Abstract
Immunotherapy to treat cancer is now an established clinical approach. Immunotherapy can be applied systemically, as done with checkpoint blockade antibodies, but it can also be injected directly into identified tumors, in a strategy of in situ vaccination (ISV). ISV is designed to stimulate a strong local antitumor immune response involving both innate and adaptive immune cells, and through this generate a systemic antitumor immune response against metastatic tumors. A variety of ISVs have been utilized to generate an immunostimulatory tumor microenvironment (TME). These include attenuated microorganisms, recombinant proteins, small molecules, physical disruptors of TME (alternating magnetic and focused ultrasound heating, photothermal therapy, and radiotherapy), and more recently nanoparticles (NPs). NPs are attractive and unique since they can load multiple drugs or other reagents to influence immune and cancer cell functions in the TME, affording a unique opportunity to stimulate antitumor immunity. Here, we describe the NP-ISV therapeutic mechanisms, review chemically synthesized NPs (i.e., liposomes, polymeric, chitosan-based, inorganic NPs, etc.), biologically derived NPs (virus and bacteria-based NPs), and energy-activated NP-ISVs in the context of their use as local ISV. Data suggests that NP-ISVs can enhance outcomes of immunotherapeutic regimens including those utilizing tumor hyperthermia and checkpoint blockade therapies.
1. Introduction
Clinically detectable tumors typically generate an immune-suppressive tumor microenvironment (TME) that enables them to evade immune surveillance [Citation1–3]. To address this, immunotherapy agents that reverse or circumvent immune suppression and stimulate immune cell activation against tumor cells are increasingly being utilized clinically [Citation4,Citation5]. Currently, this is most frequently attempted using immunotherapies that block the negative signaling between innate immune cells or tumor cells, and T cells, using ‘checkpoint blockade’ antibodies that disrupt suppressive signaling to T cells through anti-cytotoxic T-lymphocyte-associated protein (CTLA)-4, antiprogrammed cell death (PD)-1, and anti-PD-ligand (PD-L) monoclonal antibodies [Citation6,Citation7]. Other immunotherapy approaches include chimeric antigen receptor (CAR)-T cell therapy [Citation8–10] or T-VEC, a modified oncolytic herpes virus that expresses GM-CSF [Citation11,Citation12]. Although of value for a minority of patients, the success of immunotherapy approaches are often dependent on the baseline tumor-recognizing innate and T cells [Citation13]. One approach to achieve an immune stimulatory TME can be by in situ vaccination (ISV). ISV treatments are administered locally in solid tumors [Citation14]. This can be done using local application of immunostimulatory reagents or by physical treatments; all of which generate a robust local antitumor immune response and expand the population of tumor-recognizing effector T cells to systemically combat metastatic disease.
A variety of ISVs with enhanced efficacy and reduced systemic and autoimmune toxicities have been reported [Citation15–21]. In particular, the use of nanoparticle-(NP)-ISV agents is highly appealing. NP’s tunability of size, shape, surface charge, and biocompatibility along with their high surface area-to-volume ratio and physical properties (e.g., photothermal, magnetic, etc.) provide unique opportunities for the alteration of the TME. Therapeutically, NPs can directly stimulate or release encapsulated immunostimulatory molecules within TME to modulate tumor antigen presentation and generate tumor-recognizing effector T cells. NP-ISVs can also be incorporated in combinatorial immunotherapeutic regimens against a variety of tumor types to improve outcomes. Here, we review ISV usage of both chemically synthesized NPs (organic and inorganic), biological agents that are NPs by size or have physio-chemical properties of NPs, including viral- and bacterial-based NPs, and device (focused ultrasound, photothermal, and magnetic) directed NP-ISVs for cancer immunotherapy. The relevant classes and the subclasses of NPs and the treatment approaches are illustrated in .
Figure 1. NP-ISV classes and therapeutic approaches. NP-ISVs are chemically synthesized or derived from biological agents. NP-ISVs can be utilized for multiple functions such as antigen capture, immune adjuvants, gene and drug delivery, immune cell targeting, or amplified via external devices to induce local and systemic anti-tumor immunity.
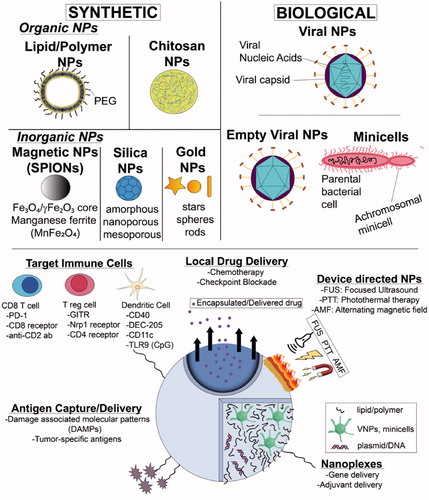
2. NP-ISV background, principles, and challenges
NPs are variably defined, but typically are below 100 nm in at least one dimension, and have various shapes (e.g., spheres, rods, stars, triangles, bars, prisms, icosahedrals, dodecahedrons etc.). NPs have been found to be adept at loading/encapsulating drug molecules and deliver them in a sustained manner to solid tumors following intravenous injections using the enhanced permeability and retention (EPR) effect [Citation22,Citation23]. In fact, EPR methodology is the primary method for enhancing tumor delivery and therapeutic effects of FDA-approved Doxil® [Citation24]. In contrast to EPR-based targeting, NP-ISVs relies on their direct injection in the TME, and is described below.
TME is composed of both tumor and non-tumor cells [fibroblasts, tumor-associated macrophages (TAMs), dendritic, and T-cells] and extracellular materials [Citation25]. Of these, TAMs can constitute ∼50% of the total tumoral cellular population in TME [Citation26]. TAMs can be of antitumoral (M1 phenotype) or protumoral (M2 phenotype). TAMs with M2-like phenotype secrete immunosuppressive cytokines like IL-23, IL-17, IL-6 to cause metastasis and promote cancer stem cells [Citation27–29]. Thus, reducing the populations of M2 macrophages should be the key goal of NP-ISVs. Approaches to address these can be illustrated by the targeting of NP-ISVs to individual immune cells (i.e., CD8+-targeting NPs via anti-PD-1 [30], conjugated anti-CD8 antibodies [Citation30] or conjugated anti-CD2 antibodies [Citation31]; Treg-targeting NPs via glucocorticoid-induced TNFR-related receptor (GITR) [Citation32,Citation33], Nrp1 receptor [Citation34] or conjugated anti-CD4 antibodies [Citation31]; DC-targeting NPs via CD40 [Citation35,Citation36], DEC-205 [Citation35,Citation37], and CD11c [35]). Alternatively, the NP-ISVs physicochemical properties can be altered to enhance the activity of immune and cancer cells in the TME.
The detailed review of how the NPs size, shape, surface, and charge influences the phagocytosis and inflammatory functions of innate immune cells (dendritic cells and macrophages) have been elegantly discussed by Liu et al. and others [Citation38,Citation39]. To summarize (), the innate cells endocytose smaller NPs (∼10–100 nm) via. the clathrin-mediated pathway. In contrast, micropinocytosis is the primary trafficking mechanism for uptake of larger particles (∼500nm). Along with size, the rate of NP phagocytosis is also dependent on its surface charge. In general, the anionic particles show minimal interactions with the negatively charged cell membrane and thus are anti-inflammatory, whereas the more cationic NPs demonstrate robust uptake to induce a pro-inflammatory phenotype in the macrophages [Citation40]. Both macrophages and DCs efficiently internalize the NPs, however, the antigen presentation can be influenced by the particle composition [Citation41]. In one study with amphiphilic poly(γ-glutamic acid) (γ-PGA) NPs containing variable levels of hydrophobic amino acid ethyl esters (AAE), the hydrophobic segments were found to discretely control the induction of cellular and humoral immunity by the dendritic cells [Citation42]. Similarly, Moyano et al. investigating gold NPs with various levels of surface hydrophobicities showed that the production of pro-inflammatory cytokines from the splenocytes was proportional to the hydrophobicity of the NPs in vitro and in vivo [Citation43]. Another aspect of NPs that determine their detection by the macrophages is the presence of protein corona on its surface. Corona is essentially the adsorption of biomolecules on the NP surface in circulation [Citation44,Citation45]. Corona formation on the NPs can be prevented to a large extent by surface functionalizations. For example, coating NPs with large and hydrophilic polyethylene glycol (PEG) can block the interactions with serum protein and resultant opsonization, preventing their rapid clearance by phagocytic cells [Citation46,Citation47]. Similarly, NPs with zwitterionic surfaces can provide resistance to protein adsorption [Citation48]. This said, a recent study reported that the NP surface composed of hydrophobic zwitterion functionality still induces an inflammatory phenotype in macrophages relative to the hydrophilic counterparts, underlying the important of surface composition [Citation49].
Table 1. Relationship of NP physicochemical properties with immune stimulation.
It may be noted that NPs were originally developed to reduce systemic toxicities of systemically administered chemotherapeutic agents. So, a majority of research utilizing NPs evaluated their immune effects following intravenous injection, or upon incubation with macrophages and dendritic cell in vitro. It is important to understand that the NP effects in TME when used as an ISV may not be similar to the ones that are observed following parenteral therapy. NP-ISVs do not encounter the serum proteins and corona formation that is typically seen with intravenous injection. NP-ISVs also make more intimate, direct, and concurrent interactions in TME with tumor and stromal cells, and thus can generate different immune-related cross-talks. Additionally, the pH of TME extracellular environment is slightly acidic [Citation50]. This may influence the NP-ISV physicochemical properties, release of encapsulated agents, and immune effects. Furthermore, the surface properties of NP-ISVs may induce dramatically different immune responses even at similar rates of endocytosis. Wu et al. developed glyco-NPs with different kinds of saccharides coronas (galactoside, mannoside, or mixed galactoside-mannoside (GM) copolymer). When incubated with the macrophages in vitro, the NPs with mixed corona (GM) showed stronger macrophage activations (as evidenced by the production of arginase and iNOS) compared to mannose or galactoside NPs alone [Citation51]. Finally, immunosuppressive untreated tumors can sometime antagonize the local antitumor immune response generated by ISVs including the NPs [Citation52]. Uncovering these fundamental mechanisms and principles that can control NP-ISV immune effects is key to optimizing the therapeutic outcomes in cancer patients.
3. Classes of NP-ISVs
NP-ISVs delivered by intratumoral injections increases tumor cell recognition via multiple mechanisms (). The NPs in TME can capture antigens [Citation53], induce polarization of TAMs/depletion, dendritic cell activation and maturation, and limit Tregs [Citation54–56]. Below we discuss the various classes of NP-ISVs, benefits, and drawbacks.
Figure 2. Proposed mechanisms and targets of NP-ISVs in the tumor microenvironment. Typically, the antigen presenting cells (APC) can be targeted with NP-ISVs to deliver antigens, or activate pattern recognition and toll-like receptors, and CD40s. Tumor cells can also be induced to generate DAMPs and release tumor antigents with energy depositing devices for NP-ISV antigen capture. The resultant APC activation and production of tumor recognizing T-cells that leave the draining lymph nodes can attack tumor cells anywhere in the patient. The activated T-cells can also synergize with CBTs to improve therapeutic outcomes.
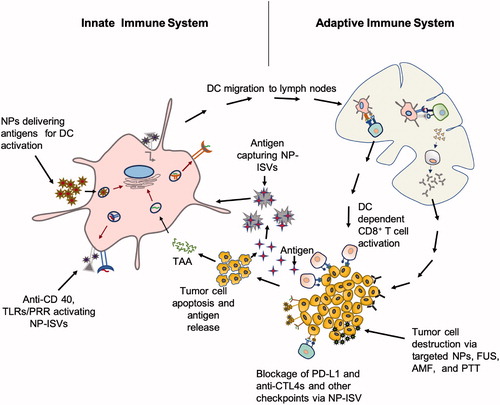
3.1. Chemically synthesized NP-ISVs
NPs are synthesized using organic, inorganic materials, or a mixture of both. Organic NPs are typically composed of molecules with a carbon backbone that could be totally synthetic or similar to those found commonly in biological systems, such as lipids. Examples of organic NPs include liposomes, which are made from phospholipid bilayers and contains an aqueous core, polymeric NPs, similarly made but from a block co-polymer and other materials (e.g., polymersomes), or chitosan-based NPs [Citation57]. In contrast, inorganic NPs are made up of noncarbon elements such as silicon, gold, iron etc. NPs can also be modified by doping organic and inorganic material or complexation with immunoadjuvant for enhancing clinical use.
3.1.1. Liposome based NP-ISV
Liposome vaccination against solid tumors via subcutaneous, intradermal, and intramuscular routes has been widely reported [Citation58–61]. For example, a continuous release liposomal formulation of interleukin-2 (IL-2) was synthesized by co-mixing hydrophobic lipids with IL-2. This formulation enhanced IL-2 residence time in systemic circulation by 8-fold via subcutaneous route compared to free IL-2 [Citation59]. Unlike injections at non-tumor sites (e.g., subcutaneous space), NP-ISVs are directly injected into the tumor. We showed recently the artificial generation of calreticulin (CRT) in murine melanoma tumors using cationic liposome based NP-ISV. CRT is a damage-associated molecular pattern (DAMP) commonly associated with immunogenic cell death (ICD). We found that the CRT-based liposomes were particularly immunostimulatory in combination with focused ultrasound (FUS) heating [Citation62]. Briefly, full-length cloned plasmid of calreticulin encapsulated in the cationic liposomes was applied sequentially after FUS heating in murine melanoma tumors. An enhanced expression of calreticulin and populations of melanoma specific CD4+ and CD8+ T cells with polarization to M1 phenotype was seen in the treated tumors. Importantly the CRT-NP/FUS treatment prevented tumor growth in the untreated distant sites, suggesting an abscopal effect. Meraz et al. developed an alternative NP-ISV approach by encapsulating monophosphoryl lipid A (MPL) and IL-12 in cationic liposomes to reduce systemic toxicity and achieve a more sustained local release of the cytokine [Citation63]. The team found that following intratumoral injection in the orthotopic model of murine breast cancer, MPL acted as a pathogen-associated molecular pattern (PAMP), and activated the pattern recognition receptor (PRR) in the dendritic cell via IRAK and MyD88 adaptors. When combined with IL-12, it synergistically enhanced the nitric oxide (NO) synthase (iNOS) expressions, resulting in high IL-2 and IFNγ expressions from T-cells, and a T helper (Th)-1 cell mediated local and systemic anti-tumor immunity. In contrast to cationic lipids that are toxic to cells, neutral lipids are relatively safer to use. Francian et al. performed intratumoral NP-ISV vaccination with ovalbumin-loaded neutral liposomes decorated with endogenous C3 serum proteins in a murine model of lymphoma [Citation64]. Data suggested an efficient targeting of the C3 receptors of macrophages and dendritic cells by the C3 proteins of liposomes. Additionally, the internalization and processing of ovalbumin by the APCs and subsequent T-cell activation achieved an efficient remission of ovalubumin expressing murine lymphoma tumors. A drawback of neutral liposome is their propensity to rapidly redistribute in the venous outflow [Citation65]. In contrast, cationic liposome binds strongly with the negative cell membrane when used as an ISV. The tumor retention can also be aided by increasing the size of lipidic carriers. Typically, large lipidic emulsions (>200nm) are efficiently retained in the tumors. Further, Harrington et al. reported that PEGylated liposomes retain the encapsulated content in the tumor (∼12-fold) compared to free form upon local injection [Citation66]. PEGylated liposomes can also target the draining lymph nodes following local administration, and thus they can potentially improve the antigen presentations in the lymph nodes.
Other liposome based NP-ISV approaches that have shown promise include the encapsulation of hydrophilic and negatively charged STING agonists (cGAMP) in its aqueous core. STING is an intracellular signaling protein in DCs that senses 2’3′-cyclic GMP-AMP (cGAMP), especially during the interactions of dying tumor cells DNA with cyclic GMP-AMP synthase (cGAS) [Citation67]. Since the free form of cGAMP is negatively charged, it does not enter the APCs efficiently as an ISV [Citation68]. Liu et al. loaded cGAMP into the phosphatidylserine coated liposome for targeting the APCs through the PS receptor. Calcium phosphate was utilized to co-precipitate the STING in the liposome core, and bypass lysosomes. Delivery of the liposomes in mice bearing melanoma tumors in lungs via aerosolization significantly enhanced the STING signaling in DCs, providing better control of treated and untreated lung metastasis in combination with radiotherapy [Citation69]. Overall, current data suggest that the liposome NP-ISVs are well-positioned to aid immunotherapy in the years to come.
3.1.2. Polymer-based NP-ISVs
The investigation of polymeric NP-ISVs continue to be on the rise for cancer immunotherapy. Like cationic lipids, polymeric ISVs incorporating positively charged moieties improve the cellular delivery of therapeutics and gene products in tumors. In particular, polycationic polyethylenimine (PEI) polymers is highly unique in its ability to achieve intrinsic TLR activation activity of the tumor associated DCs. Ruiz et al. reported a PEI-based nanocomplex for nonviral siRNA therapy of murine ovarian cancer [Citation70]. They showed that PEI improved therapeutic outcomes by acting as a TLR5 agonist to reverse the tolerogenic phenotype of tumor associated human and murine DCs. In another study, the intratumoral administration of PEI stimulated the expression of IL-12 and TNFα from myeloid-derived suppressor cells (MDSCs) and caused the production of tumor suppresive M1 phenotype [Citation71]. Another polymer type with immunomodulatory properties is poly(lactic-co-glycolic acid) (PLGA). PLGA is biodegradable and FDA approved, and is efficiently phagocytosed by the APCs. Nikitczuk et al. developed PLGA-based NP-ISV encapsulating a tumor antigen and the TLR9 agonist CpG motif DNA. Intratumoral administration of the NP in a mouse model of T-cell lymphoma induced an IFNγ Th1 response, reducing local tumor growth [Citation72]. PLGA-NPs also achieve concurrent chemo- and immune-therapy. Silva et al. co-loaded doxorubicin, TLR3 agonist poly (I:C) and TLR7/8 activator (Resiquimod; R848) in the PLGA-NPs. Intratumoral administration of the NP-ISV in treatment resistant TC-1 lung carcinoma and MC-38 colon adenocarcinoma not only induced chemotherapeutic sensitization, but also enhanced the population of tumor-specific T-cells, achieving superior regression and survival rates compared to the drug alone [Citation73]. PLGA has also been found to capture released tumor antigens. Min et al. modified the PLGA surface with amine-polyethylene glycol (NH2-PEG) (NH2 AC-NP) and 1,2-Dioleoyloxy-3-(trimethylammonium)propane (DOTAP AC-NP) to bind the released antigens during radiation therapy in tumors. Data suggested efficient retention of DAMPs such as HMGB1 in NPs in vitro [Citation74]. Also, when the NP-ISV was injected unilateraly in mice bearing bilateral melanoma, it induced an abscopal effect via improved APC presentation and T-cell cytotoxic effects. Further, its combination with checkpoint blockade therapy (CBT) resulted in a 20% complete response rates in the mice subjects. Overall, polymeric NP-ISV platform can be highly efficient in generating an immune-activated TME.
3.1.3. Chitosan-based NP-ISVs
Chitosan-based nanomaterials are another organic NP that can be highly relevant for antitumor immunity. The positively charged protonated amine groups of chitosan can efficiently bind to dendritic cells [Citation75]. Although chitosan in native form is not immunogenic, the development of mannosylated, zwitterionic, and glycated chitosan had enhanced its use as a vaccine adjuvant [Citation76]. So far, majority of anti-tumor immunization with chitosan has been attempted by subcutaneous, intramuscular and intraperitoneal routes [Citation75,Citation77,Citation78]. However, some reports of its use as a NP-ISV are starting to emerge. Kim et al. developed mannosylated chitosan (MC) for IL-12 gene delivery into tumor resident DCs [Citation79]. The presentation of IL-12 via DCs subsequently activated T-cells. In their NP-ISV approach, the team leveraged the high expression of mannose receptors on immature DCs to attain localized targeting and ligand mediated endocytosis of IL-12 gene with chitosan. Compared to chitosan alone, the MC/IL-12 gene delivery significantly improved the APC function, and IFNγ production to suppress tumor growth of murine colon cancer. Zaharoff et al. similarly formulated chitosan/IL-12 cytokine, and utilized it as an ISV [Citation80]. Although particle characterization was not performed to verify the nanosized range of the chitosan/IL-12, the intratumoral administration in colorectal and pancreatic murine cancers achieved 80–100% regression of the tumors. Mechanistic characterization of immune cells showed a high CD8+ and NK-dependent killing of tumor cells. Importantly, rechallenge in the cured mice protected them from tumor induction, verifying immunomodulatory effects in the TME. Similar outcomes were also obtained in bladder cancer with intravesicle administration of chitosan/IL-12. An enhanced IL-12 penetration with reduced and durable protection from bladder cancer was noted [Citation81]. Other applications of chitosan based NP-ISV include its use in photothermal immunotherapy for improved antitumor immunity (see Section 3.3) [Citation82]. Overall, various kind of chitosans can serve as a vaccine adjuvant alone or in combination with therapeutic devices, making them a clinically relevant NP-ISV.
3.1.4. Inorganic NP-ISVs
Inorganic NPs (gold, silica etc.) are known to trap tumor cell antigens and induce APC activations [Citation83]. In particular, gold NPs can trap immunosuppressive cytokines to increase the infiltration of T-cells in the tumor. For example, TGF‐β1 attenuates the function of dendritic and T-cells. Tsai et al. showed that when gold NPs (100 nM) co-mixed with 6 × 105 MBT‐2 cells were inoculated in the mice, the tumor growth inhibition was attenuated by trapping of TGF‐β1 by S-Au covalent bonding. Additionally, an enhanced CD4+ cells in the tumor and immune mediated tumor control was observed [Citation84]. Like cysteines (SH), gold NPs can also bind uncapped cytosine-phosphate-guanine (CpG) motifs. CpG activates TLR9 in APCs to aid T-cell activation. Upon loading the CpG on citrate coated gold NPs, and their intratumoral injection (1013 particles), mice bearing B16-OVA tumors achieved infiltration of dendritic cells, macrophages, and CD8+ cells in the tumor, resulting in regression of the masses. Finally, the application of gold particles is being extended in the realm of photothermal NP-ISV therapy (see 3.3). Nam et al. reported a photothermally stable gold NP-ISV that upon intratumoral injection and light exposures induced a ∼4-fold higher frequency of colon cancer specific CD8+ T cells than untreated control in murine model [Citation85]. This NP-ISV platform also enhanced doxorubicin chemotherapy, and induced an abscopal effect in CT26 colon cancer model.
Like gold, silica (Si) NPs with porous surface efficiently trap antigens to achieve anti-tumor immunization [Citation86]. In particular, Si particles with larger pore sizes demonstrate superior antigen cross-presentation, and anti-tumor immunizations by subcutaneous route [Citation87]. Since Si-NPs are rapidly phagocytosed by the immune and tumor cells, they induce cytotoxic effects by inflammation, oxidative stress and autophagy [Citation88]. An et al. elegantly leveraged this property to develop cationic silica NPs and conjugated it to a STING stimulator (cyclic dimeric guanosine monophosphate: c-di-GMP) [Citation89]. Upon administration as a NP-ISV, Si induced necrotic cell death of melanoma cells, tumor regression, and prolonged survival rates. Immunologically, the necrotic cell death achieved local infiltrations of neutrophil and APCs, and importantly, the mice that were cured with the NP-ISV resisted rechallenge with tumor cells in a melanoma specific CD8+ cell manner [Citation89]. Moving forward, characterizating the role of Si surface compositions (nanoporous, mesoporous, amorphous, etc.) on tumor immunotherapy, dosing regimens, and immune activations depending on baseline tumor type can shed more light on its potential therapeutic use.
Finally, mixed formulations of organic/inorganic NPs are also under investigation (). For example, an immunotherapeutic gel with biocompatible, anti-CD47-loaded CaCO3 NPs was developed. Upon spraying into the surgical site post-resection surgery, it raised the pH of the acidic TME and repolarized M2-TAMs into M1-like TAMs in B16F10 tumors. The reduction of IL-10 (associated with M2-TAMs), increase of IL-12 (associated with M1 macrophages) and reductions in MDSCs, Tregs and HIF-1α expression achieved potent antitumor effects [Citation97]. Complexed NP-ISVs have also been included in the chemotherapy (e.g., DOX) regimens to leverage the benefits of ICD. In one study, DOX-induced ICD was combined with dendritic mesoporous organosilica NPs containing Cu2+ ions and tetrasulfide groups. A five-fold increase in ROS production via glutathione depletion and Fenton’s reaction in tumors with the NPs was achieved. When the NPs were combined with DOX, it achieved a systemic antitumor effect in the 4T1 breast cancer model by increasing the TNFα and cytolytic T-cells. Additionally, this approach synergized with the CBT [91]. This particular approach also demonstrated the unique role of functionalized NPs in NP-ISV, allowing administration of Cu2+ ions encased in mesoporous silica NPs that would otherwise be systemically toxic when combined with DOX’s ICD effects.
Table 2. Synthetic NP-ISV platforms, mechanisms, and treatment approaches for anti-tumor immunity Induction.
To summarize, organic and inorganic NPs immune effects stem from their ability to target multiple aspects of the TME. These include upregulation of proinflammatory cytokines, maturation of APCs, direct tumor cell lysis, and antigen release and capture. Aspects of NP-ISVs that needs optimization include uniform activation of immune-related signaling pathways in heterogeneous TME. Additionally, ensuring that NP-ISVs do not impact systemic modalities of treatment (e.g., chemotherapy, CBT) appears essential for successful outcomes.
3.2. Biological NP-ISV approaches
NPs that are derived from viral or bacterial components can also swiftly activate the immune system. Examples of important NP-ISV players derived from biological products are described below.
3.2.1. Viral NPs (VNPs)
VNPs are derived from plants, and can self-assemble into various shapes and sizes [Citation106]. VNPs do not replicate in mammals, and thus can be administered in high doses with minimal toxicity. In particular, cowpea mosaic virus (CPMV) and its RNA free counterpart (empty CPMVs or eCPMV) are highly immunogenic, readily available, scaleable, and chemically modifiable [Citation107,Citation108]. eCPMV and CPMV-NPs achieve high immunogenicity by inducing fast recruitment of APCs in tumor compared to other plant VNPs (e.g., tobacco mosaic VNPs or TMVs) [Citation109] and immunogens (e.g., LPS, poly(I:C), and the STING agonist DMXAA). In general, the presence of RNAs in CPMV attains relatively superior antigen presentation compared to eCPMVs [Citation108]. Regardless, eCPMV have shown to upregulate IL-1β, IL-6, IL-12p40, MIP1-α, and TNF-α from bone marrow-derived DCs, and macrophages. ISV with eCPMV also causes potent tumor regression in a variety of murine models including metastatic breast, colon and ovarian carcinoma models (4T1-luc breast cancer, CT-26, and ID8-Defb29/Vegf-A ovarian) [Citation110]. eCPMV and CPMVs exerts their effect by enhancing the proliferation of neutrophils, especially the antitumoral N1 neutrophil phenotype tumors. Recently, the CPMV was also shown to work by targeting TLRs in gliomas [Citation111]. This property of the CPMV increased the tumor infiltrating neutrophil and natural killer cell populations, and immunotherapy outcomes following ISV in murine glioma. The key to maximal CPMV-ISV effect is its phagocytosis by the APCs. Thus, CPMVs are typically administered in multiple injections to tumors for enhanced effects. To reduce the frequency of treatment, Wang et al. combined CD47 blockade and CPMV-ISV therapy in murine breast and ovarian cancer [Citation112]. Since CD47 inhibits antiphagocytic signals, the team hypothesized that the infiltration of APCs in tumor by the CPMV, and the CD47 activation of pro-phagocytic signals would aid rapid tumor clearance to reduce dosage/frequency. Survival and tumor regression data suggested that CD47 indeed potentiated the CPMV immunotherapeutic effects relative to CPMV alone. In another study, Czapar et al. modified CPMVs with charged dendrimers to form slow releasing nanoplex depots [Citation113]. CPMVs are negatively charged, and can electrostatically interact with positively charged polymers such as polyamidoamine (PAMAM) dendrimers at low salt concentrations. At physiological salt concentrations in situ, they can slowly disassemble to release the CPMVs. Investigation of this approach in an orthotopic murine ovarianc cancer model revealed that a single injection of the PAMAM-CPMV was as effective as an ISV compared to weekly treatment with the soluble CPMV. These studies suggest that particle functionalizations may be needed for CPMV enhanced immune effects in tumors.
An aspect of CPMV-ISV that has impeded its clinical translation is the concern regarding the presence of anti-CPMV antibodies in the human population. Humans are exposed to plant viruses through the food chain, and may have CPMV antibodies. Thus, it is plausible that the ISV administration of CPMV can cause anaphylactic reactions or diminished therapeutic effects in cancer patients. Shukla et al. investigated the role of preexisting antibodies on eCPMV ISV efficacy against ovarian cancer [Citation114]. They found that the CPMVs were effective in both naïve and CPMV-pre-exposed mice. In fact, the presence of CPMV neutralizing antibody enhanced the CPMV therapeutic effect. They explained this phenomenon to a superior opsonization and phagocytosis of CPMVs by the APCs in the TME. Since wild type CPMVs contain RNAs and activate TLR7/8 to generate a stronger humoral antibody response, the research team also investigated whether this can influence eCPMV therapy. eCPMV-ISV was administered in CPMV naïve or exposed murine ovarian cancer model. Data suggested that the CPMV pre-exposure also enhanced the efficacy of eCPMVs in a similar manner. Overall, CPMVs are an attractive candidate for NP-ISV. The next steps are to assess their safety and translational potentials in human clinical trials.
Other plant VNPs investigated as an ISV include potato and papaya mosaic virus. Especially, Potato-virus X (PVX) NPs have displayed antitumor and immune efficacy against B16F10 tumors as an ISV [Citation115]. The effect of PVX VNPs can also be amplified with chemotherapies like DOX to increase the IL-1β, MIP-1α, MIG, MCP-1, M-CSF, and RANTES in the B16F10 tumors. Similarly, Papaya Mosaic virus based VNPs increased the IFN-α-dependent pathway, and synergy with anti-PD-1 antibody CBT in melanoma [Citation116]. The inclusion of modified multifunctional VNPs is an active goal of current ISV regimens. However, as Lee et al. showed, combining many aspects into a singular VNP seems to not always be more efficacious than co-administered therapies in their model of PVX and DOX [Citation115]. The tumor biology and the immune system have to guide the strategies of applying multifunctional VNPs of any sort. Overall, VNPs are versatile systems for bioconjugation, loading, and genetic approaches, increasing their possibility of use as an ISV [Citation117].
3.2.2. Bacteria-derived NP-ISV
Minicells are nanosized, spherical proteolipid vesicles secreted by bacteria [Citation118–120]. So far, the use of nanosized achromosomal, nonreplicating minicells, produced during genetically controlled abnormal bacterial division has been mostly for drug-loading and labeling cancer targeted antibodies rather than ISV [Citation121–126]. Many bacterial constituents are PAMPs, so minicells can be hypothetically an inherent immunostimulatory platforms for NP-ISV. Minicells can be genetically engineered to exploit effective type III secretion systems, which include ‘complex delivery of bacterial virulence effector proteins into the host cell cytosol’. This can increase cytotoxic T cell response; features not typically seen in other bacterial cancer vaccine platforms [Citation127]. Hancock et al. developed recombinant bacterial minicells from Escherichia coli (VAX014). VAX014 was engineered to deliver perfringolysin O (PFO) to α3β1 or α5β1 integrin expressing human and murine urothelial carcinoma cells. Intravesicle instillation of VAX0014 via transurethral catheterization into the bladders cancers improved the survival response [Citation128]. In addition, successful loading of chemotherapies into minicells including paclitaxel, doxorubicin, carboplatin, cisplatin, and vinblastine have been reported, and these can be leveraged for immunogenic cell death induction in ISV setting [Citation119] and use as NP-ISV [Citation129].
3.3. Combination of energy depositing/hyperthermia devices with NP as ISVs
Therapeutic energy-depositing devices such as photothermal/photodynamic therapy (PTT), alternating magnetic field (AMF), focused ultrasound (FUS), radiowave therapy or widely used ionizing radiation therapy can serve as an external modes of inducing immunogenic cell death, making them a potential part of ISU with NPs [Citation91,Citation92,Citation130–132]. The NPs-ISVs can also mediate the effects of external energy deposition into the solid tumor to increase the overall ISV immune response.
3.3.1. Photothermal (PTT) NP-ISV
PTT typically uses near infrared laser energy to excite NP-ISVs [Citation133]. The activation/absorption of light energy by NP-ISVs (e.g., gold NPs, carbon nanotubes, indocyanine green, Prussian blue etc.) causes physical, hyperthermic, and ablative effects in tumors and a variety of downstream immune effects [Citation134–137]. A detailed discussion of all PTT-based ISV approaches is beyond the scope of this review. Here, we primarily focused on the immune activation mechanisms, and describe selected studies that shows the feasibility of PTT with NP-ISVs for local and abscopal effects.
Bear et al. investigated the mechanisms of elimination of metastatic melanoma with PTT induced using PEGylated gold NP-ISV [Citation138]. The team assessed immune activations in poorly immunogenic B16F10 and highly immunogenic B16-OVA flank tumors. Following intratumoral administration of the gold NPs (40–42nm), a near-infrared laser (λ = 808 nm, 3 W/cm2, spot diameter = 8 mm) was applied in the tumor for 3 min. PTT enhanced local and systemic antitumor immune effect against the B16-OVA model by increasing the populations of tumor specific CD4+ and CD8+ effector T cell. Importantly, the increase in the infiltration rates of CD4+ and CD8+ in the distant untreated tumor was not associated with changes in the immunosuppresive Tregs, and this establishing the basis of abscopal effect. While the Tregs were unaffected, the untreated tumors showed an increase in the population of MDSCs, and this eventually resulted in the T-cell dysfunction and tumor re-growth. Interestingly, in contrast to the immunogenic B16-OVA tumors, the DC maturations in the poorly immunogenic B16F10 did not achieve abscopal effect. These indicate that PTT-NPs require high tumor cell antigenicity for successful ISV outcomes. In another study, Nam et al. coated gold NPs with polydopamine to improve the PTT efficiency of gold NPs, and correlated those to antitumor immune effects [Citation85]. Intratumoral administration of the gold-NPs, and exposure to PTT with subtherapeutic dosages of doxorubicin elicited prominent local and systemic elimination of CT26 colon and lung masses. This was ascribed to the upregulation of MHC-II and CD40 on DCs in tumor-draining lymph nodes, proliferation of tumor specific CD8+, CD107a expressing NKs, and hyperthermia induced increase in HSP70 [85]. Similarly, Mejia et al. described an elegant approach to transform the TME of neuroblastoma tumors with Prussian blue based NP(PB-NP) [Citation139]. PB-NPs are inexpensive, easy to synthesize and scale, and are FDA approved. The team developed a tumor pH (5–7) stable PB-NP for intratumoral therapy, and activated it with 808 NIR laser. The combined therapy rapidly decreased the neuroblastoma burden and prevented recurrence in the murine model by a local increase of cytotoxic T-cells compared to PB-NP alone. Also, while T-cell specificity was impressive, the best results were attained in combination with anti-CTLA-4s, suggesting a need for such agents in the PTT regimen.
Additional mechanisms to drive PTT NP-ISVs include the targeting of specific cellular pathways in the tumor. Chen et al. proposed that intratumoral therapies that are ablative kill the resident immune cells to reduce its efficacy [Citation92]. The team hypothesized that hyperthermia (40–45 °C) that causes HSP70 release from tumor cells can enhance the infiltrations of APCs, which can then be activated with resiquimod, a TLR7/8 agonist to achieve IL-6 and tumor necrosis factor alpha secretions in TME for anti-tumor immunity. A novel NP-ISV composed of photothermally responsive polyaniline (PANI)-based amphiphilic polymers was generated. Hydrophobic resiquimod was loaded in the hydrophobic polyaniline (PANI) core, and the aqueous stability was imparted by covalently linking the polymers with hydrophilic glycol-chitosan (GCS) backbone. Mild heating (40–45 °C) of mice CT colon tumors injected with the NP was performed with NIR lasers (0.9 W/cm2 for 10 min). A significant enhancement in the tumor regressions and survival for the PTT + NPs compared to the monotherapies, and a resistance to rechallenge mediated by the IFNγ expressing CD8+ was noted. Immunologically, the treated tumors showed enhanced IL-6 and decreased levels of anti-inflammatory IL-10 cytokine and a significant drop of MDSC populations; all of which reversed the suppressive TME. Additional PTT approaches utilizing ICG, CpG, and iron NPs are described in . Overall, NP-ISV with PTT enhances proinflammatory and antitumor effects in murine models. The current and future challenges are in coordinating dosing and timing to optimize efficacy in various tumor types.
3.3.2. Magnetic NP-ISV
ISV using magnetic NPs (MNPs, typically <100nm) relies on uniform tumor heating (>40 °C) with alternating magnetic field (AMF). Duval et al. utilized the RNA quantification technique to understand the immunogenic effects of low dose (CEM43 30) MNP hyperthermia on melanoma cells in vitro. The team showed that HSP70 and a number of TLR pathway genes are upregulated with MNPs plus AMF [Citation140]. This was also verified in T-9 rat glioma [Citation141] and B16 melanoma mouse models [Citation142]. A detailed review of how MNPs and AMF synergize to improve immune effect has been elegantly described by Kobayashi [Citation143]. To summarize, depending on the exposure conditions, the MNP-AMF achieves immunogenic cell death, expression of HSP70 that stimulates APC activation and MHC-I presentation, and marked augmentation of tumor selective cytotoxic T-lymphocytes. An interesting aspect of MNP-based AMF hyperthermia has been its ability to synergize with radiation therapy. Hoopes et al. showed that canine oral melanomas pre-heated with MNPs and AMF (43 °C for 60 min), and then treated with combined radiation and CPMV demonstrated significant tumor regression [Citation98]. Histologically, an upregulation of the inflammatory markers, and conversion of the ‘cold’ tumor into a ‘hot tumor’ was observed with this approach. Likewise, Oei et al. showed that MNPs hyperthermia (43 °C for 20 min) or partial ablation (5 °C for 5 min plus 43 °C for 15 min) performed 7 days post inoculation of triple negative breast cancers improved the efficacy of radiation (8 Gy; 3x) and dual-CBTs (administered on Days 7, 9, 11, and 13 after tumor implantation). An enhanced populations of T-cells following the combinatorial therapies presumably due to greate tumor damage/necrosis relative to monotherapy in tumors were noted. Although promising, the abscopal effects using this approach was modest [Citation144]. These studies () suggest that optimizing the MNP hyperthermia duration, frequency, and precise control of thermal energy deposition is needed prior to its application as an ISV. Additionally, further modifying the MNPs to leverage superior effects may be needed. For example, Manganese ferrite (MnFe2O4) utilizes the iron-oxide core to downregulate M2-associated cytokines (e.g., interleukin 10 (IL-10). It can be combined with AMF and PTT hyperthermia for superior anti-tumor immune effects [Citation144,Citation145]. Recently, Zhou et al. reported OVA protein modified PEGylated MnFe2O4 NPs loaded with R837 DC stimulant (R837-OVA-PEG-MnFe2O4 NPs) [Citation146]. ISV with the functionalized MNP in 4T1 tumor model allowed MRI tracking of the NPs in the orthotopic tumor, and its combination with PTT decreased M2 cytokines and superior immune effects, enhancing the protection against lung metastasis.
3.3.3. Focused ultrasound (FUS)-based NP-ISV
Focused ultrasound (FUS) is a noninvasive sound energy based modality for generation of mechanical and thermal effects in a tumor. We have shown that FUS improves the immune and chemotherapeutic outcomes of intravenously administered therapies [Citation145–147]. For instance, we attached doxorubicin-loaded thermally sensitive liposomes onto Salmonella typhimurim bacteria to modulate the population of antitumoral M1 and protumoral M2 macrophages in the tumor [Citation146]. We found that the NP-laden bacteria demonstrated high chemotaxis and colonization in the murine colon cancer tumors. Combination with application of local mild hyperthermia (40–42 °C; 30 min) with FUS caused a pro-inflammatory immune environment, and polarization to M1 phenotype, regressing the tumor. A key benefit of FUS over PTT or AMF modality is its ability to generate hyperthermia without NPs. FUS parameters are also tunable for various types of immune priming. For instance, low intensity FUS (LOFU; peak negative pressures from ∼2 to 5 MPa) modulates the expression and cellular distribution of HSPs family protein (e.g., HSP 60, 70, and 90). This was shown to enhance radiotherapy outcomes of breast and prostate murine cancers [Citation148]. In contrast, ablative FUS rapidly debulks tumor to release tumor antigens. Silvestrini et al. showed that the efficacy of TLR9 agonist and CBT was significantly improved by FUS ablation [Citation149]. FUS can also induce mechanical effects to enhance tumor cell stress and antigen release. Eranki et al. performed rapid and partial emulsification of neuroblastoma tumors using boiling histotripy (BH) [Citation150]. In this approach, millisecond-long pulses, with lower pulse resonance frequency and duty cycle FUS were employed to achieve millisecond heating (∼100 °C) that is typically non-denaturing. They showed that BH significantly increased the infiltration of T cells, PD-L1, and IL-2 expression in the tumor, systemic cytokines, and NK cells in spleen and lymph node within 24 h of treatment. Although BH alone was not effective in inducing abscopal effect, its combination with CBT improved the abscopal effects.
Knowing that FUS is most effective in a combinatorial setting, we and others have started incorporating immunoadjuvants including NP-ISVs to investigate their immunotherapy potentials. Recently, we developed a DAMP inducing liposome NP-ISV [62] that upregulated calreticulin in the tumors. When we combined CRT-NPs with FUS hyperthermia, T-cell-mediated abscopal effect in poorly immunogenic B16F10 melanoma model was achieved. Suzuki et al. enhance IL-12 gene therapy in murine ovarian cancer with FUS cavitation to improve therapeutic outcome [Citation151]. In this study, IL-12 was expressed in the tumor cells using liposome NPs loaded with sonosensitive bubble agents. Upon administration of the NP-ISV and application of the ultrasound (1 MHz, 0.7 W/cm2, 60 s), complete regression occurred in 80% of the tumor-bearing mice by the cytotoxic actions of IL-12 activated CD4+ and CD8+ T-cells. We and others have also utilized nonnanoparticule immunoadjuvants [Citation152]. For example, we combined intratumoral CD40 agonist antibodies with FUS mild hyperthermia in the B16F10 model [Citation130]. We observed that this approach preserved the T-cell function to cause abscopal effect. FUS combined NP-ISV is still in early stages, but tailoring NP properties and their bioeffect will aid its clinical translation efforts.
3.4. NP-ISV + CBT therapy
Monotherapy and combinatorial therapy with anti CTLA-4, PD-L1, PD-1 monoclonal antibody has gained FDA approved for a variety of indications including metastatic melanoma. CBTs work by inhibiting the T-cell immunosuppressive costimulations (e.g., CTLA-4 interacts with B7 ligands B7-1 (CD80) and B7-2 (CD86) on APCs to attenuate T-cell functions anti-CTLA-4 inhibits that process) [Citation153]. Although CBTs are effective in a minority of advanced stage melanoma, squamous cell lung cancer, renal cell carcinoma, and classical Hodgkin lymphoma patients, a large proportion with malignant disease do not respond to such therapies and can even demonstrate adverse effects. These indicate a need for the development of novel approaches to improve the populations of cell specific T-cells at reduced treatment dosages [Citation154].
Resistance to CBTs can occur by multiple mechanisms in a tumor. The T-cells can undergo anergy via interactions of its PD-1 and CTLA-4 with PD-L1 on tumor cells, or CD80/86 on APCs [Citation155]. CTLA-4 on Tregulatory cells can also interact with the B7s on APCs to inhibit its function in a tumor. Tumor cells can also reduce their antigen presentation by inducing mutation and loss of β-2-microglobulin (B2M), which processes tumor antigens, deleting MHC class I polypeptide-related sequence A and B (MICA/B) that reduces detection by the NK cells, reducing the expression of Fas, TRAILs, Caspase 8 that activates the apoptotic pathways, secreting immunosuppressive metabolites (2,3-dioxygenase (IDO) that reduces T-cell proliferations, and altering the oncogenic signals that affect T-cell responses [Citation156]. NP-ISVs have direct access to tumor and immune cell population, and thus can target such immunosuppressive signals in CBT refractory tumors. NP-ISVs can also increase the tumor cell antigenicity by increasing DAMP mediated APC activity to improve the T-cell infiltration. This can be particularly relevant to increase T-cell activity for tumors with low to moderate inflammations (e.g., pancreatic, prostate, and glioblastoma) [Citation157,Citation158].
Several recent studies report the enhancement of CBT efficacy with NP-ISVs. Wang et al. intratumorally injected PEG grafted single walled carbon nanotubes (SWNT; dose = 0.33 mg/kg) and combined those with an 808‐nm NIR laser at 0.5 W/cm2 for 10 min to ablate 4T1 murine breast tumors. Immunologically, the treatment-induced local control, DC maturation in the lymph node, and secretion of pro‐inflammatory cytokines IL‐1β, IL‐12p70, IL‐6, and TNF‐α. To evaluate abscopal effect, the mice were rechallenged with 4T1 cells to mimic metastatic condition. Data suggested a significant increase in the populations of Tregs in the untreated tumor and tumor growth. To overcome this barrier, the researcher administered anti‐CTLA‐4 mAb to target CTLA‐4 on the T cells (Tregs). This resulted in a three-fold increase in the CD8+ T cells in the secondary tumors, thereby enhancing the immunological response. For effective outcomes, anti-PD-1 therapy is typically administered for >1 year, and this may cause severe adverse effect. Luo et al. reported an alternative approach of PTT activatable poly(lactic-co-glycolic) acid (PLGA) NPs encapsulating hollow gold nanoshells (photothermal agent) and AUNP12 (anti-PD-1 peptide) for sustained anti-PD-1 immunotherapy, especially in combination with TLR9 activation of CpG [Citation159]. Intratumoral administration of NPs + CpG and NIR laser exposure (1.5 W/cm2, 3 min) in a 4T1 breast cancer model significantly enhanced the activated DCs and CD8+ T cell populations in the tumor. Systemically, the combined NP + CpG treatment caused inhibition of distant tumor growth, which was attributed to the peridural anti-PD1 release in the primary tumor that caused humoral immunity in mice. Tselikas et al. similarly investigated the role of direct intratumoral injections of anti-CTL4 loaded NPs on anti-metastatic therapy [Citation160]. The team posited that direct injections of mAbs to tumors may require repeated injections to achieve sustained effect, cause in-homogeneous distribution with maximal localization in the low-pressure necrotic areas, and leak from the TME to outside. To overcome this, PLGA-NPs and anti-CTLA-4 antibodies were suspended in an ethiodized oil to achieve slow and sustained controlled immunomodulation in the tumors. Although the response rates for the anti-CTLA-4 alone and the NP-ISV groups were found similar in the highly immunogenic CT26 model, this approach may still be of value in advanced stage poorly inflamed tumor. Several similar formulations based on CBT-NP-ISV approaches have been reported [Citation91,Citation94,Citation95,Citation139]. Overall, it is clear that CBT with immune-stimulating NP-ISV creates a stronger antitumor responses and a more immunogenic TME compared to CBT or NP-ISV monotherapy.
4. Conclusions
Synthetic and biological NP-ISVs are versatile and amenable to carrying reagents. The interaction of NPs with phagocytes and cancer cells in a solid tumor generates a broad spectrum of bioeffects as part of ISV. NP-ISVs directly kill cancer cells, stimulate local antitumor immunity, generate systemic antitumor immunity, enhance immunological memory, and aid CBT outcomes. The mechanisms are via stimulation of innate immune cells and associated induction of T-effector cell responses against tumor-associated antigens or neoantigens. NP-ISVs can incorporate multiple immunotherapy reagents, proteins, cytokines, adjuvants, antigens, and can be combined with energy applicators to increase immunogenic cell death and attain synergistic responses. As the NP-ISV immunomodulatory approaches gain pace, eliciting immunological memory against distant, metastatic, or deep-seated tumors will continue to be the goal against which each new iteration will be compared.
5. Future perspectives
It is clear that tumor immunosuppression is an outcome of cross-talks between the immune, stromal, and cancer cells. While targeting a certain cell type in the tumors locally with NPs has merits, future investigation should consider developing multifunctional formulations that concurrently activates multiple cell types in a tumor. In this regards, an aspect of NP-ISV that is gaining pace is the loading of multiple immunomodulatory agents in the same platform. While interesting, ensuring that the effects of encapsulated agents are synergistic via multiple pathways with such platforms are crucial.
An aspect of cancer immunotherapy where NP-ISVs can play an important role is their incorporation in CBT regimens. CBTs are currently effective in minority of patients, so reversing immunoresistance through targeting of TME (e.g., reducing Treg populations, preventing deletion of genes responsible for MHC-I expressions, enhancing overall tumor inflammation, etc.) should improve the therapeutic responses. New research in murine models with multifocal and immunoresistant tumors, and the inclusion of genetically engineered models can shed more light on their potentials for eventual clinical use. Finally, the intratumoral distribution of NPs as an ISV is not similar to parentally injected NPs. Little research is focused on optimizing the distributions rates, or tracking their locations in real-time. Use of image-guided NP-ISVs can be highly insightful in such cases.
It is also critical to increase the repertoire of NP-ISV library so that the best candidate can be selected for specific applications. In this regard, inclusion of newer ISVs incorporating biologically derived NPs such as oncolytic virus based NPs, outer membrane vesicles from bacteria, or exosome—from mammalian cells appears important. With strong immunomodulatory effects and the combined progress in NP formulations, mechanistic understanding of immunotherapy and appreciation of the simple but powerful potential of ISU, NP-ISV approaches will be significant contributors to future immunomodulatory approaches and incorporated widely into standard of care for solid tumors.
Disclosure statement
No potential conflict of interest was reported by the author(s).
Additional information
Funding
References
- Abe BT, Shin DS, Mocholi E, et al. NFAT1 supports tumor-induced energy of CD4(+) T cells. Cancer Res. 2012;72:4642–4651.
- Rabinovich GA, Gabrilovich D, Sotomayor EM. Immunosuppressive strategies that are mediated by tumor cells. Annu Rev Immunol. 2007;25:267–296.
- Zamarin D, Ricca JM, Sadekova S, et al. PD-L1 in tumor microenvironment mediates resistance to oncolytic immunotherapy. J Clin Invest. 2018;128:1413–1428.
- Wei SC, Anang NAAS, Sharma R, et al. Combination anti-CTLA-4 plus anti-PD-1 checkpoint blockade utilizes cellular mechanisms partially distinct from monotherapies. Proc Natl Acad Sci U S A. 2019;116:22699–22709.
- Zamarin D, Holmgaard RB, Subudhi SK, et al. Localized oncolytic virotherapy overcomes systemic tumor resistance to immune checkpoint blockade immunotherapy. Sci Transl Med. 2014;6:226ra32.
- Morello S, Capone M, Sorrentino C, et al. Soluble CD73 as biomarker in patients with metastatic melanoma patients treated with nivolumab. J Transl Med. 2017;15:244.
- Deinlein T, Wolf IH, Rainer B, et al. Treatment of primary and metastatic multifocal mucosal melanoma of the oral cavity with imatinib. Case Rep Oncol. 2017;10:558–563.
- Neelapu SS, Locke FL, Bartlett NL, et al. Axicabtagene ciloleucel CAR T-cell therapy in refractory large B-cell lymphoma. N Engl J Med. 2017;377:2531–2544.
- Raje N, Berdeja J, Lin Y, et al. Anti-BCMA CAR T-cell therapy bb2121 in relapsed or refractory multiple myeloma. N Engl J Med. 2019;380:1726–1737.
- Cohen AD, Garfall AL, Stadtmauer EA, et al. B cell maturation antigen-specific CAR T cells are clinically active in multiple myeloma. J Clin Invest. 2019;129:2210–2221.
- Andtbacka RHI, Amatruda T, Nemunaitis J, et al. Biodistribution, shedding, and transmissibility of the oncolytic virus talimogene laherparepvec in patients with melanoma. EBioMed. 2019;47:89–97.
- Franke V, Berger DMS, Klop WMC, et al. High response rates for T-VEC in early metastatic melanoma (stage IIIB/C-IVM1a). Int J Cancer. 2019;145:974–978.
- Jiang J, Zhou H, Ni C, et al. Immunotherapy in pancreatic cancer: new hope or mission impossible?. Cancer Lett. 2019;445:57–64.
- Hammerich L, Binder A, Brody JD. In situ vaccination: cancer immunotherapy both personalized and off-the-shelf. Mol Oncol. 2015;9:1966–1981.
- Mi Y, Smith CC, Yang F, et al. A dual immunotherapy nanoparticle improves T-cell activation and cancer immunotherapy. Adv Mater Weinheim. 2018;30:e1706098.
- Singh M, Khong H, Dai Z, et al. Effective innate and adaptive antimelanoma immunity through localized TLR7/8 activation. J Immunol. 2014;193:4722–4731.
- Fakhari A, Nugent S, Elvecrog J, et al. Thermosensitive gel-based formulation for intratumoral delivery of toll-like receptor 7/8 dual agonist, MEDI9197. J Pharm Sci. 2017;106:2037–2045.
- Brody JD, Ai WZ, Czerwinski DK, et al. In situ vaccination with a TLR9 agonist induces systemic lymphoma regression: a phase I/II study. J Clin Oncol. 2010;28:4324–4332.
- Wang S, Campos J, Gallotta M, et al. Intratumoral injection of a CpG oligonucleotide reverts resistance to PD-1 blockade by expanding multifunctional CD8+ T cells. Proc Natl Acad Sci U S A. 2016;113:E7240–E7249.
- Sagiv-Barfi I, Czerwinski DK, Levy S, et al. Eradication of spontaneous malignancy by local immunotherapy. Sci Transl Med. 2018;10:eaan4488.
- Kwong B, Liu H, Irvine DJ. Induction of potent anti-tumor responses while eliminating systemic side effects via liposome-anchored combinatorial immunotherapy. Biomaterials. 2011;32:5134–5147.
- Hewakuruppu YL, Dombrovsky LA, Chen C, et al. Plasmonic "pump-probe" method to study semi-transparent nanofluids. Appl Opt. 2013;52:6041–6050.
- Baetke SC, Lammers T, Kiessling F. Applications of nanoparticles for diagnosis and therapy of cancer. Br J Radiol. 2015;88:20150207.
- Barenholz Y. Doxil®-the first FDA-approved nano-drug: lessons learned. J Control Release. 2012;160:117–134.
- Elahi-Gedwillo KY, Carlson M, Zettervall J, et al. Antifibrotic therapy disrupts stromal barriers and modulates the immune landscape in pancreatic ductal adenocarcinoma. Cancer Res. 2019;79:372–386.
- Zhou S, Zhang T, Peng B, et al. Targeted delivery of epirubicin to tumor-associated macrophages by sialic acid-cholesterol conjugate modified liposomes with improved antitumor activity. Int J Pharm. 2017;523:203–216.
- Mielgo A, Schmid MC. Impact of tumour associated macrophages in pancreatic cancer. BMB Rep. 2013;46:131–138.
- Grivennikov SI, Wang K, Mucida D, et al. Adenoma-linked barrier defects and microbial products drive IL-23/IL-17-mediated tumour growth. Nature. 2012;491:254–258.
- Kong L, Zhou Y, Bu H, et al. Deletion of interleukin-6 in monocytes/macrophages suppresses the initiation of hepatocellular carcinoma in mice. J Exp Clin Cancer Res. 2016;35:131.
- Bicho A, Peça IN, Roque ACA, et al. Anti-CD8 conjugated nanoparticles to target mammalian cells expressing CD8. Int J Pharm. 2010;399:80–86.
- Horwitz DA, Bickerton S, Koss M, et al. Suppression of murine lupus by CD4+ and CD8+ Treg cells induced by T cell-targeted nanoparticles loaded with interleukin-2 and transforming growth factor β. Arthritis Rheumatol. 2019;71:632–640.
- Schmid D, Park CG, Hartl CA, et al. T cell-targeting nanoparticles focus delivery of immunotherapy to improve antitumor immunity. Nat Commun. 2017;8:1747.
- Sacchetti C, Rapini N, Magrini A, et al. In vivo targeting of intratumor regulatory T cells using PEG-modified single-walled carbon nanotubes. Bioconjug Chem. 2013;24:852–858.
- Ou W, Thapa RK, Jiang L, et al. Regulatory T cell-targeted hybrid nanoparticles combined with immuno-checkpoint blockage for cancer immunotherapy. J Control Release. 2018;281:84–96.
- Cruz LJ, Rosalia RA, Kleinovink JW, et al. Targeting nanoparticles to CD40, DEC-205 or CD11c molecules on dendritic cells for efficient CD8(+) T cell response: a comparative study. J Control Release. 2014;192:209–218.
- Rosalia RA, Cruz LJ, van Duikeren S, et al. CD40-targeted dendritic cell delivery of PLGA-nanoparticle vaccines induce potent anti-tumor responses. Biomaterials. 2015;40:88–97.
- Raghuwanshi D, Mishra V, Suresh MR, et al. A simple approach for enhanced immune response using engineered dendritic cell targeted nanoparticles. Vaccine. 2012;30:7292–7299.
- Hu G, Guo M, Xu J, et al. Nanoparticles targeting macrophages as potential clinical therapeutic agents against cancer and inflammation. Front Immunol. 2019;10:1998.
- Liu Y, Hardie J, Zhang X, et al. Effects of engineered nanoparticles on the innate immune system. Semin Immunol. 2017;34:25–32.
- Oh WK, Kim S, Choi M, et al. Cellular uptake, cytotoxicity, and innate immune response of silica-titania hollow nanoparticles based on size and surface functionality. ACS Nano. 2010;4:5301–5313.
- Jia J, Zhang Y, Xin Y, et al. Interactions between nanoparticles and dendritic cells: from the perspective of cancer immunotherapy. Front Oncol. 2018;8:404.
- Shima F, Akagi T, Akashi M. Effect of hydrophobic side chains in the induction of immune responses by nanoparticle adjuvants consisting of amphiphilic poly(γ-glutamic acid). Bioconjug Chem. 2015;26:890–898.
- Moyano DF, Goldsmith M, Solfiell DJ, et al. Nanoparticle hydrophobicity dictates immune response. J Am Chem Soc. 2012;134:3965–3967.
- Oh JY, Kim HS, Palanikumar L, et al. Cloaking nanoparticles with protein corona shield for targeted drug delivery. Nat Commun. 2018;9:4548.
- Saha K, Rahimi M, Yazdani M, et al. Regulation of macrophage recognition through the interplay of nanoparticle surface functionality and protein corona. ACS Nano. 2016;10:4421–4430.
- Settanni G, Zhou J, Suo T, et al. Protein corona composition of poly(ethylene glycol)- and poly(phosphoester)-coated nanoparticles correlates strongly with the amino acid composition of the protein surface. Nanoscale. 2017;9:2138–2144.
- Gref R, Lück M, Quellec P, et al. Stealth' corona-core nanoparticles surface modified by polyethylene glycol (PEG): influences of the corona (PEG chain length and surface density) and of the core composition on phagocytic uptake and plasma protein adsorption. Colloids Surf B Biointerf. 2000;18:301–313.
- Xiao W, Lin J, Li M, et al. Prolonged in vivo circulation time by zwitterionic modification of magnetite nanoparticles for blood pool contrast agents. Contrast Media Mol Imaging. 2012;7:320–327.
- Moyano DF, Liu Y, Ayaz F, et al. Immunomodulatory effects of coated gold nanoparticles in LPS-stimulated in vitro and in vivo murine model systems. Chem. 2016;1:320–327.
- Kato Y, Ozawa S, Miyamoto C, et al. Acidic extracellular microenvironment and cancer. Cancer Cell Int. 2013;13:89.
- Wu L, Zhang Y, Li Z, et al. "Sweet" architecture-dependent uptake of glycocalyx-mimicking nanoparticles based on biodegradable aliphatic polyesters by macrophages. J Am Chem Soc. 2017;139:14684–14692.
- Morris ZS, Guy EI, Werner LR, et al. Tumor-specific inhibition of in situ vaccination by distant untreated tumor sites. Cancer Immunol Res. 2018;6:825–834.
- Iyoda T, Yamasaki S, Kawamura M, et al. Optimal therapeutic strategy using antigen-containing liposomes selectively delivered to antigen-presenting cells. Cancer Sci. 2019;110:875–887.
- Jain S, Tran TH, Amiji M. Macrophage repolarization with targeted alginate nanoparticles containing IL-10 plasmid DNA for the treatment of experimental arthritis. Biomaterials. 2015;61:162–177.
- Wang Y, Lin YX, Qiao SL, et al. Polymeric nanoparticles promote macrophage reversal from M2 to M1 phenotypes in the tumor microenvironment. Biomaterials. 2017;112:153–163.
- Mahon OR, Browe DC, Gonzalez-Fernandez T, et al. Nano-particle mediated M2 macrophage polarization enhances bone formation and MSC osteogenesis in an IL-10 dependent manner. Biomaterials. 2020;239:119833.
- Wang AZ, Langer R, Farokhzad OC. Nanoparticle delivery of cancer drugs. Annu Rev Med. 2012;63:185–198.
- Amidi M, de Raad M, Crommelin DJ, et al. Antigen-expressing immunostimulatory liposomes as a genetically programmable synthetic vaccine. Syst Synth Biol. 2011;5:21–31.
- Kanaoka E, Takahashi K, Yoshikawa T, et al. A novel and simple type of liposome carrier for recombinant interleukin-2. J Pharm Pharmacol. 2001;53:295–302.
- Kanaoka E, Takahashi K, Yoshikawa T, et al. Continuous release of interleukin-2 from liposomal IL-2 (mixture of interleukin-2 and liposomes) after subcutaneous administration to mice. Drug Dev Ind Pharm. 2003;29:1149–1153.
- Anderson PM, et al. Depot characteristics and biodistribution of interleukin-2 liposomes: importance of route of administration. J Immunother. 1992;12:19–31.
- Sethuraman SN, Singh MP, Patil G, et al. Novel calreticulin-nanoparticle in combination with focused ultrasound induces immunogenic cell death in melanoma to enhance antitumor immunity. Theranostics. 2020;10:3397–3412.
- Meraz IM, Savage DJ, Segura-Ibarra V, et al. Adjuvant cationic liposomes presenting MPL and IL-12 induce cell death, suppress tumor growth, and alter the cellular phenotype of tumors in a murine model of breast cancer. Mol Pharm. 2014;11:3484–3491.
- Francian A, Namen S, Stanley M, et al. Intratumoral delivery of antigen with complement C3-bound liposomes reduces tumor growth in mice. Nanomedicine. 2019;18:326–335.
- Nomura T, Koreeda N, Yamashita F, et al. Effect of particle size and charge on the disposition of lipid carriers after intratumoral injection into tissue-isolated tumors. Pharm Res. 1998;15:128–132.
- Harrington KJ, Rowlinson-Busza G, Syrigos KN, et al. Pegylated liposomes have potential as vehicles for intratumoral and subcutaneous drug delivery. Clin Cancer Res. 2000;6:2528–2537.
- Kato K, Omura H, Ishitani R, et al. Cyclic GMP-AMP as an endogenous second messenger in innate immune signaling by cytosolic DNA. Annu Rev Biochem. 2017;86:541–566.
- Koshy ST, Cheung AS, Gu L, et al. Liposomal delivery enhances immune activation by STING agonists for cancer immunotherapy. Adv Biosyst. 2017;1:1600013.
- Liu Y, Crowe WN, Wang L, et al. An inhalable nanoparticulate STING agonist synergizes with radiotherapy to confer long-term control of lung metastases. Nat Commun. 2019;10:5108.
- Cubillos-Ruiz JR, Engle X, Scarlett UK, et al. Polyethylenimine-based siRNA nanocomplexes reprogram tumor-associated dendritic cells via TLR5 to elicit therapeutic antitumor immunity. J Clin Invest. 2009;119:2231–2244.
- He W, Liang P, Guo G, et al. Re-polarizing myeloid-derived suppressor cells (MDSCs) with cationic polymers for cancer immunotherapy. Sci Rep. 2016;6:24506.
- Nikitczuk KP, Schloss RS, Yarmush ML, et al. PLGA-polymer encapsulating tumor antigen and CpG DNA administered into the tumor microenvironment elicits a systemic antigen-specific IFN-γ response and enhances survival. J Cancer Ther. 2013;4:280–290.
- Da Silva CG, Camps MGM, Li TMWY, et al. Effective chemoimmunotherapy by co-delivery of doxorubicin and immune adjuvants in biodegradable nanoparticles. Theranostics. 2019;9:6485–6500.
- Min Y, Roche KC, Tian S, et al. Antigen-capturing nanoparticles improve the abscopal effect and cancer immunotherapy. Nat Nanotechnol. 2017;12:877–882.
- Han HD, Byeon Y, Jang JH, et al. In vivo stepwise immunomodulation using chitosan nanoparticles as a platform nanotechnology for cancer immunotherapy. Sci Rep. 2016;6:38348.
- Li X, Min M, Du N, et al. Chitin, chitosan, and glycated chitosan regulate immune responses: the novel adjuvants for cancer vaccine. Clin Dev Immunol. 2013; 2013:387023.
- Zaharoff DA, Rogers CJ, Hance KW, et al. Chitosan solution enhances both humoral and cell-mediated immune responses to subcutaneous vaccination. Vaccine. 2007;25:2085–2094.
- Tahamtan A, Barati M, Tabarraei A, et al. Antitumor immunity induced by genetic immunization with chitosan nanoparticle formulated adjuvanted for HPV-16 E7 DNA vaccine. Iran J Immunol. 2018;15:269–280.
- Kim TH, Jin H, Kim HW, et al. Mannosylated chitosan nanoparticle-based cytokine gene therapy suppressed cancer growth in BALB/c mice bearing CT-26 carcinoma cells. Mol Cancer Ther. 2006;5:1723–1732.
- Zaharoff DA, Hance KW, Rogers CJ, et al. Intratumoral immunotherapy of established solid tumors with chitosan/IL-12. J Immunother. 2010;33:697–705.
- Zaharoff DA, Hoffman BS, Hooper HB, et al. Intravesical immunotherapy of superficial bladder cancer with chitosan/interleukin-12. Cancer Res. 2009;69:6192–6199.
- Guo L, Yan DD, Yang D, et al. Combinatorial photothermal and immuno cancer therapy using chitosan-coated hollow copper sulfide nanoparticles. ACS Nano. 2014;8:5670–5681.
- Fogli S, Montis C, Paccosi S, et al. Inorganic nanoparticles as potential regulators of immune response in dendritic cells. Nanomedicine (Lond). 2017;12:1647–1660.
- Tsai YS, Chen YH, Cheng PC, et al. TGF-β1 conjugated to gold nanoparticles results in protein conformational changes and attenuates the biological function. Small. 2013;9:2119–2128.
- Nam J, Son S, Ochyl LJ, et al. Chemo-photothermal therapy combination elicits anti-tumor immunity against advanced metastatic cancer. Nat Commun. 2018;9:1074.
- Cha BG, Jeong JH, Kim J. Extra-large pore mesoporous silica nanoparticles enabling co-delivery of high amounts of protein antigen and toll-like receptor 9 agonist for enhanced cancer vaccine efficacy. ACS Cent Sci. 2018;4:484–492.
- Hong X, Zhong X, Du G, et al. The pore size of mesoporous silica nanoparticles regulates their antigen delivery efficiency. Sci Adv. 2020;6:eaaz4462.
- Chen L, Liu J, Zhang Y, et al. The toxicity of silica nanoparticles to the immune system. Nanomedicine (Lond). 2018;13:1939–1962.
- An M, Yu C, Xi J, et al. Induction of necrotic cell death and activation of STING in the tumor microenvironment via cationic silica nanoparticles leading to enhanced antitumor immunity. Nanoscale. 2018;10:9311–9319.
- Zhang Y, Chen H, Wang H, et al. A synergistic cancer immunotherapy nano-system for preventing tumor growth. Chem Eng J. 2020;380:122472.
- Chen Q, Xu L, Liang C, et al. Photothermal therapy with immune-adjuvant nanoparticles together with checkpoint blockade for effective cancer immunotherapy. Nat Commun. 2016;7:13193.
- Chen PM, Pan WY, Wu CY, et al. Modulation of tumor microenvironment using a TLR-7/8 agonist-loaded nanoparticle system that exerts low-temperature hyperthermia and immunotherapy for in situ cancer vaccination. Biomaterials. 2020;230:119629.
- Mühlberger M, Janko C, Unterweger H, et al. Functionalization of T lymphocytes with citrate-coated superparamagnetic iron oxide nanoparticles for magnetically controlled immune therapy. Int J Nanomed. 2019;14:8421–8432.
- Yang Y, Tang J, Abbaraju PL, et al. Hybrid nanoreactors: enabling an off-the-shelf strategy for concurrently enhanced chemo-immunotherapy. Angew Chem Int Ed Engl. 2018;57:11764–11769.
- Zhang N, Song J, Liu Y, et al. Photothermal therapy mediated by phase-transformation nanoparticles facilitates delivery of anti-PD1 antibody and synergizes with antitumor immunotherapy for melanoma. J Control Release. 2019;306:15–28.
- Lu S, Neoh KG, Huang C, et al. Polyacrylamide hybrid nanogels for targeted cancer chemotherapy via co-delivery of gold nanoparticles and MTX. J Colloid Interf Sci. 2013;412:46–55.
- Chen Q, Wang C, Zhang X, et al. In situ sprayed bioresponsive immunotherapeutic gel for post-surgical cancer treatment. Nat Nanotechnol. 2019;14:89–97.
- Hoopes PJ, Wagner RJ, Duval K, et al. Treatment of canine oral melanoma with nanotechnology-based immunotherapy and radiation. Mol Pharm. 2018;15:3717–3722.
- Ito A, Tanaka K, Honda H, et al. Complete regression of mouse mammary carcinoma with a size greater than 15 mm by frequent repeated hyperthermia using magnetite nanoparticles. J Biosci Bioeng. 2003;96:364–369.
- Chen W, Qin M, Chen X, et al. Combining photothermal therapy and immunotherapy against melanoma by polydopamine-coated Al2O3 nanoparticles. Theranostics. 2018;8:2229–2241.
- Cano-Mejia J, Bookstaver ML, Sweeney EE, et al. Prussian blue nanoparticle-based antigenicity and adjuvanticity trigger robust antitumor immune responses against neuroblastoma. Biomater Sci. 2019;7:1875–1887.
- Ito A, Matsuoka F, Honda H, et al. Antitumor effects of combined therapy of recombinant heat shock protein 70 and hyperthermia using magnetic nanoparticles in an experimental subcutaneous murine melanoma. Cancer Immunol Immunother. 2004;53:26–32.
- Zhang X, Wu F, Men K, et al. Modified Fe3O4 magnetic nanoparticle delivery of CpG inhibits tumor growth and spontaneous pulmonary metastases to enhance immunotherapy. Nanoscale Res Lett. 2018;13:240.
- Toraya-Brown S, Sheen MR, Zhang P, et al. Local hyperthermia treatment of tumors induces CD8(+) T cell-mediated resistance against distal and secondary tumors. Nanomedicine. 2014;10:1273–1285.
- Zhou Z, Zhang B, Zai W, et al. Perfluorocarbon nanoparticle-mediated platelet inhibition promotes intratumoral infiltration of T cells and boosts immunotherapy. Proc Natl Acad Sci U S A. 2019;116:11972–11977.
- Steinmetz NF. Viral nanoparticles as platforms for next-generation therapeutics and imaging devices. Nanomedicine. 2010;6:634–641.
- Miermont A, Barnhill H, Strable E, et al. Cowpea mosaic virus capsid: a promising carrier for the development of carbohydrate based antitumor vaccines. Chemistry. 2008;14:4939–4947.
- Wang C, Beiss V, Steinmetz NF. Cowpea mosaic virus nanoparticles and empty virus-like particles show distinct but overlapping immunostimulatory properties. J Virol. 2019;93:e00129-19.
- Murray AA, Wang C, Fiering S, et al. In situ vaccination with cowpea vs tobacco mosaic virus against melanoma. Mol Pharm. 2018;15:3700–3716.
- Lizotte PH, Wen AM, Sheen MR, et al. In situ vaccination with cowpea mosaic virus nanoparticles suppresses metastatic cancer. Nat Nanotechnol. 2016;11:295–303.
- Kerstetter-Fogle A, et al. Plant virus-like particle in situ vaccine for intracranial glioma immunotherapy. Cancers (Basel). 2019;11:515.
- Wang C, Steinmetz NF. CD47 blockade and cowpea mosaic virus nanoparticle in situ vaccination triggers phagocytosis and tumor killing. Adv Healthc Mater. 2019;8:e1801288.
- Czapar AE, Tiu BDB, Veliz FA, et al. Slow-release formulation of cowpea mosaic virus for in situ vaccine delivery to treat ovarian cancer. Adv Sci (Weinh). 2018;5:1700991–1700991.
- Shukla S, Wang C, Beiss V, et al. Antibody response against cowpea mosaic viral nanoparticles improves in situ vaccine efficacy in ovarian cancer. ACS Nano . 2020;14:2994–3003.
- Lee KL, Murray AA, Le DHT, et al. Combination of plant virus nanoparticle-based in situ vaccination with chemotherapy potentiates antitumor response. Nano Lett. 2017;17:4019–4028.
- Lebel MÈ, Chartrand K, Tarrab E, et al. Potentiating cancer immunotherapy using papaya mosaic virus-derived nanoparticles. Nano Lett. 2016;16:1826–1832.
- Pokorski JK, Steinmetz NF. The art of engineering viral nanoparticles. Mol Pharm. 2011;8:29–43.
- Farley MM, Hu B, Margolin W, et al. Minicells, back in fashion. J Bacteriol. 2016;198:1186–1195.
- MacDiarmid JA, Mugridge NB, Weiss JC, et al. Bacterially derived 400 nm particles for encapsulation and cancer cell targeting of chemotherapeutics. Cancer Cell. 2007;11:431–445.
- Schwechheimer C, Kuehn MJ. Outer-membrane vesicles from Gram-negative bacteria: biogenesis and functions. Nat Rev Microbiol. 2015;13:605–619.
- Jivrajani M, Nivsarkar M. Ligand-targeted bacterial minicells: futuristic nano-sized drug delivery system for the efficient and cost effective delivery of shRNA to cancer cells. Nanomedicine. 2016;12:2485–2498.
- Zhang Y, Ji W, He L, et al. E. coli nissle 1917-derived minicells for targeted delivery of chemotherapeutic drug to hypoxic regions for cancer therapy. Theranostics. 2018;8:1690–1705.
- Tsuji S, Chen X, Hancock B, et al. Preclinical evaluation of VAX-IP, a novel bacterial minicell-based biopharmaceutical for nonmuscle invasive bladder cancer. Mol Ther Oncolytics. 2016;3:16004–16004.
- MacDiarmid JA, Langova V, Bailey D, et al. Targeted doxorubicin delivery to brain tumors via minicells: proof of principle using dogs with spontaneously occurring tumors as a model. PLoS One. 2016;11:e0151832.
- MacDiarmid JA, Madrid-Weiss J, Amaro-Mugridge NB, et al. Bacterially-derived nanocells for tumor-targeted delivery of chemotherapeutics and cell cycle inhibitors. Cell Cycle. 2007;6:2099–2105.
- Solomon BJ, Desai J, Rosenthal M, et al. A first-time-in-human phase I clinical trial of bispecific antibody-targeted, paclitaxel-packaged bacterial minicells. PLoS One. 2015;10:e0144559.
- Carleton HA, Lara-Tejero M, Liu X, et al. Engineering the type III secretion system in non-replicating bacterial minicells for antigen delivery. Nat Commun. 2013;4:1590–1590.
- Hancock BM, McGuire KL, Tsuji S, et al. A single intravesical instillation of VAX014 inhibits orthotopic superficial bladder tumor implantation to increase survival. Anticancer Res. 2016;36:6243–6248.
- Karagiannis ED, Anderson DG. Minicells overcome tumor drug-resistance. Nat Biotechnol. 2009;27:620–621.
- Singh MP, Sethuraman SN, Ritchey J, et al. In-situ vaccination using focused ultrasound heating and anti-CD-40 agonistic antibody enhances T-cell mediated local and abscopal effects in murine melanoma. Int J Hyperthermia. 2019;36:64–73.
- Beg S, Kawish SM, Panda SK, et al. Nanomedicinal strategies as efficient therapeutic interventions for delivery of cancer vaccines. Semin Cancer Biol. 2019.
- Zhang R, Billingsley MM, Mitchell MJ. Biomaterials for vaccine-based cancer immunotherapy. J Control Release. 2018;292:256–276.
- Young JK, Figueroa ER, Drezek RA. Tunable nanostructures as photothermal theranostic agents. Ann Biomed Eng. 2012;40:438–459.
- Zhang Z, Wang J, Chen C. Near-infrared light-mediated nanoplatforms for cancer thermo-chemotherapy and optical imaging. Adv Mater Weinheim. 2013;25:3869–3880.
- Zhou F, Li X, Naylor MF, et al. InCVAX-a novel strategy for treatment of late-stage, metastatic cancers through photoimmunotherapy induced tumor-specific immunity. Cancer Lett. 2015;359:169–177.
- Zhang Z, Wang J, Nie X, et al. Near infrared laser-induced targeted cancer therapy using thermoresponsive polymer encapsulated gold nanorods. J Am Chem Soc. 2014;136:7317–7326.
- Wang C, Xu L, Liang C, et al. Immunological responses triggered by photothermal therapy with carbon nanotubes in combination with anti-CTLA-4 therapy to inhibit cancer metastasis. Adv Mater Weinheim. 2014;26:8154–8162.
- Bear AS, Kennedy LC, Young JK, et al. Elimination of metastatic melanoma using gold nanoshell-enabled photothermal therapy and adoptive T cell transfer. PloS One. 2013;8:e69073.
- Cano-Mejia J, Burga RA, Sweeney EE, et al. Prussian blue nanoparticle-based photothermal therapy combined with checkpoint inhibition for photothermal immunotherapy of neuroblastoma. Nanomedicine. 2017;13:771–781.
- Duval KEA, Vernice NA, Wagner RJ, et al. Immunogenetic effects of low dose (CEM43 30) magnetic nanoparticle hyperthermia and radiation in melanoma cells. Int J Hyperthermia. 2019;36:37–46.
- Ito A, Shinkai M, Honda H, et al. Heat shock protein 70 expression induces antitumor immunity during intracellular hyperthermia using magnetite nanoparticles. Cancer Immunol Immunother. 2003;52:80–88.
- Tanaka K, Ito A, Kobayashi T, et al. Intratumoral injection of immature dendritic cells enhances antitumor effect of hyperthermia using magnetic nanoparticles. Int J Cancer. 2005;116:624–633.
- Kobayashi T, Kakimi K, Nakayama E, et al. Antitumor immunity by magnetic nanoparticle-mediated hyperthermia. Nanomedicine (Lond)). 2014;9:1715–1726.
- Oei AL, Korangath P, Mulka K, et al. Enhancing the abscopal effect of radiation and immune checkpoint inhibitor therapies with magnetic nanoparticle hyperthermia in a model of metastatic breast cancer. Int J Hyperthermia. 2019;36:47–63.
- Ranjan A, Jacobs GC, Woods DL, et al. Image-guided drug delivery with magnetic resonance guided high intensity focused ultrasound and temperature sensitive liposomes in a rabbit Vx2 tumor model. J Controlled Release. 2012;158:487–494.
- Ektate K, Munteanu MC, Ashar H, et al. Chemo-immunotherapy of colon cancer with focused ultrasound and Salmonella-laden temperature sensitive liposomes (thermobots). Sci Rep. 2018;8:13062.
- VanOsdol J, Ektate K, Ramasamy S, et al. Sequential HIFU heating and nanobubble encapsulation provide efficient drug penetration from stealth and temperature sensitive liposomes in colon cancer. J Control Release. 2017;247:55–63.
- Skalina KA, Singh S, Chavez CG, et al. Low intensity focused ultrasound (LOFU)-mediated acoustic immune priming and ablative radiation therapy for in situ tumor vaccines. Sci Rep. 2019;9:15516.
- Silvestrini MT, Ingham ES, Mahakian LM, et al. Priming is key to effective incorporation of image-guided thermal ablation into immunotherapy protocols. JCI Insight. 2017;2:e90521.
- Eranki A, Farr N, Partanen A, et al. Boiling histotripsy lesion characterization on a clinical magnetic resonance imaging-guided high intensity focused ultrasound system. PloS ONE. 2017;12:e0173867.
- Suzuki R, Namai E, Oda Y, et al. Cancer gene therapy by IL-12 gene delivery using liposomal bubbles and tumoral ultrasound exposure. J Control Release. 2010;142:245–250.
- Chen YL, Wang CY, Yang FY, et al. Synergistic effects of glycated chitosan with high-intensity focused ultrasound on suppression of metastases in a syngeneic breast tumor model. Cell Death Dis. 2014;5:e1178.
- Wei SC, Duffy CR, Allison JP. Fundamental mechanisms of immune checkpoint blockade therapy. Cancer Discov. 2018;8:1069–1086.
- Francis DM, Thomas SN. Progress and opportunities for enhancing the delivery and efficacy of checkpoint inhibitors for cancer immunotherapy. Adv Drug Deliv Rev. 2017;114:33–42.
- Barrueto L, Caminero F, Cash L, et al. Resistance to checkpoint inhibition in cancer immunotherapy. Transl Oncol. 2020;13:100738.
- Ajina R, Zahavi DJ, Zhang YW, et al. Overcoming malignant cell-based mechanisms of resistance to immune checkpoint blockade antibodies. Semin Cancer Biol. 2019;65:28–37.
- Gong J, Hendifar A, Tuli R, et al. Combination systemic therapies with immune checkpoint inhibitors in pancreatic cancer: overcoming resistance to single-agent checkpoint blockade. Clin Transl Med. 2018;7:32.
- Fay AP, Antonarakis ES. Blocking the PD-1/PD-L1 axis in advanced prostate cancer: are we moving in the right direction?. Ann Transl Med. 2019;7:S7.
- Luo L, Zhu C, Yin H, et al. Laser immunotherapy in combination with perdurable PD-1 blocking for the treatment of metastatic tumors. ACS Nano. 2018;12:7647–7662.
- Tselikas L, de Baere T, Isoardo T, et al. Pickering emulsions with ethiodized oil and nanoparticles for slow release of intratumoral anti-CTLA4 immune checkpoint antibodies. J Immunother Cancer. 2020;8:e000579.