high temperatures near the applicator [Citation258]. An advantage of LITT is that it allows more accurate and safer ablation near critical structures than radiofrequency and microwave ablation. Commercially available systems include the Visualase Thermal Therapy System (Medtronic Inc, Minneapolis, MN) and the NeuroBlate LITT System (Monteris Medical Corporation, Plymouth, MN, USA). The Visualase Thermal Therapy System uses a diffusing fiber optic tip probe (980 nm at 15 W) combined with saline cooling and MRI temperature feedback [Citation259]. Besides diffusing tip probes, the NeuroBlate LITT System provides a gas-cooled side-firing directional diode solid-state laser in the Nd-YAG range (1064 nm at 12 W). Control software-actuated rotational and linear movement is used to achieve spatially controlled tumor ablation guided by real-time MRI temperature monitoring [Citation203].
Clinical applications of interstitial heating
Clinical use of interstitial hyperthermia includes mainly sites which are also considered suitable for brachytherapy such as prostate cancer, head and neck and brain tumors [Citation108–110,Citation204,Citation205,Citation228,Citation260,Citation261]. In a phase II/III randomized trial 79 glioblastoma patients were randomized to receive external beam radiotherapy (59.4 Gy; 1.8 Gy daily fractions), combined with a brachytherapy boost (60 Gy at 0.40–0.60 Gy/h) ± 30 min interstitial hyperthermia at 915 MHz immediately before and after brachytherapy. Two year survival was 31% for the hyperthermia group vs. 15% for the brachytherapy alone group [Citation110]. A retrospective analysis of 197 confirmed osteoid osteoma lesions treated with RFA showed this is a safe and effective technique. Two-year follow-up data were available for 126 procedures and showed 89% complete relief of symptoms. The success rate was 91% for initial treatments, and 60% for recurrences (p < 0.001) [Citation143]. The Surveillance versus Radiofrequency Ablation (SURF) trial randomized 136 patients with Barrett Esophagus to RFA (n = 68) or surveillance (n = 68) and found that RFA reduced the risk of progression to high-grade dysplasia or adenocarcinoma to 1.5% for RFA vs 26.5% for control (95% CI, 14.1%–35.9%; p < 0.001) and the risk of progression to adenocarcinoma to 1.5% for RFA vs 8.8% for control (95% CI, 0%–14.7%; p = 0.03) [Citation144]. A position paper by an international panel of ablation experts established the status of RFA in thermal ablation of colorectal liver metastases and recommended best practice based on 15 papers, each reporting on at least 50 patients, yielding a total of 1613 patients for evaluation. The data thus obtained allowed analysis of various relevant treatment parameters, including size of the tumor lesions that can be treated successfully, which is typically <3 cm [Citation262].
RFA and MWA are both widely used to ablate thoracic and gastrointenstinal tumors, e.g., liver [Citation142,Citation145,Citation146,Citation263], lung [Citation142,Citation147–149], thyroid [Citation150–153], kidney [Citation154,Citation155], breast [Citation156], esophagus [Citation144,Citation157] and pancreas [Citation158]. MWA is deemed capable of creating larger and more spherical ablation zones than RFA and thus clinical results of MWA are comparable to RFA for smaller lesions, but may be better for larger lesions [Citation145,Citation146]. A randomized controlled trial involving 76 patients with hepatocellular carcinoma treated with RFA and 76 patients treated with MWA showed that at 2 years 6/98 lesions (6%) had local tumor progression in the MWA arm, versus 12/104 (12%) in the RFA arm (p = 0.27) [Citation159]. Better results for MWA versus RFA have also been reported for lung tumors [Citation160]. Vogl et al. [Citation161] found in a retrospective analysis of 109 patients with colorectal lung metastases treated with LITT (21 patients, 31 ablations), RFA (41 patients, 75 ablations), and MWA (47 patients, 125 ablations) that MWA outperformed LITT and RFA in obtaining local tumor control. Narsule et al. [Citation162] found both RFA and MWA are a viable option for inoperable patients with early stage non-small cell lung cancer (NSCLC). MW ablation was also found to be safe, feasible, prevent hemorrhage and capable of eradicating tumors in treatment of hepatic adenomas [Citation219]. A recent meta-analysis concluded that transarterial chemoembolization is more successful when combined with MWA for unresectable hepatocellular carcinoma with tumor size >5 cm [Citation163].
Clinical use of LITT in brain tumors appears to be safe and to result in better gross total resection combined with less neurologic morbidity than with surgical resection [Citation164,Citation264]. A multicenter prospective study showed that LITT stabilized the Karnofsky score, preserved quality of life and cognition, and had a steroid-sparing effect in salvage treatment of patients showing progressive brain metastases after stereotactic radiosurgery [Citation165]. LITT is thus currently frequently used as a salvage therapy for treatment of patients with recurrent malignant gliomas [Citation206]. Laser ablation is feasible for bladder cancer as an alternative to transurethral bladder resection, although it remains unclear whether results are comparable due to the lack of randomized trials [Citation166]. Laser ablation also provides a safe and effective treatment of obstructive benign prostatic disease, as demonstrated in a randomized study [Citation167,Citation168]. Clinical outcome is equivalent to transurethral resection of the prostate (TURP), but with a more favorable toxicity profile; major intraoperative toxicity was absent [Citation167,Citation168].
3.1.2. Scanned focused ultrasound
Equipment for ablation
US and MR guided scanned HIFU is used for thermal ablation of tumors in a steadily growing number of applications [Citation265]. During treatment, a piezoelectric ultrasound transducer is used to create a highly concentrated focal spot of acoustic energy. This HIFU focal spot is moved through the target region under US or MR guidance, with the aim of inducing temperatures exceeding ∼60 °C, which result in nearly instantaneous tissue coagulation in the focus while creating minimal thermal damage in the tissue around the focal volume. It is important that sufficient cooling time typically of a few minutes is ensured between sonication at two adjacent locations to prevent prefocal thermal damage. HIFU thus provides a noninvasive form of thermal tumor ablation with a 5–10% lower risk of side effects than for other methods [Citation266]. The heating is localized to the focal volume, and, using long focal length transducers, also more deep-seated locations can be treated. Available CE or FDA approved devices include the Sonablate (SonaCare Medical, Charlotte, NC) and Ablatherm or Focal One® (EDAP TMS, Lyon, France) and the TULSA-PRO (Profound Medical Inc., Mississauga, Canada) for prostate tissue ablation, the Sonalleve system (Profound Medical Inc., Mississauga, Canada) and the ExAblate (InSighTec, Haifa, Israel) for both malignant and benign abdominal tumors and bone metastases. The ExAblate Neuro system uses dedicated phased-array transducers, which eliminates the need for a craniotomy when treating the brain. Up to 1024 ultrasound transducer elements mounted on a ‘helmet’ heat and ablate a deep brain target with no surgical incisions, guided by MR imaging. FDA approval was received for ablation of brain tissue for treatment of central tremor [Citation173,Citation267].
Equipment for hyperthermia
In principle MR guided HIFU is also capable of providing a temperature elevation in the mild hyperthermia range over an extended period of time (15–60 min), but this application is still experimental. HIFU guided by noninvasive MR thermometry is presently pursued primarily to induce local mild hyperthermia as a trigger for localized tumor-specific drug release from drugs encapsulated in thermosensitive liposomes [Citation268]. Phantom tests with the ExAblate 2100 HIFU ablation system (InSightec Ltd, Haifa, Israel) [Citation269,Citation270] and preclinical tests with the Sonalleve system (Profound Medical Inc., Mississauga, Canada) have demonstrated the feasibility of inducing a stable MR controlled temperature rise [Citation271–273] resulting in significantly enhanced ThermoDox delivery in mouse [Citation274], rat [Citation275], rabbit [Citation276] and pig models [Citation277]. Using the electronic beam steering of a single HIFU focus limits the size of the maximum volume with a stable elevated temperature level to a few cubic centimeters. Larger volumes – as needed for human treatments – can be heated stably with multi-focus HIFU systems [Citation278], or by a combination of electronic beam steering with a mechanical repositioning of the HIFU transducer [Citation42].
Clinical applications for HIFU
Good clinical results achieved in benign and malignant tumors include ablation of uterine fibroids [Citation174–176,Citation207], palliation of pain from bone metastases [Citation177] and ablation of prostate tissue [Citation178–183] have resulted in approval of both MR and US guided HIFU for the treatment of these sites in several countries. A prospective multicentre study of 625 patients undergoing HIFU for clinically significant nonmetastatic prostate cancer reported a 5 year failure-free survival, metastasis-free survival, cancer-specific survival, and overall survival of 88%, 98%, 100%, and 99%, respectively, with very few side effects compared to standard whole-gland therapy [Citation179]. A review of 31 uncontrolled studies concluded that HIFU results in short- to medium-term tumor control in treatment of prostate cancer, with a low complication rate comparable with those of established therapies [Citation184]. Successful use of HIFU mediated tumor ablation has been reported in a high number of explorative studies, among others for tumors of the pancreas [Citation158,Citation185], the breast [Citation186], soft tissue sarcoma [Citation187], pediatric tumors [Citation188,Citation279], liver [Citation189], kidney [Citation189], esophagus [Citation190], glioma [Citation280] and other organs [Citation191,Citation192,Citation281]. A recent pilot study, published in the Lancet Oncology, showed that highly targeted mild hyperthermia (≥39.5 °C for at least 30 min) by ultrasound triggered delivery of doxorubicin to liver tumors that were refractory to standard chemotherapy is feasible, safe and able to enhance intratumoral drug delivery, providing targeted chemoablative response [Citation70].
3.1.3. Electroporation
Equipment
Irreversible Electroporation (IRE) is a relatively new interstitial local tumor ablation technique that creates pores in the tumor cell membrane by using a series of very short (e.g., ∼100 μs), high voltage direct-current electrical pulses (ranging from hundreds to thousands of V/cm) between electrodes implanted around the tumor [Citation282,Citation283]. The irreversible aspect is a result of the number of pores created such that a cell cannot recover from the collapse of cell membrane function. Though presented as non-thermal, inclusion of IRE in this review is justified since significant temperature rises in the hyperthermic and in the thermal ablation range are reported to occur during IRE, significantly contributing to the ablation effect [Citation284–290]. These significant temperature increases are a result of the excessively high SAR generated in a short time (1 – 10 min) in the treatment area during IRE, particularly close to the electrodes. Careful placement of the electrodes is essential to manage these effects and prevent damage to surrounding healthy tissue, critical vascular structures and adjacent organs [Citation291].
Clinical applications of IRE
Clinical IRE is performed using the NanoKnife System of Angiodynamics (Latham, NY) and has been applied for treatment of tumors in lung, liver, kidney, pancreas and prostate, and has been proposed as a safer option than conventional ablation for tumors in close proximity to critical structures such as blood vessels and biliary ducts where thermal ablation would have caused serious complications [Citation193–198]. Patients with persistent locally advanced disease after chemotherapy for whom surgical exploration is not feasible could benefit from this less invasive approach [Citation194]. Note that general anesthesia is required with paralytic agents to prevent undesired muscular contractions, as well as careful synchronization with the electrical activity of the heart to avoid arrhythmias that could arise if the administered pulses stray outside of the refractory period of the cardiac cycle. Recently, high-frequency irreversible electroporation (H-FIRE) technology has been developed to enable electroporation of tumors without stimulation of the nearby skeletal muscle; this would avoid the need for general anesthesia [Citation292]. Treatment efficacy decreases with increasing tumor size, and the procedure becomes more challenging with a large number of electrodes. Therefore, IRE should be limited to a maximum tumor diameter of 5 cm [Citation293]. Phase III trials are ongoing, for example comparing chemotherapy plus stereotactic ablative radiotherapy with chemotherapy plus IRE for locally advanced pancreatic cancer.
3.1.4. Ferromagnetic seeds and nanoparticles
Equipment
Ferromagnetic seeds provide a form of conductive interstitial hyperthermia developed for use in conjunction with brachytherapy. The advantage of using ferromagnetic seeds is the absence of a need for connecting wires since the heating is generated by exposing the patient to an external magnetic field, allowing for remotely controlled tissue heating from within the target [Citation294]. Another advantage is the option to use self-regulating alloys, which heat only to the Curie temperature, i.e., the temperature at which a magnetic phase transition from ferromagnetic to paramagnetic occurs. The Curie temperature depends on the alloy composition and can be set to match the desired therapeutic level [Citation295,Citation296].
Magnetic Fluid Hyperthermia (MFH) uses MNPs for heating the tumor while avoiding toxicity to the surrounding healthy tissue. The quality of the implantation of the nanoparticles in and around the target region, as pre-calculated in a planning system, is important. Nevertheless, by virtue of both variable tumor physical properties and complexities of nanofluid dynamics, nanoparticle distributions in tissue are often inhomogeneous [Citation297], resulting in a heterogeneous temperature distribution. One method to compensate for this is to dynamically adjust the heating by adjusting the magnetic field amplitude [Citation297]. Nanoparticle heat output is non-linear with magnetic field amplitude (i.e., specific loss power ∝ H2 or H3). The heat generated by nanoparticles will transfer away from the heat source(s) by conduction and convection, depending on tissue properties and cooling/heat sink effects of fluid (e.g., blood), respectively. On the other hand, tissue response to heating is also non-linear and dynamic vis a vis thermoregulatory response that changes the heat sink effect, and tissue damage resulting in collapse of perfusion-dependent cooling. Amplitude adjustment exploits these non-linear interactions to explicitly generate intense heat locally for a period of time (high amplitude), followed by a period of reduced heating (low amplitude) to allow time for heat generated to transfer throughout the target tissue. In the ideal scenario, a balance is struck between high/low amplitude cycling that deposits sufficient power to achieve a desired target thermal dose (e.g., CEM43T90 = 60 min). With this technique, the applied power is adjusted based on temperature feedback measured at the tumor-tissue boundary, in combination with a proportional-integral-derivative (PID) control system. Numerical simulations have recently shown that this is a promising strategy to realize a more homogeneous temperature distribution compared with straightforward constant power heating [Citation297].
Clinical applications of ferromagnetic seeds and nanoparticles
Clinical results of ferromagnetic seeds were encouraging; Mack et al. [Citation298] reported minimum and maximum temperatures of 41.0 °C and 43.7 °C and a 93% total response rate with only 7% grade 3 or 4 toxicity in a study of 44 patients with advanced primary or recurrent extra-cranial solid malignancies. However, optimized performance of ferromagnetic seeds is impaired by the limited 3 D temperature control and by the low thermal conductivity of the catheters or coatings, which are needed to implant the seeds or to ensure biocompatibility. This makes it difficult to ensure precision treatment.
Most MNPs are in a preclinical stage of development and clinical experience with MNPs is mainly limited to treating glioblastoma. The brain is a challenging tumor site where other hyperthermia methods are expected to cause major side effects. Results of a single-arm phase 2 study with 59 patients with small (<4 cm) recurrent glioblastoma lesions treated with MNP (median peak tumor temperature 51.2 °C) combined with external beam radiotherapy (median biologically effective dose 30 Gy; 5 × 2 Gy/week) were encouraging with results suggesting a doubling of expected overall survival to 13.2 months, albeit in a favorable patient group [Citation112]. For monitoring tumor progression the use of MRI is excluded due to the very high particle concentrations used, but good alternatives PET and SPECT are available [Citation112]. NanoTherm® AS1 (MagForce Nanotechnologies AG, Berlin), the Iron-Oxide MNP used in this study, was approved for use combined with RT in patients with recurrent glioblastoma in Europe in 2010 and received FDA approval for a trial in prostate cancer patients in the USA.
3.1.5. Intraluminal hyperthermia/HIVEC
Equipment
Intracavitary and intraluminal techniques apply local hyperthermia from within a cavity or lumen, e.g., vagina, esophagus, urethra or bladder. Heating is generally limited to the lumen and its close proximity up to a depth of ≤1 cm. Both radiative and conductive techniques are available for intraluminal hyperthermia combined with chemotherapy for non-muscle invasive bladder cancer (NMIBC) [Citation299]. Hyperthermic Intra-Vesical Chemotherapy (HIVEC®) involves recirculating a heated chemotherapy solution in the bladder using for example the Combat BRS system (Combat Medical, Wheathampstead, UK) [Citation55]. Similar commercially available recirculating systems include the Unithermia system (Elmedical Ltd, Hod-Hasharon, Israel) [Citation199] and the BR-TRG-I hyperthermic perfusion system (Guangzhou Bright Medical Technology Co., Ltd. Guangzhou, China) [Citation200]. Recirculation of heated chemotherapy is a technically straightforward form of conductive heating and will give a fairly uniform temperature rise over the bladder wall provided the flow is sufficiently high. Its maximum penetration depth of ∼0.5 cm in the bladder wall makes it suitable for early stage NMIBC [Citation302]. Deeper tissue penetration up to ∼1 cm is achieved using the Synergo® system (Medical Enterprises, Amsterdam, the Netherlands). This system uses an intracavitary 915 MHz microwave antenna in a Foley catheter, which also includes up to five thermocouples to monitor the bladder wall temperature, and two channels for recirculation of the chemotherapy in the bladder [Citation300,Citation301].
Clinical applications of intraluminal hyperthermia and HIVEC
A recent trial in 161 intermediate and high risk NMIBC patients instilled with pirarubicin demonstrated a 24-month recurrence-free survival of 82.9% in the HIVEC group (n = 76, three 45 min 45 °C HT sessions 48 h apart, 2nd session combined with pirarubicin instillation), versus 63.5% in the non-hyperthermia control group (n = 85, p = 0.008) [Citation71].
A randomized controlled trial compared thermochemotherapy using the Synergo system (heating to 42 ± 2 °C) and chemotherapy alone with Mitomycin C for 83 patients with intermediate-/high-risk NMIBC. Eight weekly 40–60 min treatment sessions were followed by 4-monthly sessions. The 10-year disease-free survival rate was increased from 15% in the Mitomycin C arm to 53% in the Mitomycin C plus hyperthermia arm [Citation72]. Two randomized trials demonstrated that Mitomycin C + Synergo based hyperthermia provides similar or possibly better results compared to standard Bacillus Calmette-Guérin (BCG) treatment for NMIBC patients [Citation73,Citation74]. Disadvantages of this intracavitary heating approach are an uneven SAR distribution over the bladder wall, and that treatment of more deeply penetrating muscle invasive bladder cancer will require the use of a locoregional hyperthermia device [Citation299,Citation302].
3.1.6. Superficial hyperthermia
Equipment
In superficial heating, the energy is deposited in a limited volume of tissue close to the heating device. It is therefore applied only to tumors extending up to ∼3–4 cm below the skin surface [Citation303]. Several heating devices have been developed for both human [Citation304] and veterinary clinical use [Citation305]. Clinically used superficial heating equipment includes external infrared sources, microwave antennas, radiofrequency electrodes or ultrasound transducers [Citation304].
Superficial tumors infiltrating up to 1.5–2 cm can be treated with infrared heating. The Hydrosun system (Hydrosun medizintechnik GmbH) provides contact free heating of large tumor areas. The penetration depth of infrared is normally less than 1 cm, but the Hydrosun applies water filtering of the radiation emitted by a halogen lamp of high color temperature to ensure effective heating up to 1.5–2 cm depth, and to avoid both overheating of the skin and tissue dehydration [Citation209]. The Hydrosun system provides real-time infrared thermography measurement and control of superficial temperatures [Citation209].
For superficial lesions with deeper infiltration, microwave heating is often applied. A water bolus with water circulating at a temperature typically around 40 °C is used to couple the electromagnetic energy into the tissue. The selected exact water temperature influences the skin temperature and depends on the desired heating depth and whether the skin is target or not [Citation306–308]. Many in-house developed prototypes have been introduced over the years and the first microwave systems usually applied a frequency of 2450 MHz [Citation210,Citation309]. However, the penetration depth is relatively low at this high frequency, which means that tumors extending to 4 cm beneath the skin will not receive an adequate hyperthermia dose. Significant progress has been made in further development of systems with better penetration depth at 915 and 434 MHz. Furthermore, superficial antennas with various effective field sizes allowing adequate treatment of both small and larger tumor areas have been developed. This has resulted in clear progress in the quality of superficial heating equipment [Citation310–315].
The 915 MHz BSD-500 system (Pyrexar Medical, Salt Lake City, UT, USA), which delivers both interstitial and superficial hyperthermia, provides three different sizes of rigid waveguides: type MA-100, MA-120, and MA-151, with aperture sizes of 10 × 13 cm2, 18 × 24 cm2 and 4 × 5 cm2, respectively. The effective heating areas are somewhat smaller than the aperture size: 8 × 10 cm2, 12.5 × 19.5 cm2 and 2.5 × 2.5 cm2, respectively [Citation303]. A water bolus couples electromagnetic fields into tissue. Additionally, two multi-element applicators are available with this system: an 8-element rigid array (SA-812) and a 24-element flexible array with spiral antennas (SA-24). The SA-812 applicator consists of a closely spaced array of 8 dual-armed Archimedean spiral antennas with a diameter of ∼3.5 cm, 7 spirals surround a central spiral [Citation316]. This allows adaptation of the heating pattern to irregularly shaped tumors by using appropriate amplitude settings for the individual antennas. The diameter of the applicator is 14 cm and spiral antennas are deposited on a plexiglass substrate with an integral water bolus. The SA-24 applicator consists of 24 dual-armed Archimedean spiral antennas mounted in a 4 × 6 array on a rectangular silicone carrier [Citation317]. The applicator is flexible and can follow the body contour. The spiral antennas are connected in pairs of three to one of the eight RF power amplifiers of the BSD-500 system. These spiral antennas are considerably lighter in weight than waveguide applicators, and easier to position near complex patient anatomies. The heating depth of this 915 MHz system is 2–2.5 cm.
Deeper heating, up to 4 cm, can be achieved with 434 MHz antennas. The Yahta-4 system uses contact flexible microstrip applicator (CFMA; SRPC ‘Istok’, Fryazino, Russia) consisting of two coplanar active electrodes and a shield electrode, separated by a thin fluoroplastic substrate [Citation318]. The applicator has an integrated water bolus and can be bent to follow the curvature of the body contour. Five different sizes are available to cover target regions of various dimensions. The smallest antenna, type 1H, has an aperture size of 7.2 × 19.7 cm2 and the largest, type 3H and 5H, have aperture sizes of 28.7 × 20.7 cm2 and 19.7 × 28.5 cm2, respectively, the difference being a 90° shift in field direction. Bending the applicator increases the effective heating depth [Citation319,Citation320]. The water bolus thickness should not exceed 2 cm in order to avoid resonance effects [Citation321]. The CFMA-SRH applicator has been developed with characteristics similar to the conventional CFMA, but allows simultaneous application of radiation and hyperthermia [Citation322]. Simultaneous application of radiotherapy and hyperthermia yields comparable thermal enhancement in tumor tissue and normal tissue [Citation323], which could lead to normal tissue toxicity. Therefore, tumor selective heating is important and new types of CFMA-SRH have been developed, consisting of an array of independent microstrip heating elements [Citation314]. Four types of applicators are available, consisting of arrays of 1 × 3, 2 × 2, 3 × 3 and 3 × 4 heating elements. The sizes of the individual heating elements in the arrays range from 6 to 7.5 cm.
The ALBA ON 4000 (ALBA Hyperthermia, Rome, Italy) is a 434 MHz system for superficial hyperthermia, which uses antennas similar to the CFMA, but with a fixed curvature. The system has an integrated thermocouple thermometry system for continuous monitoring of 4–64 temperature sensors. The ALBA Double ON 4000 consists of two treatment units that can be used simultaneously. This allows coverage of larger treatment areas by placing two adjacent applicators, as well as treatment of two separate lesions at the same time.
The lucite cone applicator (LCA) is a 434 MHz water-filled horn applicator developed at Erasmus Medical Center [Citation313]. An advantage of the LCA is the large effective field size, which approaches the aperture size and is ∼2.5 times larger than for a conventional waveguide [Citation312]. The aperture size of a single LCA is 10 × 10 cm2 and multiple antennas can be combined in an array configuration to cover the desired treatment area. In clinical practice, the size and rigidity of the LCA may compromise the treatment set-up and an applicator mounting system is required.
Superficial tumors can extend more than 4 cm deep and heating equipment operating in the microwave range does not provide sufficient penetration depth to heat these tumors effectively. Capacitive heating systems can penetrate to greater depths than 4 cm. Commercially available capacitive systems include the Thermotron RF8 (Yamamoto Vinita Co, Osaka, Japan), EHY-2000+ and EHY-2030 (Oncotherm Kft, Budapest, Hungary), Celsius TCS (Celsius42+ GmbH, Cologne, Germany), HY-DEEP 600WM (Andromedic srl, Velletri, Italy) and Synchrotherm (Synchrotherm, Vigevano, Italy). These systems all operate at a frequency of 8 or 13.56 MHz and because of the large wavelength at these frequencies, temperatures and treated volumes are comparable at 8 and 13.56 MHz. The devices use circular electrodes with an integrated water bolus bag and two opposing electrodes are positioned around the target region. The electrodes used clinically vary between 4 cm and 30 cm in diameter. When using two electrodes of differing size, the energy deposition is concentrated under the smallest electrode. Some systems apply added amplitude modulation in the kHz range to induce either additional non-thermal effects or very localized heating of cell structures. However, the latter has been demonstrated to be physically impossible [Citation324], but research on the non-thermal effects is still ongoing, suggesting that the RF field might affect the mitochondrial function in cancer cells [Citation325,Citation326] and change cell topography, morphology, motility, adhesion and division [Citation327]. Capacitive variants of the CFMA and CFMA-SRH have also been developed, operating at 27, 40.68 or 70 MHz [Citation314,Citation318], but clinical use of these antennas is sparse. A clinical limitation of capacitive heating in general may be excessive heating of fat tissue due to the orientation of the main electric field component [Citation28,Citation328].
To realize the greater attainable penetration depth with radiative systems, either lower frequencies and/or multi-applicator arrangements are required. Radiative heating systems that provide sufficient penetration to heat deeply infiltrating superficial tumors are the 70 MHz AMC-2 system [Citation119], the 70 MHz breast applicator developed at the Academic Medical Center Amsterdam [Citation120] and the 140 MHz breast applicator developed at Duke University Medical Center [Citation329]. The AMC-2 system has been developed for treatment of deep-seated advanced breast tumors and supraclavicular tumors [Citation119]. The system consists of two horizontally revolving and height adjustable rectangular 70 MHz waveguides. The dorsal antenna is integrated in the treatment table and has an aperture size of 20 × 34 cm2. To enhance flexibility of this system. the top waveguide is interchangeable and aperture sizes of 8.5 × 34 cm2, 15 × 34 cm2 and 20 × 34 cm2 can be selected, depending on the treatment location and target dimension. Clinical results demonstrate adequate heating [Citation119]. Both breast applicators have been developed for an intact breast. The 70 MHz AMC breast applicator has a treatment bed fitted with a 50 × 40 × 16 cm3 temperature controlled open water bolus [Citation120]. The patient’s breast is immersed in the water and positioned in front of a 70 MHz waveguide in the bottom of the bolus, with aperture size 20 × 34 cm2. Clinical results demonstrate adequate heating, though the system is less comfortable for obese patients [Citation120]. The 140 MHz Duke applicator uses a phased-array of four end-loaded dipole antennas, mounted on a Lexan water tank. Geometric focusing is employed, such that each antenna points in the direction of the target [Citation329].
The HYPERcollar is a dedicated phased-array system for more challenging head and neck tumors and has been developed at the Erasmus Medical Center [Citation330]. The system consists of multiple patch antennas, operating at 434 MHz. First clinical results show that the system is safe and feasible [Citation121] and currently an MR-compatible system is under development [Citation331].
Ultrasound energy has also been used to achieve superficial hyperthermia and there has been a similar evolution in technology as for microwave heating. Early devices applied single transducers operating at 0.5–3.5 MHz and only small superficial lesions could be treated [Citation332]. The Sonotherm 1000 system (Labthermics Technologies Inc, Champaign, Il) provides two sizes of multi-sector applicators, combining multiple transducers in a non-focused planar array [Citation211,Citation333–335]. Each sector can be adapted individually to realize a uniform heating pattern. A 16-sector applicator of 15 × 15 cm2 has been developed to heat larger superficial tumor volumes with a depth up to 8 cm. A smaller 4-sector applicator of 7.5 × 7.5 cm2 allows treatment of anatomical sites difficult to reach with the large applicator, such as the head and neck. The treatment depth can be varied by changing the frequency (1 or 3.4 MHz). The system has a 16 channel thermometry system for thermocouple based thermometry and a therapy control algorithm controls the energy deposition, based on the information of the temperature measurement points.
Clinical applications of superficial heating
Superficial heating is commonly applied to melanoma, head and neck cancer, and chest wall recurrences of breast cancer with good clinical results. The importance of sufficient heating depth to achieve good tumor response was demonstrated for example by Van der Zee et al. [Citation210], who compared 2450 MHz and 434 MHz for treatment of recurrent breast cancer. Re-irradiation (eight fractions of 4 Gy twice weekly) was combined with 60 min hyperthermia. The complete response rate (CR) was 74%, but for tumors with a diameter larger than 3 cm heating at 2450 MHz was less effective (65%) than for smaller tumors (CR 87%). Heating at 434 MHz performed equally well for tumors larger or smaller than 3 cm.
Randomized trials have demonstrated the effectiveness of radiation combined with microwave hyperthermia for recurrent breast cancer [Citation212]. Vernon et al. [Citation122] combined the results of five randomized trials for radiation ± hyperthermia for superficial localized locally advanced and recurrent breast cancer and found an increase in overall CR rate from 41% with radiotherapy alone to 59% for combination with hyperthermia. The greatest effect was observed in patients with recurrent lesions in previously irradiated areas, where the re-irradiation dose was limited. A randomized trial by Jones et al. [Citation213] confirmed this good response and also showed that the effect was even better for previously irradiated patients. For this group the CR was 23.5% for re-irradiation alone versus 68.2% in the re-irradiation plus hyperthermia arm. In a recent study very good results were also reported for water-filtered infrared heating using the Hydrosun system combined with hypofractionated re-irradiation (4 Gy once per week up to a total dose of 20 Gy, within 1–4 min after heating) for heavily pretreated patients with large breast cancer recurrences [Citation209]. A complete response rate of 61% was observed in patients with macroscopic disease with only grade 1 toxicity. This study suggests that even repeated re-irradiation could be considered as a treatment option when combined with hyperthermia.
The value of microwave superficial hyperthermia as an adjuvant to radiotherapy was also demonstrated in a multi-center randomized trial for patients with recurrent or metastatic malignant melanoma [Citation123]. After a combined treatment of radiotherapy (three fractions of 8 or 9 Gy in 8 days) plus 60 min hyperthermia 2-year tumor control was 46%, compared with 28% with radiation alone [Citation124].
Treatment of recurrent breast tumors with infiltration beyond 4 cm is more challenging. Capacitive heating yields sufficient penetration depth and allows therapeutic temperatures to be achieved, but temperature distributions can be very heterogeneous with treatment limiting hot spots [Citation328]. The feasibility of adequate heating of these tumors with fewer hot spots has been demonstrated with the radiative 70 MHz AMC-2 system [Citation119] and with the 70 MHz AMC breast applicator [Citation120].
Capacitive hyperthermia has been demonstrated to be an effective technique for heating locally advanced head and neck tumors. A randomized trial comparing radiotherapy (66–70 Gy in 6.5–7 weeks) with radiotherapy plus weekly hyperthermia for 30 min showed that adding hyperthermia increased the complete response rate from 42.4% to 78.6% [Citation116]. No excessive thermal toxicity was reported in this study.
Clinical results for ultrasound hyperthermia using the Sonotherm 1000 system have been reported for tumors in four sites: groin/trunk, axilla, breast or chest wall, and head and neck [Citation211]. Therapeutic heating levels are feasible and of these categories groin and trunk tumors were most effectively heated. Head and neck tumors proved more difficult to heat with only 8% of the measurement points exceeding 42 °C.
3.2. Locoregional heating techniques
Locoregional hyperthermia aims at heating the tumor target volume plus an additional margin of normal tissue. Locoregional heating techniques include electromagnetic heating, Hyperthermic IntraPeritoneal Chemotherapy (HIPEC) and isolated perfusion. Extending heating to an additional normal tissue margin is effective for pre-heating of blood supply to the tumor or to treat microscopic spread outside the macroscopic tumor.
3.2.1. Electromagnetic locoregional heating
Equipment
Electromagnetic locoregional heating uses external heating devices with antennas positioned around the patient and is typically applied for deep-seated tumors, for example in the pelvic or abdominal region. In contrast to more focused techniques such as HIFU, with locoregional heating the region around the tumor is also heated to some extent and the temperature distribution is largely determined by the individual anatomy. In the pelvis tumor heating is sometimes restricted by hot spots near bony and fatty tissues. Compared with local heating techniques, locoregional heating allows a more homogeneous temperature distribution in the target region to be achieved, since the heated volume is larger, including a large normal tissue margin, and the inflowing blood will be slightly preheated and thus cause less cooling [Citation257]. Mild heating of normal tissue around the tumor will usually not increase normal tissue toxicity when radiotherapy and hyperthermia are applied sequentially with a short time interval [Citation139,Citation336,Citation337]. However, hot spots in normal tissue should be avoided. These are manifested as complaints of pain when the temperature exceeds 45 °C. These hot spots typically occur at tissue interfaces associated with a large contrast in dielectric and thermal tissue properties, e.g., going from muscle to fat or bone, particularly if the E-field is perpendicular to these interfaces. Pace-makers and metallic implants in the treatment region are usually a contra-indication for treatment.
Electromagnetic locoregional heating can be performed using capacitive heating devices or radiative phased-array systems. Commercially available capacitive heating systems have already been discussed in the section ‘Superficial Hyperthermia’. For deep heating of centrally located tumors equally sized electrodes with a diameter ≥ 25 cm are usually used. These double-electrode systems have no power steering potential to avoid hot spots. The choice of bolus and its specific design is important for capacitive heating to reduce heating of the superficial fat layers, which is a serious risk because the main E-Field direction is perpendicular to the superficial fat layers. Overlay boluses can be used for more aggressive skin cooling [Citation338], but achievable temperatures at deep-seated tumor locations decrease for increasing fat layer thickness [Citation29].
Radiative phased array systems consist of multiple antennas organized in one or more rings and allow power steering by adapting phase amplitude settings of the individual antennas or antenna pairs. The dominant E-field direction is parallel to the superficial fat layers, i.e., in cranial-caudal direction. Focusing of the electromagnetic energy on the tumor location, as well as suppression of severe hot spots in normal tissue can be realized by adjusting phase and amplitude settings for power steering to create constructive interference in the tumor and destructive interference at hot spot locations among the electromagnetic fields radiated by the antennas [Citation339–341]. In the applied frequency range, i.e., between 60 and 150 MHz, the heating focus is typically 10–15 cm in diameter, which allows heating of larger tumors compared to other techniques (). Commercially available phased-array systems are the BSD-2000 systems (Pyrexar Medical, Salt Lake City, UT, USA) [Citation340,Citation342] and the ALBA 4 D system (ALBA Hyperthermia, Rome, Italy) [Citation343]. Because of the large number of degrees of freedom of these systems, optimization of antenna settings by treatment planning is becoming increasingly common (see section Treatment planning) and has been validated for both systems [Citation344–347].
The BSD-2000 systems include the Sigma-60 system and the smaller bore Sigma-30, with 8 paired dipoles organized in one ring and the Sigma-Eye system, with 24 paired dipoles distributed over three rings, which allows improved power steering properties. Cross-coupling between antennas in the dipole array can affect phase-amplitude control during clinical applications [Citation348,Citation349], but on-line adjustments can overcome this problem [Citation350]. A hybrid version of the Sigma-30 and Sigma-Eye system has been integrated in suitable MR-scanners to enable noninvasive MR-thermometry. The first prototype of this MR-HT hybrid system was installed and evaluated at Charité Berlin using a Siemens Symphony 1.5 T scanner [Citation351–353]. Other versions employ a 1.5 T Philips Ingenia MR system and a 1.5 T GE MR450w MR system [Citation354]. More details on the status of MR thermography are given in the thermometry section.
The ALBA-4D system consists of four rectangular waveguides organized in one ring and is similar to the AMC-4 system, developed and built by the Academic Medical Center Amsterdam [Citation355]. The AMC-8 system, with 8 rectangular waveguides in two rings, has also been developed and built by the Academic Medical Center Amsterdam [Citation356]. This system has a greater heating length and improved power steering compared with the single ring AMC-4 system.
Clinical applications of electromagnetic locoregional heating
Capacitive locoregional heating has been investigated for several tumor locations, such as lung [Citation78–80,Citation127–129], prostate [Citation130,Citation131], bladder [Citation81,Citation132,Citation201], rectum [Citation133,Citation134] and liver [Citation82,Citation83], and treatments were generally deemed feasible. Degrees of clinical success are usually varying, but good clinical results can be achieved with capacitive hyperthermia, provided that the patient has only a thin superficial fat layer [Citation29]. Increasing temperatures to 38–39 °C have shown a significant increase in tumor blood perfusion for cervical cancer patients [Citation30]. Early results of an ongoing phase III trial using the EHY-2000+ device report improved 6 month local disease control for cervical cancer patients treated with radiochemotherapy combined with capacitive hyperthermia (45.5%) compared to radiochemotherapy alone (24.1%) [Citation84]. A multicenter randomized study in cervical cancer patients showed that cisplatin-based radiochemotherapy plus weekly hyperthermia for 60 min using capacitive heating (Thermotron RF8) resulted in a higher 5-year complete response rate (88%) than with radiochemotherapy alone (77.6%) [Citation85]. Clinical outcome appeared to depend on the thermal dose achieved; a successive analysis showed that a thermal dose of at least one cumulative equivalent minute at 43 °C (CEM43T90 ≥ 1) is required for a significant effect of hyperthermia [Citation31]. A phase III prospective, randomized controlled trial demonstrated the effectiveness of capacitive hyperthermia in patients with painful bony metastases [Citation135]. Radiotherapy (10x3Gy) plus twice weekly hyperthermia for 40 min showed a significant increase in pain control rate and an extended response duration compared to radiotherapy alone. The accumulated complete response rate within 3 months after treatment was 58.6% in the radiotherapy + hyperthermia group versus 32.1% in the radiotherapy-alone group [Citation135].
Excellent clinical results have been achieved with radiative locoregional heating of deep-seated tumors in the pelvis, including cervix, bladder, rectum, sarcoma and pediatric tumors. The Dutch deep hyperthermia trial showed the beneficial effect of combining standard radiotherapy with 60 min weekly hyperthermia for pelvic tumors (cervix, rectum, bladder) [Citation139]. The treatment effect did not differ significantly with tumor site, but adding hyperthermia seemed to be most effective for cervical cancer, for which 3-year overall survival was 27% in the radiotherapy group and 51% in the radiotherapy plus hyperthermia group [Citation139]. After 12 years the survival of the patients in the radiotherapy plus hyperthermia group was still twice as high as survival in the radiotherapy-arm (37% vs 20%) [Citation208]. A randomized phase III trial for soft-tissue sarcoma demonstrated that adding 60 min hyperthermia to chemotherapy (etoposide, ifosfamide and doxorubicin) on day 1 and 4 for each cycle significantly improves long-term outcome, with a 10-year survival of 52.6% for chemotherapy plus hyperthermia versus 42.7% for chemotherapy alone [Citation89]. The combination of hyperthermia plus chemotherapy is also very important in treatment of pediatric sarcoma or germ cell tumors that respond poorly to chemotherapy, or recur after chemotherapy [Citation90–92]. A prospective study reported a 5-year survival of 72% and the long-term prognosis was almost similar to those for patients receiving first-line chemotherapy treatment [Citation90]. Ongoing clinical trials evaluate the addition of radiative locoregional hyperthermia to chemotherapy in treatment of e.g., pancreatic cancer [Citation93], or to chemoradiation in treatment of e.g., rectum, pancreatic and anal cancer [Citation357–359].
3.2.2. Hipec
Equipment
Hyperthermic IntraPeritoneal Chemotherapy (HIPEC) is a procedure during which a solution containing chemotherapeutic drugs is maintained at an elevated temperature level (41–43 °C) and circulated in the peritoneal cavity (abdomen) for a period of 30–90 min, aiming at eradication of microscopic tumor lesions of peritoneal carcinomatosis (PC). As drug penetration is limited to a few millimeters [Citation360], HIPEC is generally preceded by cytoreductive surgery (CRS), surgical removal of macroscopic tumor [Citation53]. Commercially available HIPEC devices consist of a peristaltic or roller pump, a temperature controlled container for the chemotherapy, tubing to transport chemotherapy to and from the patient and temperature probes to be used in the peritoneal cavity. Systems used include the ThermoChemTM HT-1000 and HT-2000 (ThermaSolutions, White Bear Lake, MN, USA), Performer (RAND Biotech, Medolla, Italy) and other devices. The chemoperfusion technique is either ‘closed’ (with temporary skin closure during chemoperfusion) or ‘open’ (the coliseum technique, referring to the skin being pulled upward around the open peritoneal cavity) during HIPEC [Citation53]. Both approaches have specific advantages in terms of flow and temperature management during HIPEC.
Clinical applications of HIPEC
Clinical application of HIPEC has gained much popularity in the last decade [Citation222–226,Citation361,Citation362], with promising results in stage III ovarian cancer and in gastric cancer with peritoneal metastases. A phase III study comparing cytoreductive surgery with surgery plus 90 min HIPEC with cisplatin at 40 °C for ovarian cancer reported that HIPEC increased the median overall survival from 33.9 months to 45.7 months after a median follow-up of 4.7 years, with no significant difference in side-effects [Citation102]. In gastric cancer with peritoneal metastases, a recent retrospective multicenter comparison with propensity score based matching of CRS alone versus CRS combined with HIPEC showed that the addition of HIPEC improved overall survival from 12 to 19 months (hazard ratio 0.42–0.86; p = 0.005) [Citation103]. In colorectal cancer with peritoneal metastases (PM), the place of HIPEC remains uncertain. A Dutch randomized trial comparing IV chemotherapy alone (only fluorouracil with leucovorin) with CRS and 90 min mitomycin C-based HIPEC at 41–42 °C inflow temperature showed that the median survival doubled from 12.6 months with standard therapy to 22.3 months with CRS and HIPEC, after a median follow up of 21.6 months [Citation104]. However, recent randomized trials of surgery alone versus surgery combined with oxaliplatin based HIPEC for 30 min at 42 °C failed to show efficacy of the addition of HIPEC, either in the treatment or in the prevention of PM [Citation105,Citation363,Citation364]. As a result, the value of HIPEC for colorectal cancer regarding optimal choice of drug, HIPEC temperature and treatment duration are under debate [Citation365], even including the role of the raised temperature [Citation366].
3.2.3. Isolated perfusion
Equipment
Prophylactic hyperthermic isolated limb perfusion (ILP) is applied for treatment of localized high-risk melanoma and soft tissue sarcoma in extremities [Citation106,Citation107]. ILP involves placement of intraluminal vascular catheters in the inflow and outflow vessels of the extremity, which are then connected to an extracorporeal flow circuit to circulate heated chemotherapeutics for 60–90 min. An upstream tourniquet compresses collateral vessels to fully isolate the extremity. An advantage of ILP is the ability to use higher drug doses, thus locally enhancing efficacy of chemotherapy in the limb while avoiding systemic side effects. By using the vascular system for heat delivery, the temperature rise is inherently uniform. All the equipment required consists of standard equipment already in use for standard surgical care (tourniquet, catheters, heart-lung machine).
Clinical applications of isolated perfusion
This very effective treatment approach may avoid the need for amputations. A multicenter non-randomized trial combining tumor necrosis factor (TNF) with 60–90 min melphalan-based ILP for treatment of locally advanced soft tissue sarcomas in extremities reported response rates above 70% and limb salvage rates above 80% [Citation107]. For ILP mild temperatures of 38–39 °C are essential to obtain a good response without damage to the normal tissues in the limb. Higher temperatures (42–43 °C) increase the response rate, but are also associated with very severe normal tissue toxicity [Citation107].
3.3. Whole body hyperthermia
Equipment
During whole body hyperthermia (WBH) the body core temperature is increased to 39–40 °C for 6 h (fever range WBH) [Citation367] or to 41–42 °C for 60 min (extreme WBH) [Citation35,Citation368]. WBH is usually applied in combination with systemic therapy, for treatment of metastatic disease. Insulation of the body is required to avoid heat loss. During extreme WBH the patient is under sedation or even general anesthesia. Several systems have been developed to apply WBH using radiant heat. Current commercially available WBH systems use infrared radiation and include the Iratherm1000 (Von Ardenne GmbH, Dresden, Germany) and the Heckel HT3000 (Hydrosun Medizintechik GmbH, Müllheim, Germany) systems. Both devices use water-filtered infrared A and are capable of providing a safe, well controlled and fairly homogeneous temperature elevation [Citation368]. Extra-corporal perfusion and convective WBH techniques have also been applied, but these tended to be associated with higher levels of toxicity and have been abandoned in favor of radiative WBH techniques [Citation368].
Clinical applications of whole body hyperthermia
Phase I/II clinical trials have been performed for e.g., ovarian cancer, colorectal adenocarcinoma, lung cancer and sarcoma and studies report serious grade 3/4 toxicity, of which myelosuppression is most common [Citation35]. Absence of phase III trial results makes the additive value of WBH as part of palliative or curative care unclear. Less demanding treatment options are usually preferred. Ongoing research is investigating the potential benefit of WBH in combination with immunotherapy [Citation13] as well as for nonmalignant diseases [Citation369].
4. Treatment control
Treatment control is important for ensuring high quality hyperthermia treatments. For all non-conductive hyperthermia techniques the steady state tumor temperature distribution achieved during hyperthermia depends on the balance between heat generated in the tissue by the hyperthermia device, and heat removed by thermal conduction, blood flow in discrete large vessels and perfusion in small vessels. Heat generation and removal are both difficult to predict accurately due to uncertainty in the dynamic and patient dependent tissue and perfusion properties. At hyperthermic temperatures, perfusion is significantly increased in the heated region as a result of physiological temperature regulation mechanisms in the body. The magnitude of this increase is dynamic and on forehand unknown and depends on the local temperature and other factors [Citation370]. Therefore temperature feedback combined with treatment planning is needed to generate the desired temperature distribution. In this section we briefly discuss the role of thermometry and treatment planning for achieving treatment control for the devices presented in this review.
4.1. Thermometry
Adequate temperature control is crucial in clinical hyperthermia in view of the strong dose–effect relationship of hyperthermia both for achieving optimal therapeutic gain [Citation3,Citation124,Citation371] as well as for avoiding side effects in normal tissue [Citation372,Citation373]. Thermometry is performed to this end with either single or multi-sensor probes in or near the target volume, or noninvasively by external thermometry methods. Invasive temperature probes provide the gold standard (except with ultrasound where viscous artifacts give unpredictable results) and are based on different measurement principles, including thermocouples, thermistors and fiber-optic sensors. A method is selected based on its appropriateness, including compatibility with the hyperthermia methods, used as outlined in reviews and guidelines [Citation374,Citation375]. The metal leads of thermocouple probes require attention as they may cause local interference and erroneous read-outs when combined with EM and US based hyperthermia devices [Citation376–378]. When thermocouple thermometry is combined with MR thermometry the metal leads may cause field inhomogeneities resulting in critical MR signal voids. Fiber-optic probes are generally insensitive to electromagnetic interference, but are mechanically fragile and can be disturbed when used during US or laser-based hyperthermia. A major limitation of probes is their invasive nature, which is not always feasible.
Noninvasive thermal monitoring would be ideal, and for very superficial skin tumors real-time noninvasive infrared thermography can provide 2D temperature maps. This thermometry method measures the infrared energy of the patient and converts this to temperature. Infrared thermometry is standardly used in combination with the contact-free infrared hyperthermia treatment using the Hydrosun system (Hydrosun medizintechnik GmbH) [Citation209]. For 3 D thermal monitoring, CT, MR and US based noninvasive thermometry methods have been developed [Citation375,Citation379–381], but presently these do not provide the same accuracy as thermometry probes. A major drawback of CT thermometry is the ionizing radiation issue, which makes that MR and US-based methods are generally preferred. When applying MR-thermometry, proton resonance frequency shift (PRFS) methods are preferred because of the excellent linearity and temperature dependence of the PRF [Citation379]. Qualitative US ablation monitoring, which targets tissue coagulation and bubble formation is feasible and robust US thermometry exists for temperatures above 50 °C [Citation375]. Several ultrasound acquisition strategies are employed in ultrasound thermometry and ablation monitoring. For example, changes in stiffness monitored with US shear wave imaging are indicative of cellular necrosis [Citation382].
Noninvasive thermometry is less challenging and often clinically used for thermal ablation monitoring, where a relatively low temperature resolution suffices to detect a temperature rise as this rise is much higher (10 – 40 °C) than for hyperthermia devices (1 – 6 °C). Therefore, applications for mild hyperthermia are still limited and particularly preferred for tumor sites where placement of invasive probes bears significant clinical risks, bearing in mind the pitfalls of the presently available MR or US based noninvasive thermometry methods when used in the mild hyperthermia temperature range. Although US methods have made significant progress for thermometry in this range [Citation375], noninvasive PRFS-based MR-thermometry is most frequently used [Citation383]. This method can provide reference-based temperature maps in near-real time. Under favorable, low blood flow conditions an accuracy of ∼1 °C can be achieved, but the method is sensitive to field drifts and susceptibilities and significant artifacts can occur due to movement, e.g., in the presence of high blood flow, breathing, cardiac and bowel motion, limiting the number of appropriate tumor locations [Citation375,Citation379,Citation384]. These issues all need to be compensated for, for accurate temperature mapping. MR-guided hyperthermia works well for large fixated tumors (especially soft tissue sarcoma) [Citation385]. Since MR-thermometry requires a tradeoff between spatial and temporal resolution, acquisition is often limited to representative tissue slices to reduce acquisition times and allow online control. Practical methods for on-line optimization of the temperature distribution achieved with locoregional hyperthermia with MR-thermometry feedback are still under development [Citation350].
For scanned focused ultrasound treatments, US guidance offers fast imaging with the benefit of immediately revealing obstacles in the acoustic beam path, while MR provides high contrast soft tissue images suitable for planning. Furthermore, since MR allows the acquisition of near-real time temperature information, this can be used to establish a feedback system to the ultrasound driving electronics [Citation386,Citation387]. The closed-loop feedback can be used either to achieve or to maintain a given target temperature or to deliver a predefined thermal dose. This provides better lesion control as it can compensate real-time for local differences in ultrasound absorption or heat loss due to perfusion. Recently, a model predictive control algorithm in combination with MR temperature mapping was introduced for a voxel-based heating strategy that allows compensation for local heat losses e.g., due to perfusion, with a high spatial resolution [Citation39]. MR thermometry finds now routine use in all MR-HIFU applications [Citation388,Citation389].
Based on these useful clinical applications, heating devices such as HIFU or RF antennas have been integrated into MR scanners to allow temperature mapping [Citation350,Citation390]. Commercially available MR guided devices which incorporate MR-thermometry sequences include the BSD-2000 Sigma-Eye which can be integrated into the MR systems of various vendors (see section ‘Locoregional heating techniques’), as well as the Sonalleve HIFU system integrated into the 3 T Achieva MR system (Philips) and the ExAblate FUS system integrated into the Discovery MR450w 1.5 T wide bore and Discovery MR750w 3.0 T wide bore MR systems (GE). US guidance is clinically used in combination with ablative SFUS devices; the Sonablate [Citation391] and Ablatherm [Citation392] systems, where feedback is based on changes in echogenicity rather than temperature mapping [Citation393] (see also section ‘Local heating techniques: scanned focused ultrasound’).
4.2. Treatment planning
Treatment planning simulates SAR distributions and/or temperature patterns in the patient to visualize the effect of different treatment strategies [Citation394]. This can be very helpful in assisting in treatment control. The prediction of SAR and temperature distributions is clinically helpful, even though well-known dependencies on patient models and tissue properties remain. Therefore, quantitatively reliable prospective treatment planning of SAR distributions is challenging and quantitative prediction of temperature is even more challenging because of the uncertainties in tissue properties, and a lack a priori in knowledge of the dynamic changes in perfusion levels which occur during heating [Citation394–397].
Treatment planning is therefore still a research tool for most heating techniques, or is under development for relatively new techniques such as MNP, IRE and HIPEC [Citation398–402]. For thermal ablation, treatment planning can be especially helpful to predict the size of the ablation zone when the target region is located close to critical structures or large cooling blood vessels which can affect the ablation zone [Citation403]. Recent research focused on 3 D planning of image-guided RFA by means of interactive access path determination based on optimization [Citation404]. A first retrospective study indicated that this approach has the potential to improve the classical RFA planning based on inspection of 2 D images alone [Citation404]. Software-guided RFA of primary and secondary liver tumors is currently evaluated in the ClinicIMPPACT study; a multicenter-, prospective-, non-randomized clinical trial to evaluate the accuracy and efficiency of innovative planning and simulation software [Citation405]. MWA has the potential of creating larger ablation zones compared to RFA, but at the same time this yields a greater risk of thermal damage in surrounding healthy tissues, which explains the research interest in development of personalized MWA treatment planning [Citation406]. However, the ultimate planning of MWA is still based on clinical experience of the physician. Characterization of dielectric and thermal property changes and tissue shrinkage is essential for the development of numerical predictive tools.
An additional challenge for reliable hyperthermia treatment planning is the fact that dielectric tissue properties change significantly during treatment in the ablative temperature range [Citation407]. To improve the robustness of treatment planning predictions for ablation, application of probabilistic modeling techniques is presently investigated [Citation408]. For most anatomical locations (with exception of the brain) treatment planning for ultrasound is very challenging since full-wave 3 D simulations are extremely computationally intensive, and thus it is mostly used to optimize or adapt the aperture of US phased arrays to the tumor location or shape [Citation409,Citation410]. Treatment planning for HIFU has to some extent become obsolete because of the use of on-line MR-thermometry for treatment guidance.
Significant progress has been made in the development of treatment planning for classical electromagnetic superficial and locoregional hyperthermia, where the integration into the clinical workflow is becoming common practice [Citation394]. Treatment planning can be very useful for applicator selection to determine a treatment strategy [Citation27,Citation411] or to evaluate potential for heating [Citation345,Citation412]. Despite the limited quantitative reliability, treatment planning can help to optimize heating patterns for locoregional phased array systems. A cross-over trial comparing treatment planning-guided steering and steering with empirical steering guidelines demonstrated that steering guided by treatment planning is feasible [Citation413]. Since measured and simulated changes in SAR and temperature resulting from phase and amplitude adjustments during hyperthermia treatments correlate [Citation414,Citation415], adaptive treatment planning was further evaluated to be used on-line to determine alternative antenna settings that suppress hot spots, or to improve tumor heating during treatment. The practical use of treatment planning to improve treatment control has been demonstrated during clinical hyperthermia treatments [Citation34,Citation385,Citation416,Citation417]. Furthermore, ongoing research aims to quantify the radiosensitizing effect of hyperthermia in terms of equivalent radiation dose, i.e., the radiation dose yielding the same biological effect as the combination of radiotherapy and hyperthermia. Results from biological modeling applied to prostate cancer [Citation418], cervical cancer [Citation419], and recurrent pediatric sarcoma [Citation420] patients demonstrated substantial dose escalations of typically 10 Gy by adding hyperthermia. Biological modeling can also be useful to answer clinically relevant research questions such as the optimal timing between radiotherapy and hyperthermia [Citation337]. Thermoradiotherapy planning is feasible and important to optimize hyperthermia combined with radiotherapy in individual patients [Citation421].
5. Future perspectives
As demonstrated in this review, a large number of hyperthermia and thermal ablation devices have been developed, which are routinely and successfully used for a variety of tumor indications. Progress has resulted in many dedicated devices designed to meet specific heating requirements for specific tumor sites. The hyperthermia field has progressed from single source hyperthermia applicators to sophisticated multiple source instruments. Successful clinical trials have been conducted which combined hyperthermia with radiotherapy or chemotherapy. In some cases, the hyperthermia devices possessed limited spatial control of the SAR distribution. Nevertheless, results demonstrated that the degree of control was sufficient for the tumor sites involved, which included bladder, superficial tumors and deep-seated pelvic tumors. Unmet clinical needs are progressing toward more challenging tumor sites such as brain, deep-seated head and neck tumors and peritoneal metastases [Citation94,Citation422–424]. As a result, there is a trend toward device designs providing greater spatial control of the energy deposition to ensure that SAR and temperature distributions in tissues reliably match with rigorous treatment plans to achieve effective treatment of challenging tumor sites, while sparing adjacent critical structures. Real-time 3-D temperature feedback data combined with reliable treatment planning to guide the applicator settings is also required to achieve the desired tumor target temperature distribution. Examples of this trend include development of dedicated MR guided hyperthermia applicators for brain tumors [Citation422] and H&N tumors [Citation121,Citation330,Citation331] based on MW technology, as well as US or MR guided HIFU devices, both for hyperthermia and for thermal ablation. A design challenge for most of these devices is matching the technical and clinical performance of the device with the oncological requirements for the specific site, e.g., the target volume size for gastrointestinal tumors and the need to spare surrounding normal tissue for brain tumors. For treatment planning, the use of validated and robust algorithms is important, which could possibly be standardized by defining established modeling and validation guidelines for development of software tools.
As shown in , another clear trend over the past two decades is the steadily growing interest in almost all hyperthermic applications, but especially applications of thermal ablation techniques and the more recent rise of HIPEC. This trend is also evident in the increasing clinical application of specific forms of thermal ablation and hyperthermia as shown in . Thermal ablation is now more popular than application of hyperthermia as radiosensitizer or chemosensitizer, and the rapid rise of HIPEC and hyperthermic chemotherapy for NMIBC testify that combination of hyperthermia with chemotherapy is growing faster than combination of hyperthermia with radiotherapy, which is also associated with a different focus in device development. A breakthrough in heat-triggered tumor targeted drug delivery is one trend that would further strengthen popularity of the combination with chemotherapy [Citation70,Citation268,Citation274–277]. Finally, the recent trend to combine thermal therapies with immunotherapies has demonstrated significant potential to address recurrence and metastasis in preclinical and clinical pilot studies [Citation425–428]. When this is supported by further clinical evidence, a further growing interest in hyperthermic applications can be expected.
Figure 5. Trends in heating technologies, represented by a rough estimate of the number of publications from 1995 till present on whole body hyperthermia (WBH), hyperthermia combined with radiotherapy (HT + RT), hyperthermia combined with chemotherapy (HT + CHT), hyperthermic intraperitoneal chemotherapy (HIPEC) and thermal ablation. Search terms used in Web of Science were: (WBH OR whole body hyperthermia; (hyperthermia AND radiotherapy) OR thermoradiotherapy; (hyperthermia AND chemotherapy) OR thermochemotherapy; HIPEC OR Hyperthermic Intraperitoneal Chemotherapy; thermal ablation OR RFA OR MWA). NB: Although this search did probably not retrieve all relevant publications and some overlap is likely between topics (e.g., HT + CHT and HIPEC), this graph is indicative for the trends in interest for different heating techniques.
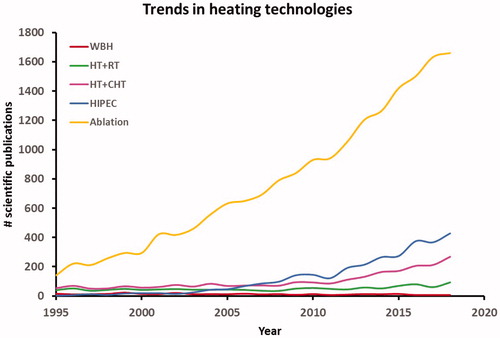
Disclosure statement
Robert Ivkov is an inventor on nanoparticle patents. All patents are assigned to either The Johns Hopkins University or Aduro BioTech, Inc. Robert Ivkov consults for, and is a member of the Scientific Advisory Board for Imagion Biosystems, a company developing imaging with magnetic iron oxide nanoparticles. Robert Ivkov also consults for Magnetic Insight, a company developing magnetic particle imaging. All other authors report no conflicts of interest. The contents of this paper are solely the responsibility of the authors.
Additional information
Funding
References
- Coley WB. The treatment of malignant tumors by repeated inoculations of erysipelas. With a report of ten original cases. Am J Med Sci. 1893;105:487–511.
- Rau B, Wust P, Tilly W, et al. Preoperative radiochemotherapy in locally advanced or recurrent rectal cancer: regional radiofrequency hyperthermia correlates with clinical parameters. Int J Radiat Oncol Biol Phys. 2000;48:381–391.
- Kroesen M, Mulder HT, Van Holthe JML, Aangeenbrug AA, et al. Confirmation of thermal dose as a predictor of local control in cervical carcinoma patients treated with state-of-the-art radiation therapy and hyperthermia. Radiother Oncol. 2019;140:150–158.
- Bakker A, Van der Zee J, van Tienhoven G, et al. Temperature and thermal dose during radiotherapy and hyperthermia for recurrent breast cancer are related to clinical outcome and thermal toxicity: a systematic review. Int J Hyperthermia. 2019;36:1024–1039.
- van Rhoon GC. Is CEM43 still a relevant thermal dose parameter for hyperthermia treatment monitoring? Int J Hyperthermia. 2016;32:50–62.
- Sapareto SA, Dewey WC. Thermal dose determination in cancer therapy. Int J Radiat Oncol Biol Phys. 1984;10:787–800.
- Dewey WC, Hopwood LE, Sapareto SA, et al. Cellular responses to combinations of hyperthermia and radiation. Radiology. 1977;123:463–474.
- Krawczyk PM, Eppink B, Essers J, et al. Mild hyperthermia inhibits homologous recombination, induces BRCA2 degradation, and sensitizes cancer cells to poly (ADP-ribose) polymerase-1 inhibition. Proc Natl Acad Sci U S A. 2011;108:9851–9856.
- Kampinga HH, Dikomey E. Hyperthermic radiosensitization: mode of action and clinical relevance. Int J Radiat Biol. 2001;77:399–408.
- Jones EL, Prosnitz LR, Dewhirst MW, et al. Thermochemoradiotherapy improves oxygenation in locally advanced breast cancer. Clin Cancer Res. 2004;10:4287–4293.
- Vujaskovic Z, Song CW. Physiological mechanisms underlying heat-induced radiosensitization. Int J Hyperthermia. 2004;20:163–174.
- Issels RD. Hyperthermia adds to chemotherapy. Eur J Cancer. 2008;44:2546–2554.
- Bull JMC. A review of immune therapy in cancer and a question: can thermal therapy increase tumor response? Int J Hyperthermia. 2018;34:840–852.
- Dewhirst MW, Vujaskovic Z, Jones E, et al. Re-setting the biologic rationale for thermal therapy. Int J Hyperthermia. 2005;21:779–790.
- van den Tempel N, Laffeber C, Odijk H, et al. The effect of thermal dose on hyperthermia-mediated inhibition of DNA repair through homologous recombination. Oncotarget. 2017;8:44593–44604.
- Prakash P, Diederich CJ. Considerations for theoretical modelling of thermal ablation with catheter-based ultrasonic sources: implications for treatment planning, monitoring and control. Int J Hyperthermia. 2012;28:69–86.
- Calderwood SK, Theriault JR, Gong J. How is the immune response affected by hyperthermia and heat shock proteins? Int J Hyperthermia. 2005;21:713–716.
- Skitzki JJ, Repasky EA, Evans SS. Hyperthermia as an immunotherapy strategy for cancer. Curr Opin Investig Drugs. 2009;10:550–558.
- Chu KF, Dupuy DE. Thermal ablation of tumours: biological mechanisms and advances in therapy. Nat Rev Cancer. 2014;14:199–208.
- Dewhirst MW, Viglianti BL, Lora-Michiels M, et al. Basic principles of thermal dosimetry and thermal thresholds for tissue damage from hyperthermia. Int J Hyperthermia. 2003;19:267–294.
- Cihoric N, Tsikkinis A, van Rhoon G, et al. Hyperthermia-related clinical trials on cancer treatment within the ClinicalTrials.gov registry. Int J Hyperthermia. 2015;31:609–614.
- Stauffer PR. Evolving technology for thermal therapy of cancer. Int J Hyperthermia. 2005;21:731–744.
- Foster KR, Schwan HP. Dielectric properties of tissues and biological materials: a critical review. Crit Rev Biomed Eng. 1989;17:25–104.
- Wust P, Seebass M, Nadobny J, et al. Electromagnetic deep heating technology. In: Seegenschmiedt MH, Fessenden P, Vernon CC, editors. Thermoradiotherapy and thermochemotherapy. Volume 1, Biology, physiology and physics. Berlin: Springer Verlag; 1995. p. 219–251.
- Griffiths DJ. Introduction to electrodynamics. 2nd ed. Englewood Cliffs (NJ): Prentice Hall International; 1989.
- Gabriel S, Lau RW, Gabriel C. The dielectric properties of biological tissues: II. Measurements in the frequency range 10 Hz to 20 GHz. Phys Med Biol. 1996;41:2251–2269.
- Kok HP, Navarro F, Strigari L, et al. Locoregional hyperthermia of deep-seated tumours applied with capacitive and radiative systems: a simulation study. Int J Hyperthermia. 2018;34:714–730.
- Kok HP, Crezee J. A comparison of the heating characteristics of capacitive and radiative superficial hyperthermia. Int J Hyperthermia. 2017;33:378–386.
- Hiraoka M, Jo S, Akuta K, et al. Radiofrequency capacitive hyperthermia for deep-seated tumors. I. Studies on thermometry. Cancer. 1987;60:121–127.
- Lee SY, Kim JH, Han YH, et al. The effect of modulated electro-hyperthermia on temperature and blood flow in human cervical carcinoma. Int J Hyperthermia. 2018;34:953–960.
- Ohguri T, Harima Y, Imada H, et al. Relationships between thermal dose parameters and the efficacy of definitive chemoradiotherapy plus regional hyperthermia in the treatment of locally advanced cervical cancer: data from a multicentre randomised clinical trial. Int J Hyperthermia. 2018;34:461–468.
- Ho JC, Nguyen L, Law JJ, et al. Non-invasive radiofrequency field treatment to produce hepatic hyperthermia: efficacy and safety in swine. IEEE J Transl Eng Health Med. 2017;5:1500109.
- Vrba J, Franconi C, Lapes M. Theoretical limits for the penetration depth of intracavitary applicators. Int J Hyperthermia. 1996;12:737–742.
- Kok HP, Korshuize - van Straten L, Bakker A, et al. Online adaptive hyperthermia treatment planning during locoregional heating to suppress treatment-limiting hot spots. Int J Radiat Oncol Biol Phys. 2017;99:1039–1047.
- Lassche G, Crezee J, Van Herpen CML. Whole-body hyperthermia in combination with systemic therapy in advanced solid malignancies. Crit Rev Oncol Hematol. 2019;139:67–74.
- Missios S, Bekelis K, Barnett GH. Renaissance of laser interstitial thermal ablation. Neurosurg Focus. 2015;38:E13.
- Diederich CJ, Hynynen K. Ultrasound technology for hyperthermia. Ultrasound Med Biol. 1999;25:871–887.
- Zhu L, Altman MB, Laszlo A, et al. Ultrasound hyperthermia technology for radiosensitization. Ultrasound Med Biol. 2019;45:1025–1043.
- Sebeke L, Deenen DA, Maljaars E, et al. Model predictive control for MR-HIFU-mediated, uniform hyperthermia. Int J Hyperthermia. 2019;36:1040–1050.
- Salomir R, Vimeux FC, de Zwart JA, et al. Hyperthermia by MR-guided focused ultrasound: accurate temperature control based on fast MRI and a physical model of local energy deposition and heat conduction. Magn Reson Med. 2000;43:342–347.
- Quesson B, Vimeux F, Salomir R, et al. Automatic control of hyperthermic therapy based on real-time Fourier analysis of MR temperature maps. Magn Reson Med. 2002;47:1065–1072.
- Tillander M, Hokland S, Koskela J, et al. High intensity focused ultrasound induced in vivo large volume hyperthermia under 3D MRI temperature control. Med Phys. 2016;43:1539–1549.
- Coussios CC, Farny CH, Haar GT, et al. Role of acoustic cavitation in the delivery and monitoring of cancer treatment by high-intensity focused ultrasound (HIFU). Int J Hyperthermia. 2007;23:105–120.
- Ebbini ES, ter Haar G. Ultrasound-guided therapeutic focused ultrasound: current status and future directions. Int J Hyperthermia. 2015;31:77–89.
- Ter Haar G. HIFU tissue ablation: concept and devices. Adv Exp Med Biol. 2016;880:3–20.
- Bing C, Ladouceur-Wodzak M, Wanner CR, et al. Trans-cranial opening of the blood-brain barrier in targeted regions using a stereotaxic brain atlas and focused ultrasound energy. J Ther Ultrasound. 2014;2:13.
- Kwan JJ, Myers R, Coviello CM, Graham SM, et al. Ultrasound-propelled nanocups for drug delivery. Small. 2015;11:5305–5314.
- Treat LH, McDannold N, Zhang Y, et al. Improved anti-tumor effect of liposomal doxorubicin after targeted blood-brain barrier disruption by MRI-guided focused ultrasound in rat glioma. Ultrasound Med Biol. 2012;38:1716–1725.
- Rahim AA, Taylor SL, Bush NL, et al. Spatial and acoustic pressure dependence of microbubble-mediated gene delivery targeted using focused ultrasound. J Gene Med. 2006;8:1347–1357.
- Kennedy JE, Wu F, ter Haar GR, et al. High-intensity focused ultrasound for the treatment of liver tumours. Ultrasonics. 2004;42:931–935.
- Rodrigues DB, Stauffer PR, Vrba D, et al. Focused ultrasound for treatment of bone tumours. Int J Hyperthermia. 2015;31:260–271.
- ten Eikelder HM, Bosnacki D, Elevelt A, et al. Modelling the temperature evolution of bone under high intensity focused ultrasound. Phys Med Biol. 2016;61:1810–1828.
- Helderman R, Loke DR, Kok HP, et al. Variation in clinical application of hyperthermic intraperitoneal chemotherapy: a review. Cancers (Basel). 2019;11:78.
- de Bree E, van Ruth S, Baas P, et al. Cytoreductive surgery and intraoperative hyperthermic intrathoracic chemotherapy in patients with malignant pleural mesothelioma or pleural metastases of thymoma. Chest. 2002;121:480–487.
- Sousa A, Pineiro I, Rodriguez S, et al. Recirculant hyperthermic IntraVEsical chemotherapy (HIVEC) in intermediate-high-risk non-muscle-invasive bladder cancer. Int J Hyperthermia. 2016;32:374–380.
- Westermark F. Uber die behandlung des ulzerierenden cervixkarzinoms mittels konstanter warme. Zentralbl Gynakol. 1898;22:1335–1339.
- van Wieringen N, van Dijk JD, Nieuwenhuys GJ, et al. Power absorption and temperature control of multi-filament palladium-nickel thermoseeds for interstitial hyperthermia. Phys Med Biol. 1996;41:2367–2380.
- Gilchrist RK, Medal R, Shorey WD, et al. Selective inductive heating of lymph nodes. Ann Surg. 1957;146:596–606.
- Wust P, Gneveckow U, Johannsen M, et al. Magnetic nanoparticles for interstitial thermotherapy-feasibility, tolerance and achieved temperatures. Int J Hyperthermia. 2006;22:673–685.
- Lanier OL, Korotych OI, Monsalve AG, et al. Evaluation of magnetic nanoparticles for magnetic fluid hyperthermia. Int J Hyperthermia. 2019;36:687–701.
- Jordan A, Wust P, Fahling H, et al. Inductive heating of ferrimagnetic particles and magnetic fluids: physical evaluation of their potential for hyperthermia. Int J Hyperthermia. 1993;9:51–68.
- Eggeman AS, Majetich SA, Farrell D, et al. Size and concentration effects on high frequency hysteresis of iron oxide nanoparticles. IEEE Trans Magn. 2007;43:2451–2453.
- Dennis CL, Ivkov R. Physics of heat generation using magnetic nanoparticles for hyperthermia. Int J Hyperthermia. 2013;29:715–729.
- Dennis CL, Krycka KL, Borchers JA, et al. Internal magnetic structure of nanoparticles dominates time-dependent relaxation processes in a magnetic field. Adv Funct Mater. 2015;25:4300–4311.
- Mahmoudi K, Bouras A, Bozec D, et al. Magnetic hyperthermia therapy for the treatment of glioblastoma: a review of the therapy's history, efficacy and application in humans. Int J Hyperthermia. 2018;34:1316–1328.
- Sharma A, Cornejo C, Mihalic J, et al. Physical characterization and in vivo organ distribution of coated iron oxide nanoparticles. Sci Rep. 2018;8:4916.
- Gruttner C, Muller K, Teller J, et al. Synthesis and antibody conjugation of magnetic nanoparticles with improved specific power absorption rates for alternating magnetic field cancer therapy. J Magn Magn Mater. 2007;311:181–186.
- Dennis CL, Jackson AJ, Borchers JA, et al. Nearly complete regression of tumors via collective behavior of magnetic nanoparticles in hyperthermia. Nanotechnology. 2009;20:395103.
- Gruttner C, Muller K, Teller J, et al. Synthesis and functionalisation of magnetic nanoparticles for hyperthermia applications. Int J Hyperthermia. 2013;29:777–789.
- Lyon PC, Gray MD, Mannaris C, et al. Safety and feasibility of ultrasound-triggered targeted drug delivery of doxorubicin from thermosensitive liposomes in liver tumours (TARDOX): a single-centre, open-label, phase 1 trial. Lancet Oncol. 2018;19:1027–1039.
- Zhou J, Li L, Li X, et al. Efficacy analysis of a novel thermochemotherapy scheme with pirarubicin for intermediate- and high-risk nonmuscle-invasive bladder cancer: a singleinstitution nonrandomized concurrent controlled trial. Int J Hyperthermia. 2019;36:867–875.
- Colombo R, Salonia A, Leib Z, et al. Long-term outcomes of a randomized controlled trial comparing thermochemotherapy with mitomycin-C alone as adjuvant treatment for non-muscle-invasive bladder cancer (NMIBC). BJU.Int. 2011;107:912–918.
- Arends TJH, Nativ O, Maffezzini M, et al. Results of a randomised controlled trial comparing intravesical chemohyperthermia with mitomycin C versus Bacillus Calmette-Guerin for adjuvant treatment of patients with intermediate- and high-risk non-muscle-invasive bladder cancer. Eur Urol. 2016;69:1046–1052.
- Tan WS, Panchal A, Buckley L, et al. Radiofrequency-induced thermo-chemotherapy effect versus a second course of Bacillus Calmette-Guérin or institutional standard in patients with recurrence of non-muscle-invasive bladder cancer following induction or maintenance Bacillus Calmette-Guérin Therapy (HYMN): a phase III, open-label, randomised controlled trial. Eur Urol. 2019;75:63–71.
- Ohno S, Tomoda M, Tomisaki S, et al. Improved surgical results after combining preoperative hyperthermia with chemotherapy and radiotherapy for patients with carcinoma of the rectum. Dis Colon Rectum. 1997;40:401–406.
- Takahashi M, Fujimoto S, Kobayashi K, et al. Clinical outcome of intraoperative pelvic hyperthermochemotherapy for patients with Dukes' C rectal cancer. Int J Hyperthermia. 1994;10:749–754.
- Huilgol NG, Gupta S, Dixit R. Chemoradiation with hyperthermia in the treatment of head and neck cancer. Int J Hyperthermia. 2010;26:21–25.
- Ohguri T, Imada H, Narisada H, et al. Systemic chemotherapy using paclitaxel and carboplatin plus regional hyperthermia and hyperbaric oxygen treatment for non-small cell lung cancer with multiple pulmonary metastases: preliminary results. Int J Hyperthermia. 2009;25:160–167.
- Moon SD, Ohguri T, Imada H, et al. Definitive radiotherapy plus regional hyperthermia with or without chemotherapy for superior sulcus tumors: a 20-year, single center experience. Lung Cancer. 2011;71:338–343.
- Kodama K, Doi O, Higashiyama M, et al. Long-term results of postoperative intrathoracic chemo-thermotherapy for lung cancer with pleural dissemination. Cancer. 1993;72:426–431.
- Ohguri T, Imada H, Nomoto S, et al. Initial experience of bladder preservation therapy using chemoradiotherapy with regional hyperthermia for muscle-invasive bladder cancer. Jpn J Hyperthermic Oncol. 2005;21:151–157.
- Nagata Y, Hiraoka M, Nishimura Y, et al. Clinical results of radiofrequency hyperthermia for malignant liver tumors. Int J Radiat Oncol Biol Phys. 1997;38:359–365.
- Murata T, Akagi K, Imamura M, et al. Studies on hyperthermia combined with arterial therapeutic blockade for treatment of tumors: (Part III) effectiveness of hyperthermia combined with arterial chemoembolization using degradable starch microspheres on advanced liver cancer. Oncol Rep. 1998;5:709–712.
- Minnaar CA, Kotzen JA, Ayeni OA, et al. The effect of modulated electro-hyperthermia on local disease control in HIV-positive and -negative cervical cancer women in South Africa: early results from a phase III randomised controlled trial. PLoS One. 2019;14:e0217894
- Harima Y, Ohguri T, Imada H, et al. A multicentre randomised clinical trial of chemoradiotherapy plus hyperthermia versus chemoradiotherapy alone in patients with locally advanced cervical cancer. Int J Hyperthermia. 2016;32:801–808.
- Ohguri T, Imada H, Yahara K, et al. Concurrent chemoradiotherapy with gemcitabine plus regional hyperthermia for locally advanced pancreatic carcinoma: initial experience. Radiat Med. 2008;26:587–596.
- Saeki H, Kawaguchi H, Kitamura K, et al. Recent advances in preoperative hyperthermochemoradiotherapy for patients with esophageal cancer. J Surg Oncol. 1998;69:224–229.
- Sugimach K, Kuwano H, Ide H, et al. Chemotherapy combined with or without hyperthermia for patients with oesophageal carcinoma: a prospective randomized trial. Int.J.Hyperthermia. 1994;10:485–493.
- Issels RD, Lindner Lh, Verweij J, Wessalowski R, et al.; European Organization for the Research and Treatment of Cancer-Soft Tissue and Bone Sarcoma Group and the European Society for Hyperthermic Oncology. Effect of neoadjuvant chemotherapy plus regional hyperthermia on long-term outcomes among patients with localized high-risk soft tissue sarcoma: the EORTC 62961-ESHO 95 Randomized Clinical Trial. JAMA Oncol. 2018;4:483–492.
- Wessalowski R, Schneider DT, Mils O, et al.; MAKEI study group. Regional deep hyperthermia for salvage treatment of children and adolescents with refractory or recurrent non-testicular malignant germ-cell tumours: an open-label, non-randomised, single-institution, phase 2 study. Lancet Oncol. 2013;14:843–852.
- Wessalowski R, Schneider DT, Mils O, et al. An approach for cure: PEI-chemotherapy and regional deep hyperthermia in children and adolescents with unresectable malignant tumors. Klin Padiatr. 2003;215:303–309.
- Seifert G, Budach V, Keilholz U, et al. Regional hyperthermia combined with chemotherapy in paediatric, adolescent and young adult patients: current and future perspectives. Radiat Oncol. 2016;11:65.
- Tschoep-Lechner KE, Milani V, Berger F, et al. Gemcitabine and cisplatin combined with regional hyperthermia as second-line treatment in patients with gemcitabine-refractory advanced pancreatic cancer. Int J Hyperthermia. 2013;29:8–16.
- Beck M, Ghadjar P, Weihrauch M, et al. Regional hyperthermia of the abdomen, a pilot study towards the treatment of peritoneal carcinomatosis. Radiat Oncol. 2015;10:157.
- Maluta S, Schaffer M, Pioli F, et al. Regional hyperthermia combined with chemoradiotherapy in primary or recurrent locally advanced pancreatic cancer : an open-label comparative cohort trial. Strahlenther Onkol. 2011;187:619–625.
- Geijsen ED, De Reijke TM, Koning CCE, et al. Combining mitomycin C and regional 70 MHz hyperthermia in patients with nonmuscle invasive bladder cancer: a pilot study. J Urol. 2015;194:1202–1208.
- Inman BA, Stauffer PR, Craciunescu OA, et al. A pilot clinical trial of intravesical mitomycin-C and external deep pelvic hyperthermia for non-muscle-invasive bladder cancer. Int J Hyperthermia. 2014;30:171–175.
- Westermann A, Mella O, van der Zee J, et al. Long-term survival data of triple modality treatment of stage IIB-III-IVA cervical cancer with the combination of radiotherapy, chemotherapy and hyperthermia – an update. Int J Hyperthermia. 2012;28:549–553.
- Hulshof MC, Van Haaren PM, van Lanschot JJ, et al. Preoperative chemoradiation combined with regional hyperthermia for patients with resectable esophageal cancer. Int J Hyperthermia. 2009;25:79–85.
- Cho C, Wust P, Hildebrandt B, et al. Regional hyperthermia of the abdomen in conjunction with chemotherapy for peritoneal carcinomatosis: evaluation of two annular-phased-array applicators. Int J Hyperthermia. 2008;24:399–408.
- Petrovich Z, Langholz B, Kapp DS, et al. Deep regional hyperthermia of the liver. A clinical study of 49 patients. Am J Clin Oncol. 1989;12:378–383.
- van Driel WJ, Koole SN, Sikorska K, et al. Hyperthermic Intraperitoneal Chemotherapy in Ovarian Cancer. N Engl J Med. 2018;378:230–240.
- Bonnot PE, Piessen G, Kepenekian V, et al.; FREGAT and BIG-RENAPE Networks. Cytoreductive surgery with or without hyperthermic intraperitoneal chemotherapy for gastric cancer with peritoneal metastases (CYTO-CHIP study): a propensity score analysis. J Clin Oncol. 2019;37:2028–2040.
- Verwaal VJ, van Ruth S, de Bree E, et al. Randomized trial of cytoreduction and hyperthermic intraperitoneal chemotherapy versus systemic chemotherapy and palliative surgery in patients with peritoneal carcinomatosis of colorectal cancer. J Clin Oncol. 2003;21:3737–3743.
- Quenet F, Elias D, Roca L, et al.; UNICANCER-GI Group and the French BIG-Renape Group. A UNICANCER phase III trial of hyperthermic intra-peritoneal chemotherapy (HIPEC) for colorectal peritoneal carcinomatosis (PC): PRODIGE 7. J Clin Oncol. 2018;36:LBA3503.
- Koops HS, Vaglini M, Suciu S, et al. Prophylactic isolated limb perfusion for localized, high-risk limb melanoma: results of a multicenter randomized phase III trial. European Organization for Research and Treatment of Cancer Malignant Melanoma Cooperative Group Protocol 18832, the World Health Organization Melanoma Program Trial 15, and the North American Perfusion Group Southwest Oncology Group-8593. J Clin Oncol. 1998;16:2906–2912.
- Eggermont AM, de Wilt JH, ten Hagen TL. Current uses of isolated limb perfusion in the clinic and a model system for new strategies. Lancet Oncol. 2003;4:429–437.
- Van Vulpen M, Raaymakers BW, Lagendijk JJW, et al. Three-dimensional controlled interstitial hyperthermia combined with radiotherapy for locally advanced prostate carcinoma-a feasibility study. Int J Radiat Oncol Biol Phys. 2002;53:116–126.
- Kukielka AM, Hetnal M, Bereza K. Evaluation of tolerance and toxicity of high-dose-rate brachytherapy boost combined with interstitial hyperthermia for prostate cancer. Int J Hyperthermia. 2016;32:324–330.
- Sneed PK, Stauffer PR, McDermott MW, et al. Survival benefit of hyperthermia in a prospective randomized trial of brachytherapy boost +/- hyperthermia for glioblastoma multiforme. Int J Radiat Oncol Biol Phys. 1998;40:287–295.
- Engin K, Tupchong L, Waterman FM, et al. Thermoradiotherapy with combined interstitial and external hyperthermia in advanced tumours in the head and neck with depth > or = 3 cm. Int J Hyperthermia. 1993;9:645–654.
- Maier-Hauff K, Ulrich F, Nestler D, et al. Efficacy and safety of intratumoral thermotherapy using magnetic iron-oxide nanoparticles combined with external beam radiotherapy on patients with recurrent glioblastoma multiforme. J Neurooncol. 2011;103:317–324.
- Johannsen M, Thiesen B, Wust P, et al. Magnetic nanoparticle hyperthermia for prostate cancer. Int J Hyperthermia. 2010;26:790–795.
- Ren RL, Chou CK, Vora N, et al. A pilot study of intracavitary hyperthermia combined with radiation in the treatment of oesophageal carcinoma. Int J Hyperthermia. 1998;14:245–254.
- You QS, Wang RZ, Suen GQ, et al. Combination preoperative radiation and endocavitary hyperthermia for rectal cancer: long-term results of 44 patients. Int J Hyperthermia. 1993;9:19–24.
- Huilgol NG, Gupta S, Sridhar CR. Hyperthermia with radiation in the treatment of locally advanced head and neck cancer: a report of randomized trial. J Can Res Ther. 2010;6:492–496.
- Abe M, Hiraoka M, Takahashi M, et al. Multi-institutional studies on hyperthermia using an 8-MHz radiofrequency capacitive heating device (Thermotron RF-8) in combination with radiation for cancer therapy. Cancer. 1986;58:1589–1595.
- Matsuda T, Tanaka Y, Takeshita N, et al. Clinical significance of the combined use of radiation therapy and hyperthermia]. Gan to Kagaku Ryoho. 1987;14:1508–1514.
- van Stam G, Kok HP, Hulshof M, et al. A flexible 70 MHz phase-controlled double waveguide system for hyperthermia treatment of superficial tumours with deep infiltration . Int J Hyperthermia. 2017;33:796–809.
- Crezee J, Van Tienhoven G, Kolff MW, et al. Development of a 70 MHz unit for hyperthermia treatment of deep-seated breast tumors. Int J Microw Wireless Technol. 2017;9:1317–1324.
- Verduijn GM, de Wee EM, Rijnen Z, et al. Deep hyperthermia with the HYPERcollar system combined with irradiation for advanced head and neck carcinoma – a feasibility study. Int J Hyperthermia. 2018;34:994–1001.
- Vernon CC, Hand JW, Field SB, et al. Radiotherapy with or without hyperthermia in the treatment of superficial localized breast cancer: results from five randomized controlled trials. International Collaborative Hyperthermia Group. Int.J.Radiat.Oncol.Biol.Phys. 1996;35:731–744.
- Overgaard J, González González D, Hulshof M, et al. Randomised trial of hyperthermia as adjuvant to radiotherapy for recurrent or metastatic malignant melanoma. European Society for Hyperthermic Oncology. Lancet. 1995;345:540–543.
- Overgaard J, Gonzalez DG, Hulshof M, et al. Hyperthermia as an adjuvant to radiation therapy of recurrent or metastatic malignant melanoma. A multicentre randomized trial by the European Society for Hyperthermic Oncology. International Journal of Hyperthermia. 1996;12:3–20.
- Arcangeli G, Benassi M, Cividalli A, Radiotherapy and hyperthermia, et al. Analysis of clinical results and identification of prognostic variables. Cancer. 1987;60:950–956.
- Valdagni R, Amichetti M. Report of long-term follow-up in a randomized trial comparing radiation therapy and radiation therapy plus hyperthermia to metastatic lymph nodes in stage IV head and neck patients. Int J Radiat Oncol Biol Phys. 1994;28:163–169.
- Ohguri T, Imada H, Yahara K, et al. Radiotherapy with 8-MHz radiofrequency-capacitive regional hyperthermia for stage III non-small-cell lung cancer: the radiofrequency-output power correlates with the intraesophageal temperature and clinical outcomes. Int J Radiat Oncol Biol Phys. 2009;73:128–135.
- Ohguri T, Imada H, Yahara K, et al. Re-irradiation plus regional hyperthermia for recurrent non-small cell lung cancer: a potential modality for inducing long-term survival in selected patients. Lung Cancer. 2012;77:140–145.
- Ohguri T, Yahara K, Moon SD, et al. Deep regional hyperthermia for the whole thoracic region using 8 MHz radiofrequency-capacitive heating device: relationship between the radiofrequency-output power and the intra-oesophageal temperature and predictive factors for a good heating in 59 patients. Int J Hyperthermia. 2011;27:20–26.
- Tomura K, Ohguri T, Mulder HT, et al. The usefulness of mobile insulator sheets for the optimization of deep heating area for regional hyperthermia using a capacitively-coupled heating method: phantom, simulation and clinical prospective studies. Int J Hyperthermia. 2018;34:1092–1103.
- Yahara K, Ohguri T, Yamaguchi S, et al. Definitive radiotherapy plus regional hyperthermia for high-risk and very high-risk prostate carcinoma: thermal parameters correlated with biochemical relapse-free survival. Int J Hyperthermia. 2015;31:600–608.
- Masunaga SI, Hiraoka M, Akuta K, et al. Phase I/II trial of preoperative thermoradiotherapy in the treatment of urinary bladder cancer. Int J Hyperthermia. 1994;10:31–40.
- Ohguri T, Imada H, Kato F, et al. Radiotherapy with 8 MHz radiofrequency-capacitive regional hyperthermia for pain relief of unresectable and recurrent colorectal cancer. Int J Hyperthermia. 2006;22:1–14.
- Ohguri T, Imada H, Yahara K, et al. Effect of 8-MHz radiofrequency-capacitive regional hyperthermia with strong superficial cooling for unresectable or recurrent colorectal cancer. Int J Hyperthermia. 2004;20:465–475.
- Chi MS, Yang KL, Chang YC, et al. Comparing the effectiveness of combined external beam radiation and hyperthermia versus external beam radiation alone in treating patients with painful bony metastases: a phase 3 prospective, randomized, controlled trial. Int J Radiat Oncol Biol Phys. 2018;100:78–87.
- Harima Y, Nagata K, Harima K, et al. A randomized clinical trial of radiation therapy versus thermoradiotherapy in stage IIIB cervical carcinoma. Int J Hyperthermia. 2001;17:97–105.
- Yamada S, Takai Y, Nemoto K, et al. Intraoperative radiation therapy combined with hyperthermia against pancreatic carcinoma. Tohoku J Exp Med. 1992;166:395–401.
- Yamaguchi S, Ohguri T, Imada H, et al. Multimodal approaches including three-dimensional conformal re-irradiation for recurrent or persistent esophageal cancer: preliminary results. J Radiat Res. 2011;52:812–820.
- Van der Zee J, González González D, Van Rhoon GC, et al. Comparison of radiotherapy alone with radiotherapy plus hyperthermia in locally advanced pelvic tumours: a prospective, randomised, multicentre trial. Dutch Deep Hyperthermia Group. Lancet. 2000;355:1119–1125.
- Maluta S, Dall'Oglio S, Romano M, et al. Conformal radiotherapy plus local hyperthermia in patients affected by locally advanced high risk prostate cancer: preliminary results of a prospective phase II study. Int J Hyperthermia. 2007;23:451–456.
- Tilly W, Gellermann J, Graf R, et al. Regional hyperthermia in conjunction with definitive radiotherapy against recurrent or locally advanced prostate cancer T3 pN0 M0. Strahlenther Onkol. 2005;181:35–41.
- Lubner MG, Brace CL, Hinshaw JL, et al. Microwave tumor ablation: mechanism of action, clinical results, and devices. J Vasc Interv Radiol. 2010;21:S192–S203.
- Rosenthal DI, Hornicek FJ, Torriani M, et al. Osteoid osteoma: percutaneous treatment with radiofrequency energy. Radiology. 2003;229:171–175.
- Phoa KN, van Vilsteren FG, Weusten BL, et al. Radiofrequency ablation vs endoscopic surveillance for patients with Barrett esophagus and low-grade dysplasia: a randomized clinical trial. JAMA. 2014;311:1209–1217.
- Tan W, Deng Q, Lin S, et al. Comparison of microwave ablation and radiofrequency ablation for hepatocellular carcinoma: a systematic review and meta-analysis. Int J Hyperthermia. 2019;36:264–272.
- Luo W, Zhang Y, He G, et al. Effects of radiofrequency ablation versus other ablating techniques on hepatocellular carcinomas: a systematic review and meta-analysis. World J Surg Onc. 2017;15:126.
- Bi N, Shedden K, Zheng X, et al. Comparison of the effectiveness of radiofrequency ablation with stereotactic body radiation therapy in inoperable stage I non-small cell lung cancer: a systemic review and pooled analysis. Int J Radiat Oncol Biol Phys. 2016;95:1378–1390.
- Tsakok MT, Jones D, MacNeill A, et al. Is microwave ablation more effective than radiofrequency ablation in achieving local control for primary pulmonary malignancy? Interact Cardiovasc Thorac Surg. 2019.
- Sidoff L, Dupuy DE. Clinical experiences with microwave thermal ablation of lung malignancies. Int J Hyperthermia. 2017;33:25–33.
- Hamou AB, Ghanassia E, Espiard SA, et al. Safety and efficacy of thermal ablation (radiofrequency and laser): should we treat all types of thyroid nodules? Int J Hyperthermia. 2019;36:666–676.
- Wang L, Xu D, Yang Y, et al. Safety and efficacy of ultrasound-guided percutaneous thermal ablation in treating low-risk papillary thyroid microcarcinoma: a pilot and feasibility study. J Cancer Res Ther. 2019;15:1522–1529.
- Cho SJ, Baek JH, Chung SR, et al. Thermal ablation for small papillary thyroid cancer: a systematic review. Thyroid. 2019;29:1774–1783.
- Papini E, Pacella CM, Solbiati LA, et al. G, Minimally-invasive treatments for benign thyroid nodules: a Delphi-based consensus statement from the Italian minimally-invasive treatments of the thyroid (MITT) group. Int J Hyperthermia. 2019;36:376–382.
- Filippiadis D, Mauri G, Marra P, et al. F, Percutaneous ablation techniques for renal cell carcinoma: current status and future trends. Int J Hyperthermia. 2019;36:21–30.
- Higgins LJ, Hong K. Renal ablation techniques: state of the art. AJR Am J Roentgenol. 2015;205:735–741.
- Garcia-Tejedor A, Guma A, Soler T, et al. Radiofrequency ablation followed by surgical excision versus lumpectomy for early stage breast cancer: a randomized phase II clinical trial. Radiology. 2018;289:317–324.
- Pouw RE, Klaver E, Phoa KN, et al. Radiofrequency ablation for low-grade dysplasia in Barrett’s esophagus: long-term outcome of a randomized trial. Gastrointest Endosc. 2020.
- Saccomandi P, Lapergola A, Longo F, et al. Thermal ablation of pancreatic cancer: a systematic literature review of clinical practice and pre-clinical studies. Int J Hyperthermia. 2018;35:398–418.
- Vietti Violi N, Duran R, Guiu B, et al. Efficacy of microwave ablation versus radiofrequency ablation for the treatment of hepatocellular carcinoma in patients with chronic liver disease: a randomised controlled phase 2 trial. Lancet Gastroenterol Hepatol. 2018;3:317–325.
- Macchi M, Belfiore MP, Floridi C, et al. Radiofrequency versus microwave ablation for treatment of the lung tumours: LUMIRA (lung microwave radiofrequency) randomized trial. Med Oncol. 2017;34:96.
- Vogl TJ, Eckert R, Naguib NN, et al. Thermal ablation of colorectal lung metastases: retrospective comparison among laser-induced thermotherapy, radiofrequency ablation, and microwave ablation. AJR Am J Roentgenol. 2016;207:1340–1349.
- Narsule CK, Sridhar P, Nair D, et al. Percutaneous thermal ablation for stage IA non-small cell lung cancer: long-term follow-up. J Thorac Dis. 2017;9:4039–4045.
- Wang L, Ke Q, Lin N, et al. The efficacy of transarterial chemoembolization combined with microwave ablation for unresectable hepatocellular carcinoma: a systematic review and meta-analysis. Int J Hyperthermia. 2019;36:1288–1296.
- Barnett GH, Voigt JD, Alhuwalia MS. A systematic review and meta-analysis of studies examining the use of brain laser interstitial thermal therapy versus craniotomy for the treatment of high-grade tumors in or near areas of eloquence: an examination of the extent of resection and major complication rates associated with each type of surgery. Stereotact Funct Neurosurg. 2016;94:164–173.
- Ahluwalia M, Barnett GH, Deng D, et al. Laser ablation after stereotactic radiosurgery: a multicenter prospective study in patients with metastatic brain tumors and radiation necrosis. J Neurosurg. 2019;130:804–811.
- Kramer MW, Wolters M, Cash H, et al. Current evidence of transurethral Ho:YAG and Tm:YAG treatment of bladder cancer: update 2014. World J Urol. 2015;33:571–579.
- Woods E. Laser ablation of the prostate: a safe effective treatment of obstructive benign prostatic disease. Can Urol Assoc J. 2010;4:344–346.
- Al-Ansari A, Younes N, Sampige VP, et al. GreenLight HPS 120-W laser vaporization versus transurethral resection of the prostate for treatment of benign prostatic hyperplasia: a randomized clinical trial with midterm follow-up. Eur Urol. 2010;58:349–355.
- Schlottmann F, Patti MG. Current concepts in treatment of Barrett's esophagus with and without dysplasia. J Gastrointest Surg. 2017;21:1354–1360.
- Tucker RD, Platz CE, Huidobro C, et al. Interstitial thermal therapy in patients with localized prostate cancer: histologic analysis. Urology. 2002;60:166–169.
- Sherar MD, Trachtenberg J, Davidson SR, et al. Interstitial microwave thermal therapy for prostate cancer. J Endourol. 2003;17:617–625.
- Chopra R, Colquhoun A, Burtnyk M, et al. L, MR imaging-controlled transurethral ultrasound therapy for conformal treatment of prostate tissue: initial feasibility in humans. Radiology. 2012;265:303–313.
- Elias WJ, Lipsman N, Ondo WG, et al. A randomized trial of focused ultrasound thalamotomy for essential tremor. N Engl J Med. 2016;375:730–739.
- Ikink ME, Voogt MJ, Verkooijen HM, et al. Mid-term clinical efficacy of a volumetric magnetic resonance-guided high-intensity focused ultrasound technique for treatment of symptomatic uterine fibroids. Eur Radiol. 2013;23:3054–3061.
- Łoziński T, Filipowska J, Gurynowicz G, et al. The effect of high-intensity focused ultrasound guided by magnetic resonance therapy on obstetrical outcomes in patients with uterine fibroids - experiences from the main Polish center and a review of current data. Int J Hyperthermia. 2019;36:582–590.
- Pron G. Magnetic resonance-guided high-intensity focused ultrasound (MRgHIFU) treatment of symptomatic uterine fibroids: an evidence-based analysis. Ont Health Technol Assess Ser. 2015;15:1–86.
- Hurwitz MD, Ghanouni P, Kanaev SV, et al. Magnetic resonance-guided focused ultrasound for patients with painful bone metastases: phase III trial results. J Natl Cancer Inst. 2014;106:dju082.
- Golan R, Bernstein AN, McClure TD, et al. Partial gland treatment of prostate cancer using high-intensity focused ultrasound in the primary and salvage settings: a systematic review. J Urol. 2017;198:1000–1009.
- Guillaumier S, Peters M, Arya M, et al. A multicentre study of 5-year outcomes following focal therapy in treating clinically significant nonmetastatic prostate cancer. Eur Urol. 2018;74:422–429.
- Rischmann P, Gelet A, Riche B, et al. Focal high intensity focused ultrasound of unilateral localized prostate cancer: a prospective multicentric hemiablation study of 111 patients. Eur Urol. 2017;71:267–273.
- Ghai S, Perlis N, Lindner U, et al. Magnetic resonance guided focused high frequency ultrasound ablation for focal therapy in prostate cancer – phase 1 trial. Eur Radiol. 2018;28:4281–4287.
- Tay KJ, Cheng CWS, Lau WKO, et al. Focal therapy for prostate cancer with in-bore MR-guided focused ultrasound: two-year follow-up of a phase i trial-complications and functional outcomes. Radiology. 2017;285:620–628.
- Crouzet S, Blana A, Murat FJ, et al. Salvage high-intensity focused ultrasound (HIFU) for locally recurrent prostate cancer after failed radiation therapy: multi-institutional analysis of 418 patients. BJU Int. 2017;119:896–904.
- Cordeiro ER, Cathelineau X, Thuroff S, et al. High-intensity focused ultrasound (HIFU) for definitive treatment of prostate cancer. BJU Int. 2012;110:1228–1242.
- Dababou S, Marrocchio C, Rosenberg J, et al. A meta-analysis of palliative treatment of pancreatic cancer with high intensity focused ultrasound. J Ther Ultrasound. 2017;5:9.
- Peek MCL, Ahmed M, Scudder J, et al.; on behalf of the HIFU-F Collaborators. High-intensity focused ultrasound in the treatment of breast fibroadenomata (HIFU-F trial). Int J Hyperthermia. 2018;34:1002–1009.
- Yu W, Tang L, Lin F, et al. Significance of HIFU in local unresectable recurrence of soft tissue sarcoma, a single-center, respective, case series in China. Surg Oncol. 2019;30:117–121.
- Sharma KV, Yarmolenko PS, Eranki A, et al. Magnetic resonance imaging-guided high-intensity focused ultrasound applications in pediatrics: early experience at Children’s National Medical Center. Top Magn Reson Imaging. 2018;27:45–51.
- Illing RO, Kennedy JE, Wu F, et al. The safety and feasibility of extracorporeal high-intensity focused ultrasound (HIFU) for the treatment of liver and kidney tumours in a Western population. Br J Cancer. 2005;93:890–895.
- Melodelima D, Prat F, Fritsch J, et al. Treatment of esophageal tumors using high intensity intraluminal ultrasound: first clinical results. J Transl Med. 2008;6:28.
- Maloney E, Hwang JH. Emerging HIFU applications in cancer therapy. Int J Hyperthermia. 2015;31:302–309.
- Zhao WP, Zhang J, Han ZY, et al. A clinical investigation treating different types of fibroids identified by MRI-T2WI imaging with ultrasound guided high intensity focused ultrasound. Sci Rep. 2017;7:10812.
- Scheffer HJ, Nielsen K, de Jong MC, et al. Irreversible electroporation for nonthermal tumor ablation in the clinical setting: a systematic review of safety and efficacy. J Vasc Interv Radiol. 2014;25:997–1011. Quiz 1011.
- Scheffer HJ, Vroomen L, de Jong MC, et al. Ablation of locally advanced pancreatic cancer with percutaneous irreversible electroporation: results of the phase I/II PANFIRE study. Radiology. 2017;282:585–597.
- Vogel JA, Rombouts SJ, de Rooij T, et al. Induction chemotherapy followed by resection or irreversible electroporation in locally advanced pancreatic cancer (IMPALA): a prospective cohort study. Ann Surg Oncol. 2017;24:2734–2743.
- Kourounis G, Tabet PP, Moris D, et al. Irreversible electroporation (Nanoknife (R) treatment) in the field of hepatobiliary surgery: current status and future perspectives. J Buon. 2017;22:141–149.
- Guenther E, Klein N, Zapf S, et al. Prostate cancer treatment with irreversible electroporation (IRE): safety, efficacy and clinical experience in 471 treatments. Plos One. 2019;14:e0215093.
- Ricke J, Jurgens JHW, Deschamps F, et al. Irreversible electroporation (IRE) fails to demonstrate efficacy in a prospective multicenter phase II trial on lung malignancies: the ALICE trial. Cardiovasc Intervent Radiol. 2015;38:401–408.
- Soria F, Milla P, Fiorito C, et al. Efficacy and safety of a new device for intravesical thermochemotherapy in non-grade 3 BCG recurrent NMIBC: a phase I-II study. World J Urol. 2016;34:189–195.
- Ba M, Cui S, Wang B, et al. Bladder intracavitary hyperthermic perfusion chemotherapy for the prevention of recurrence of non-muscle invasive bladder cancer after transurethral resection. Oncol Rep. 2017;37:2761–2770.
- Okamura T, Ueda K, Hashimoto Y, et al. Immunohistochemical evaluation of p53 and retinoblastoma proteins in relation to hyperthermia treatment: results in human urothelial carcinomas. Int J Hyperthermia. 1996;12:813–824.
- Merten R, Ott O, Haderlein M, et al. Long-term experience of chemoradiotherapy combined with deep regional hyperthermia for organ preservation in high-risk bladder cancer (Ta, Tis, T1, T2). Oncologist. 2019;24:e1341–e1350.
- Mohammadi AM, Schroeder JL. Laser interstitial thermal therapy in treatment of brain tumors-the NeuroBlate System. Expert Rev Med Devices. 2014;11:109–119.
- Sneed PK, Gutin PH, Stauffer PR, et al. Thermoradiotherapy of recurrent malignant brain tumors. Int J Radiat Oncol Biol Phys. 1992;23:853–861.
- Hulshof MC, Raaymakers BW, Lagendijk JJ, et al. A feasibility study of interstitial hyperthermia plus external beam radiotherapy in glioblastoma multiforme using the Multi ELectrode Current Source (MECS) system. Int J Hyperthermia. 2004;20:451–463.
- Rodriguez A, Tatter SB. Laser ablation of recurrent malignant gliomas: current status and future perspective. Neurosurgery. 2016;79:S35–S39.
- He M, Jacobson H, Zhang C, et al. A retrospective study of ultrasound-guided high intensity focussed ultrasound ablation for multiple uterine fibroids in South Africa. Int J Hyperthermia. 2018;34:1304–1310.
- Franckena M, Stalpers LJ, Koper PC, et al. Long-term improvement in treatment outcome after radiotherapy and hyperthermia in locoregionally advanced cervix cancer: an update of the Dutch Deep Hyperthermia Trial. Int J Radiat Oncol Biol Phys. 2008;70:1176–1182.
- Notter M, Piazena H, Vaupel P. Hypofractionated re-irradiation of large-sized recurrent breast cancer with thermography-controlled, contact-free water-filtered infra-red-A hyperthermia: a retrospective study of 73 patients. Int J Hyperthermia. 2017;33:227–236.
- van der Zee J, van der Holt B, Rietveld PJ, et al. Reirradiation combined with hyperthermia in recurrent breast cancer results in a worthwhile local palliation. Br J Cancer. 1999;79:483–490.
- Samulski TV, Grant WJ, Oleson JR, et al. Clinical experience with a multi-element ultrasonic hyperthermia system: analysis of treatment temperatures. Int J Hyperthermia. 1990;6:909–922.
- Zagar TM, Oleson JR, Vujaskovic Z, et al. Hyperthermia combined with radiation therapy for superficial breast cancer and chest wall recurrence: a review of the randomised data. Int J Hyperthermia. 2010;26:612–617.
- Jones EL, Oleson JR, Prosnitz LR, et al. Randomized trial of hyperthermia and radiation for superficial tumors. J Clin Oncol. 2005;23:3079–3085.
- Shin BJ, Chick JF, Stavropoulos SW. Contemporary status of percutaneous ablation for the small renal mass. Curr Urol Rep. 2016;17:23.
- Amabile C, Ahmed M, Solbiati L, et al. Microwave ablation of primary and secondary liver tumours: ex vivo, in vivo, and clinical characterisation. Int J Hyperthermia. 2017;33:34–42.
- Filippiadis DK, Spiliopoulos S, Konstantos C, et al. Computed tomography-guided percutaneous microwave ablation of hepatocellular carcinoma in challenging locations: safety and efficacy of high-power microwave platforms. Int J Hyperthermia. 2018;34:863–869.
- Imajo K, Tomeno W, Kanezaki M, et al. New microwave ablation system for unresectable liver tumors that forms large, spherical ablation zones. J Gastroenterol Hepatol. 2018;33:2007–2014.
- Loriaud A, Denys A, Seror O, et al. A, Hepatocellular carcinoma abutting large vessels: comparison of four percutaneous ablation systems. Int J Hyperthermia. 2018;34:1171–1178.
- Smolock AR, Cristescu MM, Potretzke TA, et al. Microwave ablation for the treatment of hepatic adenomas. J Vasc Interv Radiol. 2016;27:244–249.
- Vogl TJ, Roman A, Nour-Eldin NA, et al. A comparison between 915 MHz and 2450 MHz microwave ablation systems for the treatment of small diameter lung metastases. Diagn Interv Radiol. 2018;24:31–37.
- Ierardi AM, Coppola A, Lucchina N, et al. Treatment of lung tumours with high-energy microwave ablation: a single-centre experience. Med Oncol. 2017;34:5.
- Klaver CEL, Stam R, Sloothaak DAM, et al. Colorectal cancer at high risk of peritoneal metastases: long term outcomes of a pilot study on adjuvant laparoscopic HIPEC and future perspectives. Oncotarget. 2017;8:51200–51209.
- Cowan RA, O'Cearbhaill RE, Zivanovic O, et al. Current status and future prospects of hyperthermic intraoperative intraperitoneal chemotherapy (HIPEC) clinical trials in ovarian cancer. Int J Hyperthermia. 2017;33:548–553.
- Pinto A, Eveno C, Pocard M. Update on clinical trials in colorectal cancer peritoneal metastasis. Int J Hyperthermia. 2017;33:543–547.
- Goere D, Passot G, Gelli M, et al. Complete cytoreductive surgery plus HIPEC for peritoneal metastases from unusual cancer sites of origin: results from a worldwide analysis issue of the Peritoneal Surface Oncology Group International (PSOGI). Int J Hyperthermia. 2017;33:520–527.
- Rovers KP, de Bree E, Yonemura Y, et al. Treatment of peritoneal metastases from small bowel adenocarcinoma. Int J Hyperthermia. 2017;33:571–578.
- Ryan TP, Brace CL. Interstitial microwave treatment for cancer: historical basis and current techniques in antenna design and performance. Int J Hyperthermia. 2017;33:3–14.
- Salgaonkar VA, Diederich CJ. Catheter-based ultrasound technology for image-guided thermal therapy: current technology and applications. Int J Hyperthermia. 2015;31:203–215.
- Dobsicek Trefna H, Schmidt M, van Rhoon GC, et al. Quality assurance guidelines for interstitial hyperthermia. Int J Hyperthermia. 2019;36:277–294.
- Stauffer PR, Diederich CJ, Seegenschmiedt MH. Interstitial heating technologies. In: Seegenschmiedt MH, Fessenden P, Vernon CC, editors. Thermoradiotherapy and thermochemotherapy. Volume 1, Biology, physiology and physics. Berlin: Springer Verlag; 1995. p. 279–320.
- Fallahi H, Prakash P. Antenna designs for microwave tissue ablation. Crit Rev Biomed Eng. 2018;46:495–521.
- Schreier K, Budihna M, Lesnicar H, et al. Preliminary studies of interstitial hyperthermia using hot water. Int J Hyperthermia. 1990;6:431–444.
- Deford JA, Babbs CF, Patel UH, et al. Effective estimation and computer control of minimum tumour temperature during conductive interstitial hyperthermia. Int J Hyperthermia. 1991;7:441–453.
- Stauffer PR, Cetas TC, Fletcher AM, et al. Observations on the use of ferromagnetic implants for inducing hyperthermia. IEEE Trans Biomed Eng. 1984;31:76–90.
- Steger AC, Lees WR, Walmsley K, et al. Interstitial laser hyperthermia: a new approach to local destruction of tumours. BMJ. 1989;299:362–365.
- Satoh T, Stauffer PR, Fike JR. Thermal distribution studies of helical-coil microwave antennas for interstitial hyperthermia. Int J Radiat Oncol Biol Phys. 1988;15:1209–1218.
- Bertram JM, Yang D, Converse MC, et al. A review of coaxial-based interstitial antennas for hepatic microwave ablation. Crit Rev Biomed Eng. 2006;34:187–213.
- O’Rourke AP, Haemmerich D, Prakash P, et al. Current status of liver tumor ablation devices. Expert Rev Med Devices. 2007;4:523–537.
- Astrahan MA, Norman A. A localized current field hyperthermia system for use with 192-iridium interstitial implants. Med Phys. 1982;9:419–424.
- Visser AG, Deurloo IKK, Levendag PC, et al. An interstitial hyperthermia system at 27 MHz. Int J Hyperthermia. 1989;5:265–276.
- Kaatee R, Crezee H, Kanis BP, et al. Spatial temperature control with a 27 MHz current source interstitial hyperthermia system. Int J Radiat Oncol Biol Phys. 1997;37:189–197.
- van der Koijk JF, Lagendijk JJW, Crezee J, et al. The influence of vasculature on temperature distributions in MECS interstitial hyperthermia: importance of longitudinal control. Int J Hyperthermia. 1997;13:365–385.
- Diederich CJ. Ultrasound applicators with integrated catheter-cooling for interstitial hyperthermia: theory and preliminary experiments. Int J Hyperthermia. 1996;12:279–297. Discussion 299–300.
- Emami B. Interstitial thermoradiotherapy in the treatment of malignant tumors. In: Urano M, Douple E, editors. Interstitial hyperthermia: physics, biology and clinical aspects. Vol. 3. Utrecht (The Netherlands): VSP; 1992. p. 199–220.
- Pyrexar Medical Inc. 2019. “BSD-500 Features”. Available from: http://www.pyrexar.com/hyperthermia/bsd-500
- Nau WH, Diederich CJ, Stauffer PR. Directional power deposition from direct-coupled and catheter-cooled interstitial ultrasound applicators. Int J Hyperthermia. 2000;16:129–144.
- Chopra R, Burtnyk M, N’djin WA, et al. M, MRI-controlled transurethral ultrasound therapy for localised prostate cancer. Int J Hyperthermia. 2010;26:804–821.
- Bihrle R, Foster RS, Sanghvi NT, et al. High-intensity focused ultrasound in the treatment of prostatic tissue. Urology. 1994;43:21–26.
- Gelet A, Chapelon JY, Margonari J, et al. High-intensity focused ultrasound experimentation on human benign prostatic hypertrophy. Eur Urol. 1993;23:44–47.
- Hutchinson EB, Hynynen K. Intracavitary ultrasound phased arrays for prostate thermal therapies: MRI compatibility and in vivo testing. Med Phys. 1998;25:2392–2399.
- Ahmed M, Brace CL, Lee FT, Jr, et al. Principles of and advances in percutaneous ablation. Radiology. 2011;258:351–369.
- Hoffmann R, Rempp H, Erhard L, et al. Comparison of four microwave ablation devices: an experimental study in ex vivo bovine liver. Radiology. 2013;268:89–97.
- Sun Y, Cheng Z, Dong L, et al. Comparison of temperature curve and ablation zone between 915- and 2450-MHz cooled-shaft microwave antenna: results in ex vivo porcine livers. Eur J Radiol. 2012;81:553–557.
- Sawicki JF, Shea JD, Behdad N, et al. The impact of frequency on the performance of microwave ablation. Int J Hyperthermia. 2017;33:61–68.
- Cavagnaro M, Amabile C, Bernardi P, et al. A minimally invasive antenna for microwave ablation therapies: design, performances, and experimental assessment. IEEE Trans Biomed Eng. 2011;58:949–959.
- Al-Hakim RA, Abtin FG, Genshaft SJ, et al. Defining new metrics in microwave ablation of pulmonary tumors: ablation work and ablation resistance score. J Vasc Interv Radiol. 2016;27:1380–1386.
- Crezee J, Lagendijk JJ. Temperature uniformity during hyperthermia: the impact of large vessels. Phys Med Biol. 1992;37:1321–1337.
- Bredlau AL, McCrackin MA, Motamarry A, Helke K, et al. Thermal therapy approaches for treatment of brain tumors in animals and humans. Crit Rev Biomed Eng. 2016;44:443–457.
- Carpentier A, McNichols RJ, Stafford RJ, et al. Laser thermal therapy: real-time MRI-guided and computer-controlled procedures for metastatic brain tumors. Lasers Surg Med. 2011;43:943–950.
- Goffinet DR, Prionas SD, Kapp DS, et al. Interstitial 192Ir flexible catheter radiofrequency hyperthermia treatments of head and neck and recurrent pelvic carcinomas. Int J Radiat Oncol Biol Phys. 1990;18:199–210.
- Prionas SD, Kapp DS, Goffinet DR, et al. Thermometry of interstitial hyperthermia given as an adjuvant to brachytherapy for the treatment of carcinoma of the prostate. Int J Radiat Oncol Biol Phys. 1994;28:151–162.
- Gillams A, Goldberg N, Ahmed M, et al. Thermal ablation of colorectal liver metastases: a position paper by an international panel of ablation experts, The Interventional Oncology Sans Frontières meeting 2013. Eur Radiol. 2015;25:3438–3454. 2015
- Weiss J, Winkelmann MT, Gohla G, et al. MR-guided microwave ablation in hepatic malignancies: clinical experiences from 50 procedures. Int J Hyperthermia. 2020;37:349–355.
- Alattar AA, Bartek J, Chian VL, et al. Stereotactic laser ablation as treatment of brain metastases recurring after stereotactic radiosurgery: a systematic literature review. World Neurosurgery. 2019;128:134–142.
- Fucused Ultrasound Foundation. Available from: https://www.fusfoundation.org/the-technology/state-of-the-technology.
- Peek MCL, Ahmed M, Napoli A, et al. Minimally invasive ablative techniques in the treatment of breast cancer: a systematic review and meta-analysis. Int J Hyperthermia. 2017;33:191–202.
- Insightec. Available from: https://www.insightec.com
- Hijnen N, Langereis S, Grull H. Magnetic resonance guided high-intensity focused ultrasound for image-guided temperature-induced drug delivery. Adv Drug Deliv Rev. 2014;72:65–81.
- Salgaonkar VA, Prakash P, Rieke V, et al. Model-based feasibility assessment and evaluation of prostate hyperthermia with a commercial MR-guided endorectal HIFU ablation array. Med Phys. 2014;41:033301.
- Ozhinsky E, Salgaonkar VA, Diederich CJ, et al. MR thermometry-guided ultrasound hyperthermia of user-defined regions using the ExAblate prostate ablation array. J Ther Ultrasound. 2018;6:7.
- Partanen A, Yarmolenko PS, Viitala A, et al. Mild hyperthermia with magnetic resonance-guided high-intensity focused ultrasound for applications in drug delivery. Int J Hyperthermia. 2012;28:320–336.
- Hijnen NM, Heijman E, Kohler MO, et al. Tumour hyperthermia and ablation in rats using a clinical MR-HIFU system equipped with a dedicated small animal set-up. Int J Hyperthermia. 2012;28:141–155.
- Lam MK, Oerlemans C, Froeling M, et al. DCE-MRI and IVIM-MRI of rabbit Vx2 tumors treated with MR-HIFU-induced mild hyperthermia. J Ther Ultrasound. 2016;4:9.
- Farr N, Wang YN, D’Andrea S, et al. Hyperthermia-enhanced targeted drug delivery using magnetic resonance-guided focussed ultrasound: a pre-clinical study in a genetic model of pancreatic cancer. Int J Hyperthermia. 2018;34:284–291.
- Hijnen N, Kneepkens E, de Smet M, et al. Thermal combination therapies for local drug delivery by magnetic resonance-guided high-intensity focused ultrasound. Proc Natl Acad Sci U S A. 2017;114:E4802–E4811.
- Bing CC, Patel P, Staruch RM, et al. Longer heating duration increases localized doxorubicin deposition and therapeutic index in Vx2 tumors using MR-HIFU mild hyperthermia and thermosensitive liposomal doxorubicin. Int J Hyperthermia. 2019;36:196–203.
- Chu W, Staruch RM, Pichardo S, et al. Magnetic resonance-guided high-intensity focused ultrasound hyperthermia for recurrent rectal cancer: MR thermometry evaluation and preclinical validation. Int J Radiat Oncol Biol Phys. 2016;95:1259–1267.
- Partanen A, Tillander M, Yarmolenko PS, et al. Reduction of peak acoustic pressure and shaping of heated region by use of multifoci sonications in MR-guided high-intensity focused ultrasound mediated mild hyperthermia. Med Phys. 2013;40:013301.
- Yarmolenko PS, Eranki A, Partanen A, et al. Technical aspects of osteoid osteoma ablation in children using MR-guided high intensity focussed ultrasound. Int J Hyperthermia. 2018;34:49–58.
- Alkins RD, Mainprize TG. High-intensity focused ultrasound ablation therapy of gliomas. Prog Neurol Surg. 2018;32:39–47.
- Izadifar Z, Izadifar Z, Chapman D, et al. An introduction to high intensity focused ultrasound: systematic review on principles, devices, and clinical applications. J Clin Med. 2020;9:460.
- Jiang CL, Davalos RV, Bischof JC. A review of basic to clinical studies of irreversible electroporation therapy. IEEE Trans Biomed Eng. 2015;62:4–20.
- Davalos RV, Mir IL, Rubinsky B. Tissue ablation with irreversible electroporation. Ann Biomed Eng. 2005;33:223–231.
- Dunki-Jacobs EM, Philips P, Martin Ii RCG. Evaluation of thermal injury to liver, pancreas and kidney during irreversible electroporation in an in vivo experimental model. Br J Surg. 2014;101:1113–1121.
- van Gemert MJC, Wagstaff PGK, de Bruin DM, et al. Irreversible electroporation: just another form of thermal therapy? Prostate. 2015;75:332–335.
- van den Bos W, Scheffer HJ, Vogel JA, et al. Thermal energy during irreversible electroporation and the influence of different ablation parameters. J Vasc Interv Radiol. 2016;27:433–443.
- Faroja M, Ahmed M, Appelbaum L, et al. Irreversible electroporation ablation: is all the damage nonthermal? Radiology. 2013;266:462–470.
- Wagstaff PG, de Bruin DM, van den Bos W, et al. Irreversible electroporation of the porcine kidney: temperature development and distribution. Urol Oncol. 2015;33:168.e1–7.
- Agnass P, Van Veldhuisen E, Van Gemert MJ, et al. Mathematical modeling of the thermal effects of irreversible electroporation for in vitro, in vivo and clinical use: a systematic review. Int J Hyperthermia. 2020:37(1):486–505.
- Agnass P, van Veldhuisen E, Vogel JA, et al. Thermodynamic profiling during irreversible electroporation in porcine liver and pancreas: a case study series. J Clin Transl Res. 2020;5:4.
- Garcia PA, Davalos RV, Miklavcic D. A numerical investigation of the electric and thermal cell kill distributions in electroporation-based therapies in tissue. Plos One. 2014;9:e103083.
- Byron CR, DeWitt MR, Latouche EL, et al. Treatment of infiltrative superficial tumors in awake standing horses using novel high-frequency pulsed electrical fields. Front Vet Sci. 2019;6:265.
- Martin RC, 2nd, Durham AN, Besselink MG, et al. Irreversible electroporation in locally advanced pancreatic cancer: a call for standardization of energy delivery. J Surg Oncol. 2016;114:865–871.
- Brezovich IA, Atkinson WJ, Mb L. Local hyperthermia with interstitial techniques. Cancer Res. 1984;44:4752–4756.
- Brezovich IA, Lilly MB, Meredith RF, et al. Hyperthermia of pet animal tumours with self-regulating ferromagnetic thermoseeds. Int J Hyperthermia. 1990;6:117–130.
- van Wieringen N, van Dijk JD, van Veldhuizen J, et al. The effect of catheters and coatings on the performance of palladium-nickel thermoseeds: evaluation and design of implantation techniques. Int J Hyperthermia. 1997;13:187–204.
- Kandala SK, Liapi E, Whitcomb LL, et al. Temperature-controlled power modulation compensates for heterogeneous nanoparticle distributions: a computational optimization analysis for magnetic hyperthermia. Int J Hyperthermia. 2019;36:115–129.
- Mack CF, Stea B, Kittelson JM, et al. Interstitial thermoradiotherapy with ferromagnetic implants for locally advanced and recurrent neoplasms. Int J Radiat Oncol Biol Phys. 1993;27:109–115.
- Stauffer PR, van Rhoon GC. Overview of bladder heating technology: matching capabilities with clinical requirements. Int J Hyperthermia. 2016;32:407–416.
- Colombo R, Lev A, Da Pozzo LF, et al. A new approach using local combined microwave hyperthermia and chemotherapy in superficial transitional bladder-carcinoma treatment. J Urol. 1995;153:959–963.
- Rath-Wolfson L, Moskovitz B, Dekel Y, et al. Combined intravesical hyperthermia and mitomycin chemotherapy: a preliminary in vivo study. Int J Exp Pathol. 2003;84:145–152.
- Schooneveldt G, Bakker A, Balidemaj E, et al. Thermal dosimetry for bladder hyperthermia treatment. An overview. Int J Hyperthermia. 2016;32:417–433.
- Van Rhoon GC. External electromagnetic methods and devices. In: Moros EG, Hendee WR, editors. Physics of thermal therapy: fundamentals and clinical applications. Boca Raton (FL): CRC Press, Taylor & Francis Group; 2013. p. 139–158.
- Trefna HD, Crezee J, Schmidt M, et al. Quality assurance guidelines for superficial hyperthermia clinical trials: II. Technical requirements for heating devices. Strahlentherapie Und Onkologie. 2017;193:351–366.
- Dressel S, Gosselin MC, Capstick MH, et al. C, Novel hyperthermia applicator system allows adaptive treatment planning: preliminary clinical results in tumour-bearing animals. Vet Comp Oncol. 2018;16:202–213.
- Van der Gaag ML, de Bruijne M, Samaras T, et al. Development of a guideline for the water bolus temperature in superficial hyperthermia. Int J Hyperthermia. 2006;22:637–656.
- Arunachalam K, Maccarini PF, Craciunescu OI, et al. Thermal characteristics of thermobrachytherapy surface applicators for treating chest wall recurrence. Phys Med Biol. 2010;55:1949–1969.
- Kok HP, Groen J, Bakker A, et al. Modelling curved contact flexible microstrip applicators for patient-specific superficial hyperthermia treatment planning. Cancers (Basel). 2020;12:656.
- Lindholm CE, Kjellen E, Nilsson P, et al. Prognostic factors for tumour response and skin damage to combined radiotherapy and hyperthermia in superficial recurrent breast carcinomas. Int J Hyperthermia. 1995;11:337–355.
- Stauffer PR, Maccarini P, Arunachalam K, et al. Conformal microwave array (CMA) applicators for hyperthermia of diffuse chest wall recurrence. Int J Hyperthermia. 2010;26:686–698.
- Lee WM, Gelvich EA, van der Baan P, et al. Assessment of the performance characteristics of a prototype 12-element capacitive contact flexible microstrip applicator (CFMA-12) for superficial hyperthermia. Int J Hyperthermia. 2004;20:607–624.
- Rietveld PJ, van Putten WL, Van der Zee J, et al. Comparison of the clinical effectiveness of the 433 MHz Lucite cone applicator with that of a conventional waveguide applicator in applications of superficial hyperthermia. Int J Radiat Oncol Biol Phys. 1999;43:681–687.
- van Rhoon GC, Rietveld PJ, van der Zee J. A 433 MHz Lucite cone waveguide applicator for superficial hyperthermia. Int J Hyperthermia. 1998;14:13–27.
- Kosterev VV, Kramer-Ageev EA, Mazokhin VN, et al. Development of a novel method to enhance the therapeutic effect on tumours by simultaneous action of radiation and heating. Int J Hyperthermia. 2015;31:443–452.
- Maccarini PF, Arunachalam K, Juang T, et al. Shaping and resizing of multifed slot radiators used in conformal microwave antenna arrays for hyperthermia treatment of large superficial diseases. Proceedings of the 2009 International Conference on Electromagnetics in Advanced Applications; Sep 14–18; Torino, Italy. 2009.
- Johnson JE, Neuman DG, Maccarini PF, et al. Evaluation of a dual-arm Archimedean spiral array for microwave hyperthermia. Int J Hyperthermia. 2006;22:475–490.
- Lee ER, Wilsey TR, Tarczy-Hornoch P, et al. Body conformable 915 MHz microstrip array applicators for large surface area hyperthermia. IEEE Trans Biomed Eng. 1992;39:470–483.
- Gelvich EA, Mazokhin VN. Contact flexible microstrip applicators (CFMA) in a range from microwaves up to short waves. IEEE Trans Biomed Eng. 2002;49:1015–1023.
- Lamaitre G, Van Dijk JDP, Gelvich EA, et al. SAR characteristics of three types of Contact Flexible Microstrip Applicators for superficial hyperthermia. Int J Hyperthermia. 1996;12:255–269.
- Kok HP, Correia D, De Greef M, et al. SAR deposition by curved CFMA-434 applicators for superficial hyperthermia: Measurements and simulations. Int.J.Hyperthermia. 2010;26:171–184.
- Gelvich EA, Mazokhin VN. Resonance effects in applicator water boluses and their influence on SAR distribution patterns. Int J Hyperthermia. 2000;16:113–128.
- Gelvich EA, Klimanov VA, Kramer-Ageev EA, et al. Computational evaluation of changes in ionizing radiation dose distribution in tissues caused by EM applicators when external radiation and hyperthermia act simultaneously. Int.J.Hyperthermia. 2006;22:343–352.
- Overgaard J. Simultaneous and sequential hyperthermia and radiation treatment of an experimental tumor and its surrounding normal tissue in vivo. Int J Radiat Oncol Biol Phys. 1980;6:1507–1517.
- Wust P, Ghadjar P, Nadobny J, et al. Physical analysis of temperature-dependent effects of amplitude-modulated electromagnetic hyperthermia. Int J Hyperthermia. 2019;36:1246–1254.
- Curley SA, Palalon F, Lu X, et al. Noninvasive radiofrequency treatment effect on mitochondria in pancreatic cancer cells. Cancer. 2014;120:3418–3425.
- Curley SA, Palalon F, Sanders KE, et al. The effects of non-invasive radiofrequency treatment and hyperthermia on malignant and nonmalignant cells. Int J Environ Res Public Health. 2014;11:9142–9153.
- Ware MJ, Tinger S, Colbert KL, et al. Radiofrequency treatment alters cancer cell phenotype. Sci Rep. 2015;5:12083.
- Van Wieringen N, Wiersma J, Zum Vörde Sive Vörding PJ, et al. Characteristics and performance evaluation of the capacitive Contact Flexible Microstrip Applicator operating at 70 MHz for external hyperthermia. Int J Hyperthermia. 2009;25:542–553.
- Wu L, McGough RJ, Arabe OA, et al. An RF phased array applicator designed for hyperthermia breast cancer treatments. Phys Med Biol. 2006;51:1–20.
- Paulides MM, Bakker JF, Neufeld E, et al. Winner of the “New Investigator Award” at the European Society of Hyperthermia Oncology Meeting 2007. The HYPERcollar: a novel applicator for hyperthermia in the head and neck. Int J Hyperthermia. 2007;23:567–576.
- Paulides MM, Bakker JF, Hofstetter LW, et al. Laboratory prototype for experimental validation of MR-guided radiofrequency head and neck hyperthermia. Phys Med Biol. 2014;59:2139–2154.
- Corry PM, Barlogie B, Tilchen EJ, et al. Ultrasound-induced hyperthermia for the treatment of human superficial tumors. Int J Radiat Oncol Biol Phys. 1982;8:1225–1229.
- Underwood HR, Burdette EC, Ocheltree KB, et al. A multi-element ultrasonic hyperthermia applicator with independent element control. Int J Hyperthermia. 1987;3:257–267.
- Moros EG, Fan X, Straube WL. Experimental assessment of power and temperature penetration depth control with a dual frequency ultrasonic system. Med Phys. 1999;26:810–817.
- Novak P, Moros EG, Straube WL, et al. SURLAS: a new clinical grade ultrasound system for sequential or concomitant thermoradiotherapy of superficial tumors: applicator description. Med Phys. 2005;32:230–240.
- Van Leeuwen CM, Oei AL, Chin K, et al. A short time interval between radiotherapy and hyperthermia reduces in-field recurrence and mortality in women with advanced cervical cancer. Radiat Oncol. 2017;12:75.
- van Leeuwen CM, Crezee J, Oei AL, et al. The effect of time interval between radiotherapy and hyperthermia on planned equivalent radiation dose. Int J Hyperthermia. 2018;34:901–909.
- Kato H, Hyodo K, Akasaka N, et al. Optimization of bolus for capacitive type heating. Jpn J Hyperthermic Oncol. 1997;13:10–17.
- Paulsen KD, Geimer S, Tang J, et al. Optimization of pelvic heating rate distributions with electromagnetic phased arrays. Int J Hyperthermia. 1999;15:157–186.
- Wust P, Seebass M, Nadobny J, et al. Simulation studies promote technological development of radiofrequency phased array hyperthermia. Int.J.Hyperthermia. 1996;12:477–494.
- Kok HP, De Greef M, Borsboom PP, et al. Improved power steering with double and triple ring waveguide systems: the impact of the operating frequency. Int J Hyperthermia. 2011;27:224–239.
- Turner PF, Tumeh A, Schaefermeyer T, et al. approach for deep local and regional hyperthermia: physics and technology. Strahlenther Onkol. 1989;165:738–741.
- Zweije R, Kok HP, Bakker A, et al. Technical and clinical evaluation of the ALBA-4D 70MHz loco-regional hyperthermia system. Proceedings of the 48th European Microwave Conference. 2018. p. 328–331.
- Gellermann J, Wust P, Stalling D, et al. Clinical evaluation and verification of the hyperthermia treatment planning system hyperplan. Int J Radiat Oncol Biol Phys. 2000;47:1145–1156.
- Sreenivasa G, Gellermann J, Rau B, et al. Clinical use of the hyperthermia treatment planning system HyperPlan to predict effectiveness and toxicity. Int J Radiat Oncol Biol Phys. 2003;55:407–419.
- Van Haaren PMA, Kok HP, Van den Berg CAT, et al. On verification of hyperthermia treatment planning for cervical carcinoma patients. Int J Hyperthermia. 2007;23:303–314.
- Van Haaren P, Kok P, Van Stam G, et al. SAR measurements and FDTD calculations in inhomogeneous phantom models. Proceedings of the 9th International Congress on Hyperthermic Oncology; 2004; St.Louis, MA. Abstracts. 2004. p. 167.
- Wust P, Beck R, Berger J, et al. Electric field distributions in a phased-array applicator with 12 channels: measurements and numerical simulations. Med Phys. 2000;27:2565–2579.
- Wust P, Fahling H, Wlodarczyk W, et al. Antenna arrays in the SIGMA-eye applicator: interactions and transforming networks. Med Phys. 2001;28:1793–1805.
- Weihrauch M, Wust P, Weiser M, et al. Adaptation of antenna profiles for control of MR guided hyperthermia (HT) in a hybrid MR-HT system. Med Phys. 2007;34:4717–4725.
- Gellermann J, Hildebrandt B, Issels R, et al. Noninvasive magnetic resonance thermography of soft tissue sarcomas during regional hyperthermia: correlation with response and direct thermometry. Cancer. 2006;107:1373–1382.
- Gellermann J, Wlodarczyk W, Hildebrandt B, et al. Noninvasive magnetic resonance thermography of recurrent rectal carcinoma in a 1.5 Tesla hybrid system. Cancer Res. 2005;65:5872–5880.
- Gellermann J, Wlodarczyk W, Ganter H, et al. A practical approach to thermography in a hyperthermia/magnetic resonance hybrid system: validation in a heterogeneous phantom. Int J Radiat Oncol Biol Phys. 2005;61:267–277.
- Mulder HT, Curto S, Paulides MM, et al. Systematic quality assurance of the BSD2000-3D MR-compatible hyperthermia applicator performance using MR temperature imaging. Int J Hyperthermia. 2018;35:305–313.
- van Dijk JDP, Schneider CJ, van Os RM, et al. Results of deep body hyperthermia with large waveguide radiators. Adv Exp Med Biol. 1990;267:315–319.
- Crezee J, Van Haaren PMA, Westendorp H, et al. Improving locoregional hyperthermia delivery using the 3-D controlled AMC-8 phased array hyperthermia system: a preclinical study. Int J Hyperthermia. 2009;25:581–592.
- Ott OJ, Schmidt M, Semrau S, et al. Chemoradiotherapy with and without deep regional hyperthermia for squamous cell carcinoma of the anus. Strahlenther Onkol. 2019;195:607–614.
- Datta NR, Pestalozzi B, Clavien PA, et al.; members of the HEATPAC Trial Group. “HEATPAC” – a phase II randomized study of concurrent thermochemoradiotherapy versus chemoradiotherapy alone in locally advanced pancreatic cancer. Radiat Oncol. 2017;12:183.
- Gani C, Bonomo P, Zwirner K, et al. Organ preservation in rectal cancer – challenges and future strategies. Clin Transl Radiat Oncol. 2017;3:9–15.
- Carlier C, Mathys A, De Jaeghere E, et al. Tumour tissue transport after intraperitoneal anticancer drug delivery. Int J Hyperthermia. 2017;33:534–542.
- Klaver CEL, Musters GD, Bemelman WA, et al. Adjuvant hyperthermic intraperitoneal chemotherapy (HIPEC) in patients with colon cancer at high risk of peritoneal carcinomatosis; the COLOPEC randomized multicentre trial. BMC Cancer. 2015;15:428.
- Sugarbaker PH. Strategies to improve local control of resected pancreas adenocarcinoma. Surg Oncol. 2017;26:63–70.
- Klaver CEL, Wisselink DD, Punt CJA, et al.; COLOPEC collaborators group. Adjuvant hyperthermic intraperitoneal chemotherapy in patients with locally advanced colon cancer (COLOPEC): a multicentre, open-label, randomised trial. Lancet Gastroenterol Hepatol. 2019;4:761–770.
- Goere D, Glehen O, Quenet F, et al.; BIG-RENAPE and PRODIGE. Results of a randomized phase 3 study evaluating the potential benefit of a second-look surgery plus HIPEC in patients at high risk of developing colorectal peritoneal metastases (PROPHYLOCHIP-NTC01226394). J Clin Oncol. 2018;36:3531–3531.
- Ceelen W. HIPEC with oxaliplatin for colorectal peritoneal metastasis: the end of the road? Eur J Surg Oncol. 2019;45:400–402.
- Rau B, Brandl A, Piso P, et al.; Peritoneum Surface Oncology Group and members of the StuDoQ|Peritoneum Registry of the German Society for General and Visceral Surgery (DGAV). Peritoneal metastasis in gastric cancer: results from the German database. Gastric Cancer. 2019;23:11–22.
- Bull JM, Scott GL, Strebel FR, et al. Fever-range whole-body thermal therapy combined with cisplatin, gemcitabine, and daily interferon-alpha: a description of a phase I-II protocol. Int J Hyperthermia. 2008;24:649–662.
- Hildebrandt B, Hegewisch-Becker S, Kerner T, et al. Current status of radiant whole-body hyperthermia at temperatures > 41.5 degrees C and practical guidelines for the treatment of adults. The German ‘Interdisciplinary Working Group on Hyperthermia. Int J Hyperthermia. 2005;21:169–183.
- Janssen CW, Lowry CA, Mehl MR, et al. Whole-body hyperthermia for the treatment of major depressive disorder: a randomized clinical trial. JAMA Psychiatry. 2016;73:789–795.
- Song CW. Effect of local hyperthermia on blood flow and microenvironment: a review. Cancer Res. 1984;44:4721s–4730s.
- Franckena M, Fatehi D, de Bruijne M, et al. Hyperthermia dose-effect relationship in 420 patients with cervical cancer treated with combined radiotherapy and hyperthermia. Eur J Cancer. 2009;45:1969–1978.
- Linthorst M, Baaijens M, Wiggenraad R, et al. Local control rate after the combination of re-irradiation and hyperthermia for irresectable recurrent breast cancer: Results in 248 patients. Radiother Oncol. 2015;117:217–222.
- Bakker A, Kolff MW, Holman R, et al. Thermal skin damage during reirradiation and hyperthermia is time-temperature dependent. Int J Radiat Oncol Biol Phys. 2017;98:392–399.
- Saccomandi P, Schena E, Silvestri S. Techniques for temperature monitoring during laser-induced thermotherapy: An overview. Int J Hyperthermia. 2013;29:609–619.
- Lewis MA, Staruch RM, Chopra R. Thermometry and ablation monitoring with ultrasound. Int J Hyperthermia. 2015;31:163–181.
- De Leeuw AAC, Crezee J, Lagendijk JJW. Temperature and SAR measurements in deep-body hyperthermia with thermocouple thermometry. Int J Hyperthermia. 1993;9:685–697.
- Hynynen K, Edwards DK. Temperature measurements during ultrasound hyperthermia. Med Phys. 1989;16:618–626.
- Morris H, Rivens I, Shaw A, et al. Investigation of the viscous heating artefact arising from the use of thermocouples in a focused ultrasound field. Phys Med Biol. 2008;53:4759–4776.
- Winter L, Oberacker E, Paul K, et al. Magnetic resonance thermometry: Methodology, pitfalls and practical solutions. Int J Hyperthermia. 2016;32:63–75.
- Fani F, Schena E, Saccomandi P, et al. CT-based thermometry: an overview. Int J Hyperthermia. 2014;30:219–227.
- Miyakawa M, Bolomey JC. Non-invasive thermometry of the human body. Boca Raton (FL): CRC Press; 1995.
- Bharat S, Techavipoo U, Kiss MZ, et al. Monitoring stiffness changes in lesions after radiofrequency ablation at different temperatures and durations of ablation. Ultrasound Med Biol. 2005;31:415–422.
- Ishihara Y, Calderon A, Watanabe H, et al. A precise and fast temperature mapping using water proton chemical shift. Magn Reson Med. 1995;34:814–823.
- Kothapalli SV, Altman MB, Zhu L, et al. Evaluation and selection of anatomic sites for magnetic resonance imaging-guided mild hyperthermia therapy: a healthy volunteer study. Int J Hyperthermia. 2018;34:1381–1389.
- Li Z, Vogel M, Maccarini PF, et al. Improved hyperthermia treatment control using SAR/temperature simulation and PRFS magnetic resonance thermal imaging. Int J Hyperthermia. 2011;27:86–99.
- Salomir R, Palussière, J, Vimeux FC, et al. Local hyperthermia with MR-guided focused ultrasound: spiral trajectory of the focal point optimized for temperature uniformity in the target region. J Magn Reson Imaging. 2000;12:571–583.
- Kim YS. Advances in MR image-guided high-intensity focused ultrasound therapy. Int J Hyperthermia. 2015;31:225–232.
- Siedek F, Yeo SY, Heijman E, et al. Magnetic resonance-guided high-intensity focused ultrasound (MR-HIFU): technical background and overview of current clinical applications (part 1). Rofo. 2019;191:522–530.
- Siedek F, Yeo SY, Heijman E, et al. Magnetic resonance-guided high-intensity focused ultrasound (MR-HIFU): overview of emerging applications (part 2). Rofo. 2019;191:531–539.
- Cline HE, Schenck JF, Hynynen K, et al. MR-guided focused ultrasound surgery. J Comput Assist Tomogr. 1992;16:956–965.
- Sanghvi NT, Chen WH, Carlson R, et al. Clinical validation of real-time tissue change monitoring during prostate tissue ablation with high intensity focused ultrasound. J Ther Ultrasound. 2017;5:24
- Pichardo S, Gelet A, Curiel L, et al. New integrated imaging high intensity focused ultrasound probe for transrectal prostate cancer treatment. Ultrasound Med Biol. 2008;34:1105–1116.
- Shahmirzadi D, Hou GY, Chen J, et al. Ex Vivo characterization of canine liver tissue viscoelasticity after high-intensity focused ultrasound ablation . Ultrasound Med Biol. 2014;40:341–350.
- Kok HP, Wust P, Stauffer PR, et al. Current state of the art of regional hyperthermia treatment planning: a review. Radiat Oncol. 2015;10:196
- De Greef M, Kok HP, Correia D, et al. Optimization in hyperthermia treatment planning: the impact of tissue perfusion uncertainty. Med Phys. 2010;37:4540–4550.
- De Greef M, Kok HP, Correia D, et al. Uncertainty in hyperthermia treatment planning: the need for robust system design. Phys Med Biol. 2011;56:3233–3250.
- van de Kamer JB, Van Wieringen N, De Leeuw AAC, et al. The significance of accurate dielectric tissue data for hyperthermia treatment planning. Int J Hyperthermia. 2001;17:123–142.
- Edd JF, Davalos RV. Mathematical modeling of irreversible electroporation for treatment planning. Technol Cancer Res Treat. 2007;6:275–286.
- Neal RE, Garcia PA, Robertson JL, et al. Experimental characterization and numerical modeling of tissue electrical conductivity during pulsed electric fields for irreversible electroporation treatment planning. IEEE Trans Biomed Eng. 2012;59:1076–1085.
- Porcheron J, Talabard JN, Breton C, et al. Intraperitoneal chemohyperthermia for peritoneal carcinomatosis: original modeling, clinical tolerance and results study about 30 patients. Hepatogastroenterology. 2000;47:1411–1418.
- Ladhari T, Szafnicki K. Modelling of some aspects of a biomedical process: application to the treatment of digestive cancer (HIPEC). 3 Biotech. 2018;8:190.
- Stigliano RV, Shubitidze F, Petryk AA, et al. Magnetic nanoparticle hyperthermia: predictive model for temperature distribution. Proc SPIE Int Soc Opt Eng. 2013;8584:858410.
- Berjano EJ. Theoretical modeling for radiofrequency ablation: state-of-the-art and challenges for the future. Biomed Eng Online. 2006;5:24.
- Schumann C, Rieder C, Haase S, et al. Interactive multi-criteria planning for radiofrequency ablation. Int J Comput Assist Radiol Surg. 2015;10:879–889.
- Reinhardt M, Brandmaier P, Seider D, et al.; ClinicIMPPACT Study Group. A prospective development study of software-guided radio-frequency ablation of primary and secondary liver tumors: clinical intervention modelling, planning and proof for ablation cancer treatment (ClinicIMPPACT). Contemp Clin Trials Commun. 2017;8:25–32.
- Lopresto V, Pinto R, Farina L, et al. Treatment planning in microwave thermal ablation: clinical gaps and recent research advances. Int J Hyperthermia. 2016;:1–39.
- Yero DD, Gonzalez FG, Van Troyen D, et al. Dielectric properties of ex vivo porcine liver tissue characterized at frequencies between 5 and 500 kHz when heated at different rates. IEEE Trans Biomed Eng. 2018;65:2560–2568.
- Sebek J, Albin N, Bortel R, et al. Sensitivity of microwave ablation models to tissue biophysical properties: A first step toward probabilistic modeling and treatment planning. Med Phys. 2016;43:2649.
- Chen D, Xia R, Chen X, et al. SonoKnife: feasibility of a line-focused ultrasound device for thermal ablation therapy. Med Phys. 2011;38:4372–4385.
- Nikolov SI, Jensen JA. Application of different spatial sampling patterns for sparse array transducer design. Ultrasonics. 2000;37:667–671.
- de Bruijne M, Wielheesen DH, Van der Zee J, et al. Benefits of superficial hyperthermia treatment planning: five case studies. Int J Hyperthermia. 2007;23:417–429.
- Trujillo-Romero CJ, Paulides MM, Drizdal T, et al. Impact of silicone and metal port-a-cath implants on superficial hyperthermia treatment quality. Int J Hyperthermia. 2015;31:15–22.
- Franckena M, Canters R, Termorshuizen F, et al. Clinical implementation of hyperthermia treatment planning guided steering: A cross over trial to assess its current contribution to treatment quality. Int J Hyperthermia. 2010;26:145–157.
- Kok HP, Ciampa S, De Kroon-Oldenhof R, et al. Toward on-line adaptive hyperthermia treatment planning: correlation between measured and simulated specific absorption rate changes caused by phase steering in patients. Int.J.Radiat.Oncol.Biol.Phys. 2014;90:438–445.
- Kok HP, Schooneveldt G, Bakker A, et al. Predictive value of simulated SAR and temperature for changes in measured temperature after phase-amplitude steering during locoregional hyperthermia treatments. Int J Hyperthermia. 2018;35:330–339.
- Kok HP, Korshuize-van Straten L, Bakker A, et al. Feasibility of on-line temperature-based hyperthermia treatment planning to improve tumour temperatures during locoregional hyperthermia. Int J Hyperthermia. 2018;34:1082–1091.
- Rijnen Z, Bakker JF, Canters RA, et al. Clinical integration of software tool VEDO for adaptive and quantitative application of phased array hyperthermia in the head and neck. Int J Hyperthermia. 2013;29:181–193.
- Kok HP, Crezee J, Franken NAP, et al. Quantifying the combined effect of radiation therapy and hyperthermia in terms of equivalent dose distributions. Int J Radiat Oncol Biol Phys. 2014;88:739–745.
- Crezee J, van Leeuwen CM, Oei AL, et al. Biological modelling of the radiation dose escalation effect of regional hyperthermia in cervical cancer. Radiat Oncol. 2016;11:14
- Kok HP, Van Dijk I, Crama KF, et al. Re-irradiation plus hyperthermia for recurrent pediatric sarcoma; a simulation study to investigate feasibility. Int J Oncol. 2019;54:209–218.
- Crezee H, van Leeuwen CM, Oei AL, et al. Thermoradiotherapy planning: Integration in routine clinical practice. Int J Hyperthermia. 2016;32:41–49.
- Schooneveldt G, Dobsicek Trefna H, Persson M, et al. Hyperthermia treatment planning including convective flow in cerebrospinal fluid for brain tumour hyperthermia treatment using a novel dedicated paediatric brain applicator. Cancers (Basel. 2019;11:1183.
- Coluccia D, Fandino J, Schwyzer L, et al. First noninvasive thermal ablation of a brain tumor with MR-guided focused ultrasound. J Ther Ultrasound. 2014;2:17.
- Kok HP, Beck M, Loke DR, et al. Locoregional peritoneal hyperthermia to enhance the effectiveness of chemotherapy in patients with peritoneal carcinomatosis: a simulation study comparing different locoregional heating systems. Int J Hyperthermia. 2020;37:76–88.
- Hoopes PJ, Wagner RJ, Duval K, et al. Treatment of canine oral melanoma with nanotechnology-based immunotherapy and radiation. Mol Pharm. 2018;15:3717–3722.
- Chao Y, Chen G, Liang C, et al. Iron nanoparticles for low-power local magnetic hyperthermia in combination with immune checkpoint blockade for systemic antitumor therapy. Nano Lett. 2019;19:4287–4296.
- Oei AL, Korangath P, Mulka K, et al. Enhancing the abscopal effect of radiation and immune checkpoint inhibitor therapies with magnetic nanoparticle hyperthermia in a model of metastatic breast cancer. Int J Hyperthermia. 2019;36:47–63.
- Duval KEA, Vernice NA, Wagner RJ, et al. Immunogenetic effects of low dose (CEM43 30) magnetic nanoparticle hyperthermia and radiation in melanoma cells. Int J Hyperthermia. 2019;36:37–46.