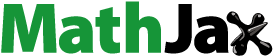
Abstract
Introduction
Metastatic colorectal cancer (CRC) is complicated by chemotherapy-resistant cell populations. Oxaliplatin is used in heated intraperitoneal hyperthermic chemoperfusion (HIPEC) for treatment of disseminated CRC. Photothermal nanoparticles can provide focal heating to improve the response of CRC cells to oxaliplatin, by confining heating near individual cells. Reduction in cellular luciferase signal may allow single-cell-resolution recording of thermal dosimetry.
Methods
Oxaliplatin resistant (OxR) variants of luciferase-expressing CT26.WT-Fluc-Neo CRC cells were developed and their sensitivity to hyperthermia was evaluated. Polymer-based photothermal nanoparticles were developed, characterized and used to explore their potential for imparting a thermal dose to improve cell response to oxaliplatin. A correlation of thermal dose to intracellular luciferase activity was established using quantitative luminescence monitoring and microscopy.
Results
Luciferase-based monitoring of thermal dose within CT26 cell lines was validated within the ranges of 0.04–8.33 CEM43 for parental cells and 0.05–9.74 CEM43 for OxR CT26 cells. This was further confirmed using nanoparticle-induced hyperthermia, where the single-cell resolution of the thermal dose can be achieved. The nanoparticles enhance cell killing of resistant cells when combined with oxaliplatin and stimulated to generate heat.
Conclusion
Nanoparticle-based hyperthermia is effective for augmenting chemotherapy and can be coupled with reductions in CT26 luciferase expression to monitor thermal dose at single-cell resolution. The development of OxR CT26.WT-Fluc-Neo CRC cells sets the stage for pre-clinical evaluations to measure nanoparticle-induced hyperthermia to augment chemotherapy (Nano-HIPEC) in a chemotherapy-resistant model of disseminated CRC.
Graphical Abstract
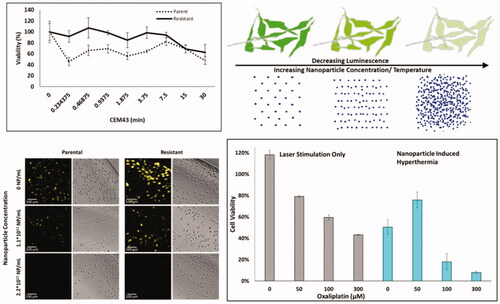
Introduction
Metastatic colorectal cancer (CRC) is a disease with few available treatments, displaying a 5-year survival of 14.2% [Citation1,Citation2]. Standard-of-care treatment for metastatic CRC is cytoreduction with systemic chemotherapy, although this is often considered palliative instead of curative [Citation3]. In the case of disseminated disease that is isolated to the peritoneal cavity, Hyperthermic Intraperitoneal Chemoperfusion (HIPEC) may be used. HIPEC involves surgical cytoreduction to remove macroscopic nodules followed immediately by intraperitoneal perfusion of heated chemotherapy (40–43 °C) at concentrations higher than allowed with systemic delivery [Citation4,Citation5]. Exposure to hyperthermia alone for 30 min or more induces apoptosis in cancer cells when restricted to below 45 °C (mild hyperthermia) and the addition of hyperthermia to chemotherapy augments the treatment [Citation6,Citation7]. In the case of disseminated peritoneal carcinomatosis of CRC (pcCRC), prior clinical studies indicate HIPEC to be beneficial over both cytoreduction alone and cytoreduction with systemic chemotherapy [Citation8].
The introduction of the platinum compound oxaliplatin into CRC treatment has expanded CRC’s therapeutic library, where pre-clinical studies have shown that hyperthermia and oxaliplatin interact synergistically to kill cancer cells and reduce overall tumor burden [Citation9,Citation10]. In spite of its promises, the recent completion of the PRODIGE 7 clinical trial investigating HIPEC regimens with oxaliplatin yielded no conclusive evidence to support HIPEC over standard cytoreduction with systemic chemotherapy in cases of colorectal pcCRC [Citation11]. Some skepticism of the study remains, particularly surrounding the limitation of the HIPEC perfusion time to only 30 min, where it is believed that HIPEC with oxaliplatin may still have a beneficial effect given sufficient perfusion time [Citation12]. In contrast to the PRODIGE 7 trial, a randomized clinical trial reporting on the use of HIPEC with cisplatin in combination with cytoreductive surgery for treatment of ovarian cancer metastases in the peritoneal cavity demonstrated a significantly longer disease-free and overall survival for patients who received HIPEC vs those who received surgery and chemotherapy alone [Citation13]. Notably, the ovarian cancer HIPEC trial involved a perfusion time of 90 min, supporting the conjecture that longer exposure to hyperthermia may be needed to for maximal benefits. Improvements in preclinical in vivo models to monitor thermal dose-dependent effects may be beneficial for the optimization of HIPEC.
One complication that may need to be overcome to achieve effective HIPEC with oxaliplatin is resistant relapse. Following the initial treatment of metastatic CRC, the relapse of undetected and insufficiently treated disease is a common occurrence, with some CRCs hypothesized to have acquired chemoresistance following the initial chemotherapeutic insult [Citation14]. Patient survival following HIPEC is complicated by the relapse of resistant disease; therefore, it is important to understand how oxaliplatin and hyperthermia interact with oxaliplatin-resistant (OxR) sub-populations [Citation15,Citation16]. Various single-institution studies indicate the use of cytoreductive surgery + HIPEC as yielding significant increases in overall survival compared to palliative treatment or no treatment [Citation8,Citation17]. While no studies investigating HIPEC treatment of CRC report chemoresistance, several studies investigating HIPEC for pcCRC of epithelial ovarian cancer report similar survival outcomes in patients with platinum-resistant recurrence relative to platinum-sensitive cases [Citation17]. Stemming from these results in CRC and other peritoneal malignancies, it is important to develop a pre-clinical model to investigate the utility of hyperthermia with oxaliplatin against OxR CRC.
More localized and effective hyperthermia can be achieved with heat-generating-nanoparticles compared to direct heating of the bulk carrier fluid by heat exchanger [Citation18–20]. A recent study has shown that rapid hyperthermia generated by photothermal nanoparticles (nanoparticles capable of generating heat following light stimulation) can achieve increased cell killing in thermotolerant breast cancer stem cells compared to bulk heating [Citation21]. It has been demonstrated that the combination of photothermal-nanoparticle-mediated hyperthermia (42 °C) for 10 s with oxaliplatin achieved equivalent CRC cell killing and chemotherapy internalization as 2 h of incubator-mediated hyperthermia (42 °C) with simultaneous oxaliplatin [Citation22]. Though still in the pre-clinical stage, the capacity to target heat-generating photothermal nanoparticles for rapid delivery of hyperthermia exclusively to cancer cells represents the next generation of thermal therapy. Our lab has previously developed dual fluorescent/photothermal polymer nanoparticles by combining two semiconducting donor-acceptor polymers within a single nanoparticle: the fluorescent polymer poly[(9,9-dihexylfluorene)-co-2,1,3-benzothiadiazole-co-4,7-di(thiophen-2-yl)-2,1,3-benzothiadiazole] (PFBTDBT10) and the photothermal polymer poly[4,4-bis(2-ethylhexyl)-cyclopenta[2,1-b;3,4-b’]dithiophene-2,6-diyl-alt-2,1,3-benzoselenadiazole-4,7-diyl] (PCPDTBSe), which is capable of producing heat following near-infrared (NIR) stimulation [Citation23]. These Hybrid Donor-Acceptor Polymer Particles (HDAPPs), when excited by 464 nm light fluorescence emission at 825 nm, and heat when photoexcited using 800 nm [Citation24].
The precise measurement of the nanoparticle-induced thermal dose is a complicated process due to the underestimation of nanoscale vs. bulk temperatures [Citation25–27]. In the current work, we explored the option that the location of the nanoparticles external to the cells is in close enough proximity that bulk heating of the medium by the particles is considered to be equivalent to intracellular temperatures. The thermal dose is commonly measured by Cumulative Equivalent Minutes at 43 °C (CEM43), where a tissue’s duration of temperature exposure is mapped to the time required at 43 °C to achieve an equivalent reduction in viability (termed isoeffect) [Citation28,Citation29]. This is performed according to the formula where t is time in minutes, R is a correction for the number of minutes required to achieve an isoeffect for each degree step away from 43 °C (R = 0.25 when T < 43 °C and R = 0.5 when T > 43 °C), and T is the temperature. When photothermal nanoparticles are used heat is generated rapidly (sometimes in under 1 min), with rapid thermal variations over the time of application. In this case, it is most appropriate to calculate CEM 43 over intervals where the temperature is relatively constant and sum the entirety of CEM43 values to obtain the calculated thermal dose [Citation30–32]. A correlational method of measuring thermal dose using intracellular luciferase-based luminescence signal decay has been previously reported [Citation33]. The combination of this technique with bioluminescence imaging at single-cell-resolution indicates the potential for recording thermal dosimetry from photothermal nanoparticles within a single CRC cell, as described in the present work using the oxaliplatin-resistant mouse (CT26) colorectal cancer cell in investigating hyperthermia and oxaliplatin combination therapy.
Materials and methods
Materials
1X Dulbecco’s Modified Eagle Medium (DMEM) supplemented with 4.5 g/L D-glucose, L-glutamine (1X), 110 mg/mL sodium pyruvate, penicillin and streptomycin (1X), 10% fetal bovine serum, and with or without 400 µg/mL G418 (neomycin) was purchased from Gibco. 1X McCoys 5 A Medium supplemented with L-glutamine (1X), penicillin and streptomycin (1X), and 10% fetal bovine serum was purchased from Gibco. Fluorobrite DMEM supplemented with 4.5 g/L D-glucose, L-glutamine (1X), 110 mg/mL sodium pyruvate, penicillin and streptomycin (1X), and 10% fetal bovine serum was purchased from Gibco. Oxaliplatin was purchased from Sigma (O9512) and reconstituted in Milli-Q grade water for a maximum storage duration of 6 months at 4 °C in the dark. CellTiter 96 AQueous One Solution Cell Proliferation Assay (MTS) was purchased from Promega (G3580). XenoLight D-Luciferin - K + Salt Bioluminescent Substrate was purchased from Perkin Elmer (122799).
4H-cyclopenta[1,2-b:5,4-b’]dithiophene, 4,7-Dibromo-2,1,3-benzoselenadiazole, 4,7-bis(5-bromo-2-thienyl)-2,1,3-benzothiadiazole, and 2,7-Bis(4,4,5,5-tetramethyl-1,3,2-dioxaborolan-2-yl)-9,9-dihexylfluorane were purchased from TCI America. Tetraethylammonium hydroxide, 4,7-dibromo-2,1,3-benzothiadiazole, 4,7-Bis(2-bromo-5-thienyl)-2,1,3-benzothiadiazole, and tetrakis(triphenylphosphine)palladium(0) were purchased from Sigma Aldrich. Toluene was purchased from Fisher Chemical Co. Tetrahydrofuran (THF) was purchased from Acros Organics. 1,2-distearoyl-sn-glycero-3-phosphoethanolamine-polyethylene glycol-OH (DSPE-PEG-OH, MW 2000) was purchased from Nanocs.
CT26.WT-Fluc-Neo, mouse colorectal carcinoma cells with transduced LV-Fluc-P2A-Neo (LV011), a firefly luciferase gene flanked by a neomycin resistance gene, were purchased from Imanis Life Sciences (CL043). Cells were cultured in a 37 °C, 5% CO2 humidified atmosphere and regularly passaged at 70–90% confluency.
Determining oxaliplatin half-maximal inhibitory concentrations (IC50)
CT26.WT-Fluc-Neo cells were plated in 200 µL of DMEM media at 40,000 cells/well in a 96-well tissue-culture-treated plate. Cells were allowed to adhere for 24 h, after which they were exposed to the following oxaliplatin concentrations in triplicate for 72 h: 0, 0.1, 0.5, 1, 2.5, 5, 10, 20, 30, 50, 100 and 300 µM (which is the maximum concentration used clinically in HIPEC) [Citation34]. After treatment, cells were immediately assayed for viability using CellTiter 96 Aqueous One Solution Cell Proliferation Assay (Promega) read at 490 nm on a TECAN infinite M2000 plate reader as described by Jensen et al. [Citation35] Viability as a function of log(oxaliplatin concentration) was fit to a 4-parameter logistic model in custom software to determine the IC50 of oxaliplatin.
Induction of oxaliplatin chemoresistance
A previously described protocol was used to develop oxaliplatin resistance within the CT26.WT-Fluc-Neo line [Citation36,Citation37]. IC50 values of oxaliplatin were monitored periodically throughout the development process. Parental CT26.WT-Fluc-Neo cells were initially seeded at 10,000 cells in DMEM + G418 medium in a T-25 flask and allowed to adhere. Cells were then treated with oxaliplatin at the previously calculated IC50 dose of 6.0 µM, or exposed to no oxaliplatin for 72 h. Following treatment, cells were washed once with 1X PBS and maintained in oxaliplatin-free media. Thirty-six hours later the media was replaced to remove any potential oxaliplatin released by dead cells. Cells were allowed to recover for an additional 36 h, after which they were trypsinized and replated at 10,000 cells/well. Twenty-four hours following this plating cells were treated with oxaliplatin or no oxaliplatin in the aforementioned manner. This process was repeated in a weekly manner for 420 days, at which point cells were found to have reached peak resistance.
Determination of resistance duration as a function of passage length
One question we sought to answer was to determine whether chemoresistant cells could retain their resistance to oxaliplatin over time. To accomplish this CT26.WT-Fluc-Neo-OxR and CT26.WT-Fluc-Neo parental cells were cultured for 5 weeks without oxaliplatin and then were plated at 6000 cells/well in a 96-well tissue-culture-treated plate. Cells were allowed to adhere for 24 h, after which they were exposed to 0, 0.1, 0.5, 1, 2.5, 5, 10, 20, 30, 50, 100 and 300 µM oxaliplatin for 72 h. After treatment, cells were immediately assayed for viability using CellTiter 96 Aqueous One Solution Cell Proliferation Assay (Promega) and read at 490 nm on a TECAN infinite M2000 plate reader. Viability as a function of log(oxaliplatin concentration) was fit to a 4-parameter logistic model in custom software to determine the IC50 of oxaliplatin.
Luminescent monitoring of cell effective thermal dose in parental and resistant CT26 cells
Thermal dose in parental and OxR CT26 cells was correlated to the loss of intracellular luciferase-based luminescence activity, as has been reported previously [Citation33]. CT26.WT-Fluc-Neo-OxR and CT26.WT-Fluc-Neo (parental) cells were plated with 10 replicates in 200 µL DMEM at 6000 cells/well in a 96-well tissue-culture-treated transparent plate pre-coated with poly-L-Lysine to aid in cell adherence. Cells were allowed to adhere for 24 h, after which they were exposed to hyperthermia in a heat block (Fisher Scientific) in a manner similar to that described by Mukherjee et al. [Citation38] Prior to use with cells the heat block was evaluated to measure temperatures of a 200 µL volume in the wells of a tissue culture plate, and it was found that there was a lag time for the heating to 42 °C of about 8 min. Thus each plate was heated at 37 or 40 °C for 1, 3, 5, 15, 30 and 60 min, or 42 °C for 15, 30, and 60 min due to heating lag. Immediately after treatment, cell media was replaced with 0.1 mL of 150 µg/mL luciferin in 1X PBS. Cells were allowed to incubate at 37 °C for 10 min, after which luminescence activity was measured using a Molecular Devices FilterMax F5 Milti-Mode Microplate Reader (1000 ms integration time). Cell luminescence was corrected using blank wells with poly-L-lysine coating and containing no cells. Additional populations of parental and OxR cells were also exposed to 37, 40, 42 or 44 °C for varying times, up to 120 min, and subsequently, cell viability was determined 24 h after hyperthermia using CellTiter 96 Aqueous One Solution Cell Proliferation Assay (Promega) read at 490 nm on a TECAN infinite M2000 plate reader. Thermal dose was calculated using the cumulative equivalent minutes at 43 °C (CEM43) unit: where t is time in minutes, R is a correction for the number of minutes required to achieve an isoeffect for each degree step away from 43 °C (R = 0.25 when T < 43 °C and R = 0.5 when T > 43 °C), and T is the temperature [Citation28,Citation29]. The temperatures of 37, 40 and 42 C were used to correspond with the heating block temperatures.
Demonstration of luminescent monitoring of cell effective thermal dose using photothermal nanoparticles
Our lab has previously developed HDAPPs photothermal nanoparticles composed of electrically conductive donor-acceptor polymers to serve as theranostic agents for fluorescent imaging and thermal ablation of cancer [Citation23]. HDAPPs coated with the amphiphilic surfactant DSPE-PEG-OH were formed according to the following protocol. Poly[(9,9-dihexylfluorene)-co-2,1,3-benzothiadiazole] (PFBTDBT10) and poly[4,4-bis(2-ethylhexyl)-cyclopenta[2,1-b;3,4-b’]dithiophene-2,6-diyl-alt-2,1,3-benzoselenadiazole-4,7-diyl] (PCPDTBSe) polymers were synthesized as described previously [Citation23]. A mixture of PFBTDBT10 (0.93 mg/mL) and PCPDTBSe (0.1 mg/mL) in 1 mL of tetrahydrofuran (THF) was rapidly injected into an 8 mL solution of 1 mg/mL DSPE-PEG-OH under horn sonication (1 min, 20% amplitude). Following nanoprecipitation, THF was evaporated off under vacuum (11 mmHg subatmospheric) and heating (55 °C) for 6 h. HDAPPs were then isolated by centrifugation at 5550 rcf for 30 min to remove aggregates, followed by centrifugation at 16,800 rcf for 4 h to yield concentrated HDAPPs. To sterilize, HDAPPs were exposed to UV light for 15 min.
Following synthesis, HDAPPs were characterized using ultraviolet-visible (UV/Vis) light spectroscopy (Mettler Toledo UV5Nano), fluorescence spectroscopy (TECAN infinite M2000), nanoparticle tracking analysis (NTA) using 405 nm laser in both scattering and fluorescence modes (Nanosight NS500), and dynamic light scattering (DLS) and zeta potential (Malvern Nano-ZS). Additionally, HDAPPs heat generation versus concentration was determined by exposing HDAPPs in water (200 μL) to 800 nm continuous wave (CW) laser at 3 W for 60 s with a 1 cm2 spot size (K-Cube, Summus Medical Laser). The dilutions used were as follows: 2.2 × 1012, 1.1 × 1012, 4.39 × 1011, 1.1 × 1011, and 0 NP/mL, where these concentrations were chosen to generate a range of thermal doses. Solution temperatures before and after laser stimulation were recorded using a thermocouple (Fluke 51 K/J Thermometer). To evaluate how the temperature of the nanoparticle solutions evolves over time the temperature change during a 60 s laser exposure was measured in 200 µL volumes of 2.2 × 1011, 1.54 × 1011, 1.11 × 1011, 4.39 × 1010, and 0 NP/mL using a NeoOptix fiber optic thermocouple. Since the time for photothermal treatment was short, CEM43 was calculated two ways and the calculations were compared to evaluate the accuracy with the luciferase correlation of thermal dose. First, CEM43 was calculated using the average solution over the 60 s laser stimulation period. Alternatively, CEM43 was calculated based on the average temperature of the solution over 1, 5, or 10 s time intervals over the 60 s laser exposure/heating time, and the cumulative CEM43 was calculated. HDAPPS’ photothermal conversion efficiency was determined using the method described by Roper et al. [Citation39]
Next, CT26 parental and OxR cells were plated in triplicate at 6000 cells/well in 200 µL Fluorobrite DMEM in a 96-well tissue-culture-treated transparent plate pre-coated with poly-L-Lysine. Cells were allowed to adhere for 24 h, after which cells were exposed to HDAPPs (2.2 × 1011, 1.54 × 1011, 1.1 × 1011, 4.39 × 1010, or 0 NP/mL). The purpose of this study was to evaluate HDAPPs mediated photothermal therapy using nanoparticles in suspension around the cells. Therefore, there was no cell uptake of the HDAPPs and after their addition, cells were immediately subjected to 800 nm 3 W, CW laser for 60 s with a 1 cm2 spot size while maintaining a baseline temperature of 37 °C. Immediately after treatment, cells were washed twice with HEPES buffered saline (250 mM) + 0.1% bovine serum albumin (BSA) and the media was replaced with 0.1 mL of 150 µg/mL luciferin in 1X PBS. Cells were allowed to incubate at 37 °C for 10 min, after which luminescence activity was measured using a Molecular Devices FilterMax F5 Multi-Mode Microplate Reader (1000 ms integration time). Cell luminescence was corrected using blank wells coated with poly-L-lysine coated wells and containing no cells. Luminescence was normalized within each concentration to the relative luminescence at 37 °C for cells treated with equivalent concentrations of HDAPPs but no laser (NL). Cell viability was quantified immediately after laser exposure, and the second set of plates was used to quantify cell viability 24 h after laser exposure, using CellTiter 96 Aqueous One Solution Cell Proliferation Assay (Promega) read at 490 nm on a TECAN infinite M2000 plate reader. To achieve cells treated with HDAPPs induced hyperthermia were imaged 10 min after incubation with luciferin at 37 °C. A microscope fit with an electron-multiplying-CCD (EM-CCD) detector and with no filter so that all emission wavelengths could be captured (although the peak luminescence was around 560 nm) (iXon, Andor Inc) (200 gain, 60 s exposure, 10× objective) was used. Images were subdivided into 9 equal-area squares, from which 10 representative adherent cells in each square were selected using a 112-pixel circle to obtain the average cell pixel intensity using ImageJ.
Response of parental and OxR CRC cells to nanoparticle induced hyperthermia with oxaliplatin
To determine the sensitivity of parental and chemoresistant cell lines to nanoparticle-induced hyperthermia, oxaliplatin, or their combination, parental and OxR cells were plated in 200 µL DMEM at 6000 cells/well in a 96-well tissue-culture-treated transparent plate, where the wells were coated with poly-L-Lysine. Cells were allowed to adhere for 24 h, after which they were exposed to oxaliplatin (0, 50, 100 and 300 µM) in 200 µL culture media with or without 2.2 × 1011 NP/mL at 37 °C for 2 h in triplicate. During this incubation, the cells were exposed to 60 s of 3 W/cm2 of 800 nm CW light three times, for a total of three temperature increases during three separate exposures, with 20 min allowed for a return to 37 °C. During laser exposure, the cell temperature was maintained at 37 °C by placing the plate in a heat block held at 37 °C. Immediately after treatment, cells were washed twice with HEPES buffered saline (250 mM) + 0.1% bovine serum albumin (BSA) and the media was replaced with 0.1 mL of 150 µg/mL luciferin in 1X PBS. Cells were allowed to incubate at 37 °C for 10 min, after which luminescence activity was measured. Following luminescence measurements, the luciferase was removed and the cells were incubated for an additional 24 h in drug-free media, after which luminescence was measured again and then cell viability was assayed using CellTiter 96 Aqueous One Solution Cell Proliferation Assay (Promega) read at 490 nm on a TECAN infinite M2000 plate reader.
Statistical methods
All data were analyzed using SigmaStat. Two-way analysis of variance (ANOVA), with factors of laser status and HDAPPs concentration, was used to examine proliferation. Additionally, two way ANOVA with factors of laser status and oxaliplatin concentration (with or without HDAPPs) was used to examine their effects on proliferation. Pairwise comparison for two way ANOVA tests was performed using the Holm–Sidak method. Cell average pixel intensities as a function of HDAPPs concentration were analyzed by one way ANOVA. Kruskal-Wallis one-way analysis of variance (ANOVA) on ranks was used to examine the effect of concentration on individual cell luminescence within parental and resistant cell populations (n = 90). A pairwise comparison was performed using a Tukey Test. An alpha level of 0.05 was used for all tests.
Results
Induction of oxaliplatin chemoresistance
Parental CT26 cells showed baseline oxaliplatin IC50 sensitivity of 6.0 µM, as shown in . Following induction of resistance by weekly intermittent treatment, the OxR cells IC50 value was 48.0, µM. The OxR variants demonstrate an order of magnitude increase in IC50 values. Passage of CT26-OxR cells for 5 weeks in the absence of oxaliplatin resulted in the loss of acquired resistance (IC50 = 18.5 µM), indicating that continued selective pressure is necessary to retain the platinum-resistant phenotype in OxR cells [Citation40,Citation41]. The viability of parental and OxR cells at increasing thermal doses are provided in , and indicate that at lower CEM43 values there are differences between the OxR and parental groups, with the OxR cells being more resistant to hyperthermia.
Figure 1. (A) IC50 values of oxaliplatin in OxR CT26 cells as a function of induction time. At approximately 400 days of intermittent treatment, the following IC50 value of CT26-OxR was 48.0 µM, accounting for a near order-of-magnitude increase. (B) Cell viability of parental and OxR CT26 cells in response to increasing thermal dose. ‘*’ indicates a significant difference in viability between the parental and resistant populations. (C) Loss of luminescence (normalized) as a function of treatment time at 37, 40, and 42 °C in CT26 parental and OxR (D) cells. Translation of treatment time and temperature to CEM43 and plotting relative to the loss of luminescence (normalized) shows valid applicable ranges for the luminescence-intensity correlation from 0.04-8.33 CEM43 (Parental) (E) and 0.05–9.74 CEM43 (OxR) (F), where corresponding normalized RLU values are between 1 and 0.
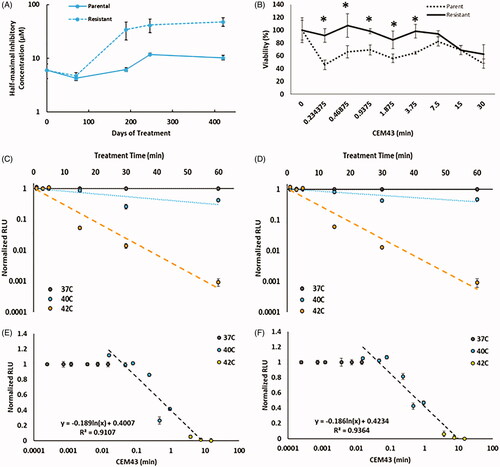
Luminescent monitoring of cell effective thermal dose in parental and resistant CT26 cells
The experimental measurement of thermal dose in cell populations using intracellular luciferase activity has been previously reported as a method to accurately measure hyperthermia [Citation33]. We validated this previous finding and demonstrated its utility in both the parental and OxR variants of CT26 CRC cells. As seen in , plots of normalized relative luminescence (RLU) vs. treatment time demonstrate a probable breakpoint (temperature above which thermotolerance no longer develops) around 42 °C in both parental and OxR cell lines, as marked by a sharp decrease in luminescence activity. When each treatment time and the temperature is shown in is translated to its equivalent CEM43 value, it can be seen that a logarithmic fit of normalized RLU as a function of CEM43 (only using 40 and 42 °C) is strongly correlated (). Here, 91% (for 40 °C) and 94% (for 42 °C) of the measurement precision is fit using the thermal dose as the explanatory variable for the loss in luminescence within parental and OxR cells. The valid range over which this method can record thermal dose is 0.04–8.33 CEM43 for CT26 parental cells and 0.05–9.74 CEM43 for CT26-OxR cells.
Demonstration of luminescent monitoring of cell effective thermal dose using photothermal nanoparticles
HDAPPs were utilized to evaluate photothermal-nanoparticle-mediated hyperthermia with luciferase-based dosimetry in both CT26 parental and OxR cells. Absorption and fluorescence of HDAPPs are shown in , where HDAPPs display a fluorescence quantum yield of 0.63%, which is sufficient to detect through 6 mm of tissue as shown by previous work in both tissue phantoms and in vivo [Citation42,Citation43]. The low quantum yield is indicative of a higher photothermal conversion efficiency (PCE), which was determined to be 51.2%. This PCE of HDAPPs is better than other conjugated polymer nanoparticles, such as polyaniline (48.5%), polypyrrole (44.7%), and melanin (40%), and in alignment with gold nanoparticles which have PCEs ranging from 30-95%, depending upon their size [Citation44–46]. Average HDAPPs diameters, as measured by DLS and NTA (scattering mode), were 96.7 nm and 80.2 nm, respectively (). Sizing between DLS and NTA shows good agreement with a relatively unimodal distribution. Average particle diameter measured using NTA in fluorescence mode indicates a particle size of 82.5 nm, in strong agreement with the scattering analysis (). This serves as confirmatory evidence that HDAPPs can be quantified with NTA to determine nanoparticle concentration in particles/mL as a function of both scattering and fluorescence. The Zeta potential measurements indicate an average surface charge of −33.2 mV. Measurement of heating potential demonstrates HDAPPs’ capacity to induce hyperthermia within both the mild and ablative (T > 45 °C) regimens depending upon the concentration (). As the aim of using HDAPPs induced heating will eventually be to augment chemotherapy, lower concentrations of nanoparticles were used in cell experiments to induce mild hyperthermia. To evaluate the temperatures that cells incubated with HDAPPs experience when exposed to 800 nm light demonstrates the temperature increases that occur during the 60 s laser exposure. The average temperature rise, over baseline (37 °C), that the cells experience are provided in .
Figure 2. Measurement of HDAPPs absorbance and fluorescence (A) spectra shows retention of the characteristic HDAPPs absorbance and fluorescence before and after UV-sterilization. Frequency plots of nanoparticle size measured by NTA in scatter (B) and fluorescence (C) modes show good agreement in HDAPPs size, 80.2 and 82.5 nm, respectively. (D) Measurement of photothermal heat generation reveals HDAPPs maintain the capacity to reach ablative potential (ΔT = 8 °C) at relatively low concentrations. (E) Increasing concentrations of HDAPPs during laser exposure demonstrate progressive changes in solution temperature.
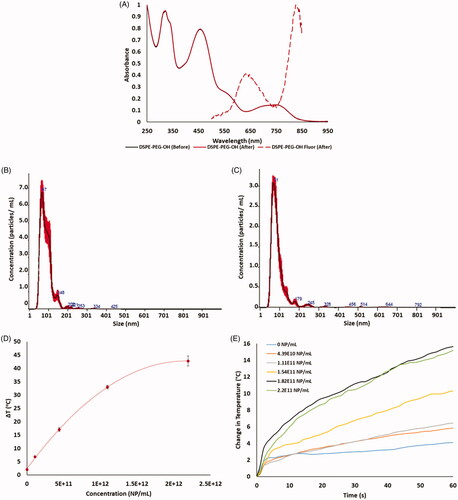
Table 1. HDAPPs concentration and the corresponding average and maximum temperature rise over baseline during stimulation with 60 s of 800 nm (3 w/cm2) continuous-wave light.
Following mild hyperthermia induced by HDAPPs at varying concentrations, the loss in luciferase activity was determined as a function of nanoparticle concentration (). Luciferase activity was then correlated to intracellular CEM43 and is shown related to the CEM43 calculated from bulk nanoparticle solution temperatures measured by a thermocouple (). The temperature values provided above the nanoparticle concentrations in were obtained from measurements of bulk solutions of HDAPPs in which the adherent cells were maintained during the photothermal experiments (800 nm CW light for 60 s). Relative fluorescent intensity units (RLU) were then used to determine CEM43 values, as shown in . The luminescent measurements map closely to their calculated CEM43 values, obtained by thermocouple measurement, illustrating the relative accuracy of the dosimetry technique in both parental and OxR cell lines. As seen in , there are substantial differences when the average temperature of the bulk HDAPPs solution over the entirety of the laser exposure (60 s) is used compared to using the cumulative sum of CEM43 values obtained over 1, 5 or 10 s intervals. Utilizing an EM-CCD capture device, the luminescent signal from individual cells was recorded and intensity decay as a function of the thermal dose is displayed following HDAPPs-mediated hyperthermia at multiple concentrations within both parental and OxR CT26 variants (). Here, spatial resolutions with pixel sizes of 1.8 µm x 1.8 µm were achieved. The median cell-luminescence intensity and respective interquartile ranges are presented for each treatment in , using a fixed region of interest to measure the luminescence of 90 cells in each treatment population. Individual cell luminescence intensity indicates modest variation in luminescence intensity within each nanoparticle concentration used, and intensity values within each concentration are statistically distinguishable in both the parental and OxR CT26 variants. Visualization of the HDAPPs induced thermal dose provides qualitative proof to support the RLU measurements and corresponding thermal dose in .
Figure 3. (A) Normalized percent loss of luminescence intensity as a function of nanoparticle concentration and CT26 cell variant under 60 s irradiation with 3 W, 800 nm continuous wave laser (1 cm2 spot size). Temperatures provided are interpolated values from experimental data collected in . (B) Correlated CEM43 as a function of nanoparticle concentration and CT26 cell variant. Solid gray bars indicate CEM43, as measured with a thermocouple in bulk solution, using the average temperature over 60 s. Gray bars with lines are CEM43 calculations using the sum of CEM43 values attained using 1 s (horizontal stripes), 5 s (diagonal stripes) or 10 s (vertical stripes) intervals. (C) Visual confirmation of loss of luminescence under respective nanoparticle concentrations in both parental and OxR CT26 cell lines. EM-CCD imaging allows resolution down to a single cell. (D) RLU decreases calculated by measuring 90 individual cells per group, as shown in part C.
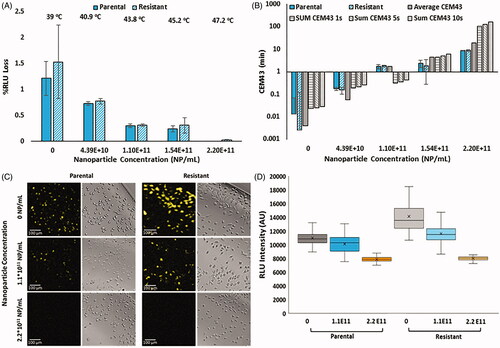
Measurement of cell viability following HDAPPs photothermal therapy results in no significant loss of viability for parental or OxR cells when viability is measured immediately after laser irradiation (), but measurement 24 h after treatment shows significant reductions in viability, as demonstrated in . For parental and OxR cells evaluated 24 h after treatment, no significant difference in viability was detected between any of the HDAPPs concentrations treated without laser. Instead, for both parental and OxR cells, when laser is included a significant difference in viability is detected when the highest concentrations are directly compared to the lower ones. When holding concentration constant and then comparing the effect of laser stimulation, there is a significant difference between no laser and laser groups at 2.2 × 1011 NP/mL for parental cells. OxR cells treated with 2.20 × 1011 NP/mL did not achieve a statistically significant decrease in viability, although they did show a difference at a concentration of 1.54 × 1011 NP/mL. While there is no significant difference between the no laser vs. laser treatments within the 2.2 × 1011 NP/mL concentration, a significant difference was determined when comparing the 2.2 × 1011 NP/mL concentration to the 0 NP/mL concentration within the laser treatment.
Figure 4. Cell viability of parental and OxR cells measured immediately after HDAPPs- induced photothermal therapy (A) and 24 h after treatment (B). ‘§’ indicates statistical significance between the identified group and the no nanoparticle group. § indicates statistical significance between the laser and non-laser treated groups within the same cell line and nanoparticle concentration. NL indicates no laser and L indicates the application of the laser stimulation.
*Signifies that there are statistical differences between oxaliplatin resistant cells treated with laser (L) compared to without (NL), or in the case of the highest nanoparticle concentration, that there are differences in the oxaliplatin sensitive cells treated with laser (L) versus without (NL).
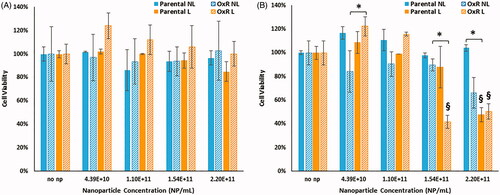
Response to HDAPPs-induced hyperthermia plus oxaliplatin
We next determined whether HDAPP-based photothermal treatment sensitized oxaliplatin sensitive and resistant CRC cells to drug treatment. Luminescence measured immediately after HDAPPs- induced hyperthermia with oxaliplatin indicates 2.12- to 4.64-fold reductions for all groups treated with laser and nanoparticles, relative to those receiving the same oxaliplatin concentration and nanoparticle combination without laser, as shown in . Luminescence measured 24 h after photothermal treatment () demonstrates decreased luminescence in all groups receiving oxaliplatin compared to no oxaliplatin. There was a profound decrease in the luminescence of almost all the groups treated with HDAPPs-induced photothermal therapy plus oxaliplatin, which may be indicative of the nanoparticle-based hyperthermia applying further stress to the cells leading to reduced cell numbers and luminescence. Although the decrease in luminescence measured 24 h after photothermal treatment cannot be related to the intracellular temperature that the cells experience prior, the results do appear to correlate with a reduction in cell viability, as shown in . For both the parental and OxR populations, the addition of HDAPPs induced photothermal therapy leads to reduced cell viability relative to groups treated with equivalent oxaliplatin concentrations without HDAPPs (with the exception of 50 µM oxaliplatin with HDAPPs photothermal therapy for OxR cells). With no oxaliplatin, photothermal therapy alone reduces parental cell viability by 2.17-fold and OxR viability by 2.18-fold. The addition of 50 µM oxaliplatin to parental cells treated with HDAPPs- induced hyperthermia resulted in a 4-fold decrease compared to parental cells treated with HDAPPs but no laser stimulation or oxaliplatin. There are notable additional reductions in cell viability when HDAPPs are combined with oxaliplatin, even in the absence of infrared stimulation to generate heat (comparing the no laser (NL) groups). At 50 µM of oxaliplatin, there is no difference in OxR cells treated with and without laser in the nanoparticle (NP) and no NP groups. Parental cells treated with 100 µM of oxaliplatin and no laser had a 1.6-fold decrease in cell viability without HDAPPs and a 2.26-fold decrease with HDAPPs and no laser. A similar reduction was observed for OxR cells treated without laser and 100 µM of oxaliplatin, which had a 1.32 reduction in viability, compared to a 1.61-fold reduction with the addition of HDAPPs. Similar trends were also noted in the 300 µM treated groups of parental (2.56- to 3.7-fold reduction, with HDAPPs) and OxR CT26 cells (2- to 2.2-fold (with HDAPPs) reduction). As our team has shown previously, and as demonstrated in the HDAPPs only group with no infrared stimulation or oxaliplatin, HDAPPs are not toxic. Infrared stimulation with HDAPPs and no oxaliplatin significantly reduces both parental and OxR cells, resulting in a 2-fold reduction in viability. Parental cells treated with 100 µM oxaliplatin and laser had a 2.7-fold reduction in cell viability and the addition of HDAPPs induced heating led to a 13.5-fold reduction. Further reduction was not noted for either parental cells treated with 300 µM, HDAPPs and laser stimulation. OxR cells had a 1.7-fold reduction with laser stimulation and no HDAPPs and 100 µM oxaliplatin. The addition of HDAPPs for heat generation with laser exposure and 100 µM oxaliplatin led to a 5.6-fold reduction. This result was not significantly improved with increased oxaliplatin concentration. There were also differences in the parental and OxR cell populations with 300 µM oxaliplatin, no laser, no HDAPPs, where the parental line has a 3.65-fold reduction compared to only a 2.17-fold reduction for OxR cells. Combination with HDAPPs induced photothermal therapy resulted in both populations having a reduction of about 12.5-fold. For all of the HDAPPs treated groups, the concentration used was 2.2 × 1011 NP/mL, which under the conditions of the current experiment yielded a CEM 43 of 18.77.
Figure 5. Parental and OxR cells were treated with increasing concentrations of oxaliplatin and with or without 2.2 × 1011 NP/mL, with or without laser exposure for 60 s. (A) Luminescence measured immediately after photothermal treatment and (B) measured 24 h after. (C) Cell viability measured 24 h after photothermal treatment. ‘§’ indicates a statistical significance between cells treated with laser stimulation or not. ‡ indicates statistical significance between the indicated group and their respective parental or OxR cells that did not receive HDAPPs or oxaliplatin. NL indicates no laser and L indicates the application of the laser stimulation.
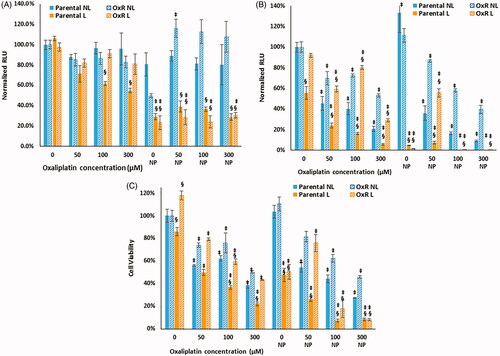
Discussion
In the present work, oxaliplatin-resistant cells were developed for the evaluation of hyperthermic chemotherapy treatment in CRC. Noting the pleiotropic effects of hyperthermia compared to other single-target molecular therapies that are largely dependent on tumor genotype, hyperthermic chemotherapy may be a superior modality for the augmentative treatment of pcCRC, even pcCRC refractory to chemotherapy [Citation47]. Our findings further link to clinical studies that prescribe the use of cytoreductive surgery + HIPEC in recurrent CRC, in particular platinum-resistant relapse associated with peritoneal dissemination [Citation13,Citation16,Citation17,Citation48–51]. Our team has previously shown that HDAPPs targeted to peritoneally disseminated CRC can delivered by intraperitoneal perfusion in a murine model of pcCRC [Citation52]. The HDAPPs attach to small CRC tumors and can generate heat, with the goal being the generation of mild hyperthermia to facilitate chemotherapy (Nano-HIPEC). HDAPPs are fluorescent and may be detectable following surgical debulking to identify regions where laser exposure is needed to generate heat. The laser would need to be applied to local regions as detected by where the HDAPPs have bound to micrometastatic lesions. The aim of the current work was to demonstrate that reductions in luminescence may be correlated with photothermal nanoparticle-induced thermal dose. Sufficiently high thermal doses will not be needed for Nano-HIPEC as the goal is to use mild hyperthermia around 42 °C. The pre-clinical animal model can also incorporate a light scattering agent (Nutrilipid®) to aid in the dispersion of the laser stimulation light, which compliments manipulation of the peritoneal tissues.
Current measurement techniques for thermal dose are often limited by specialized equipment and physical impediments that lead to restricted resolution such as in MRI-dosimetry [Citation53]. Other techniques that can achieve single-cell resolution such as fluorescent-correlated thermography often require the utilization of foreign probes that can alter cell behavior in addition to measurement imprecisions from environmental effects [Citation54,Citation55]. These variations represent the need for a standard by which thermal dose can be referenced in mild hyperthermic treatment of cancer. Particularly in cases where nanoparticle-mediated thermal dose may be severely underestimated due to misleading bulk vs. nanoscale temperatures, a native, nontoxic metric localized within the cell is important [Citation21,Citation56]. In the present work, a previously established luciferase-based model was validated within both parental and OxR CT26 CRC cells [Citation33]. Intracellular luminescence measurements serve as a precise means of measuring cellular thermal dose in the case of nanoparticle-based heating, where single-cell thermal dosing is captured. It has been reported that the effective thermal dose generated by photothermal nanoparticles is often severely underestimated by calculations of thermal dose using bulk fluid temperature, suggesting the need of a dosimetric technique that can account for this traditionally immeasurable component of nanoparticle-generated hyperthermia [Citation25,Citation26]. To determine the predictive value of the CEM43 luciferase model in photothermal nanoparticle hyperthermia, HDAPPs were utilized to generate heat with CT26 parental and OxR cell lines. Comparison of calculated CEM43 using the average temperature over the 1 min heating period correlates well with the luciferase determination of CEM 43 measurements. Since the temperature of the solution around the CRC cells is continually increasing during the photothermal treatment the sum of CEM43 calculations at specific time/temperature intervals are most appropriate to use, although they do not fit the luciferase model as well.
While our current study is limited to 2-dimensional culture, it is easily envisioned for these measurements to be performed with previously established HIPEC in vivo models [Citation43,Citation57]. We have previously shown that HDAPPs bind to luminescent CT26 cells in murine models of pcCRC [Citation52]. HDAPPs were not taken up by the cells for this work; rather, a suspension of the nanoparticles bathed the cells, which is analogous to what might be done for binding studies or perfusion similar to HIPEC. The current work has demonstrated that reductions in luminescence in response to HDAPPs-induced photothermal heating can be quantified using a luminometer, and also by microscopy. Bioluminescence emission for luciferase can vary between 480 to 620 nm, and for the current CT26 cells used in this work, the peak is 560 nm. For preclinical models (specifically Balb/c mice with luciferase-expressing CT26 tumors) we have detected luminescence in the retroperitoneal space imaging from the abdomen, or through about 1.5 cm of tissue. A thermal imaging camera might be better in some applications, but our goal is ultimately to target the fluorescent HDAPPs to small tumor nodules within the peritoneal cavity of a mouse. The future extension of this work is to evaluate reductions in luminescence that correlate with thermal dose in a murine model of pcCRC using an in vivo imaging system (IVIS), which is routinely used to measure tumor burden in preclinical models. Similar to the methodology we used in the current work to measure luminescence of cells in a specific region of interest in order to quantitatively predict thermal dose, we propose that a similar technique should be possible using IVIS. The ultimate goal of using heat-generating nanoparticles for treating pcCRC is to locally optimize the thermal dose experienced by micron-sized tumors that may not be resectable, in the presence of chemotherapy, to maximize reductions in tumor burden and minimize the collateral damage in adjacent tissues that can impede healing following HIPEC.
Additionally, we have demonstrated that the utilization of this luciferase-based dosimetry with luminescence microscopy allows for the resolution of thermal damage at the single-cell, and feasibly sub-cellular level, where such precision in thermal measurement is largely inaccessible by most other modalities of temperature measurement. Localization of luciferase through organelle-specific expression vectors, such as nuclear luciferase, represents an already available technology that can advance the spatial precision of this metric [Citation58]. Where some heterogeneity of cell signal is present within the current iteration of EM-CCD imaging for cellular luminescence, enhancement in focal plane alignment and reduction of EM-CCD capture noise should increase cell luminescence intensity homogeneity, suggesting the potential for this technique given further optimization. Perhaps the greatest benefit of this method is that it relies on simple and intuitive imaging equipment, where no in-line temperature imaging is required to measure thermal dose while a treatment is being applied. Our results demonstrate that non-contact rapid hyperthermia induced by photothermal nanoparticles can be monitored using this technique for thermal measurements in real-time. Thus, we envision the OxR-CT26 luciferase model to be critical in evaluating heat-generating nanoparticles for their capacity to serve as both a standalone, targeted mild hyperthermia therapeutic as well as a means to augment oxaliplatin against chemoresistant CRC cells based on prior evidence of the advantage of nanoparticle-mediated hyperthermia over classical modes of thermal delivery [Citation21,Citation22]. Additional coupling of these cell lines with therapeutic agents also carrying diagnostic capacity, such as the theranostic potential of fluorescent HDAPPs, will serve as a means to confirm targeting mechanisms within OxR populations as well as directly relate thermal dose to a calculable dose of nanoparticles bound to cells.
Pre-clinical models of pcCRC using Nano-HIPEC where OxR cells are utilized can begin to aid the medical community in understanding how precision heating may sensitize chemotherapy-resistant cells, which may alleviate one of the challenges of recurrent disease. HDAPPs induced hyperthermia led to a dramatic reduction in both the parental and OxR CRC cells and combination with the clinically relevant concentration (300 µM) of oxaliplatin resulting in a 5.75-fold additional reduction in cell viability compared to the 3.43-fold reduction for the parental cell line. Although a short term assay of viability was used and further analysis needs to be performed using clonogenics, the trend indicates that OxR cells may become re-sensitized to oxaliplatin upon introduction to photothermal hyperthermia. It is envisioned that photothermal nanoparticles could provide localized hyperthermia to tumors that are too small to be surgically removed by binding to the tumor cells selectively (for example, by targeting CXCR4 receptors) to identify them using the inherent fluorescence of HDAPPs, with subsequent application of infrared light locally following open abdominal debulking surgeries.
Conclusion
We present here models of OxR-CRC to serve as a quantitative, pre-clinical platform for the advancement of next-generation thermal therapies, particularly using photothermal nanoparticles. The utility of hyperthermia to augment oxaliplatin using photothermal nanoparticles has been demonstrated and correlated with a cellular thermal dose for the first time. Moreover, the development of a new high-resolution luciferase-based thermal dosimetry technique permits the investigation and translation of targeted modalities of hyperthermia, such as photothermal nanoparticles, to better realize the potential of hyperthermia within the pre-clinical practice.
Acknowledgments
Thanks to Shay Soker for allowing the use of the EM-CCD capture device.
Disclosure statement
No potential conflict of interest was reported by the author(s).
Additional information
Funding
References
- Holch J, Stintzing S, Heinemann V. Treatment of metastatic colorectal cancer: standard of care and future perspectives. Visc Med. 2016;32(3):178–183.
- Howlader NNA., Krapcho M, Miller D, et al. (eds.). SEER cancer statistics review. Bethesda (MD): National Cancer Institute; 2020.
- Goldberg RM, Rothenberg ML, Van Cutsem E, et al. The continuum of care: a paradigm for the management of metastatic colorectal cancer. Oncologist. 2007;12(1):38–50.
- Sugarbaker P. Management of peritoneal-surface malignancy: the surgeon’s role. Langenbecks Arch Surg. 1999;384(6):576–587.
- Glehen O, Cotte E, Kusamura S, et al. Hyperthermic intraperitoneal chemotherapy: nomenclature and modalities of perfusion. J Surg Oncol. 2008;98(4):242–246.
- Hildebrandt B, Wust P, Ahlers O, et al. The cellular and molecular basis of hyperthermia. Crit Rev Oncol/Hematol. 2002;43(1):33–56.
- Issels RD. Hyperthermia adds to chemotherapy. Eur J Cancer. 2008;44(17):2546–2554.
- Levine EA, Stewart IV, Shen JH, et al. Intraperitoneal chemotherapy for peritoneal surface malignancy: experience with 1,000 patients. J Am Coll Surg. 2014;218(4):573–585.
- Graham J, Mushin M, Kirkpatrick P. Oxaliplatin. Nat Rev Drug Discov. 2004;3(1):11–12.
- Zhang X-L, Hu A-B, Cui S-Z, et al. Thermotherapy enhances oxaliplatin-induced cytotoxicity in human colon carcinoma cells. World J Gastroenterol. 2012;18(7):646.
- Quenet F, Elias D, Roca L, et al. A UNICANCER phase III trial of hyperthermic intra-peritoneal chemotherapy (HIPEC) for colorectal peritoneal carcinomatosis (PC): PRODIGE 7. J Clin Oncol. 2018;36(18)
- Ceelen W. HIPEC with oxaliplatin for colorectal peritoneal metastasis: the end of the road? Eur J Surg Oncol. 2019;45(3):400–402.
- Van Driel WJ, Koole SN, Sikorska K, et al. Hyperthermic intraperitoneal chemotherapy in ovarian cancer. N Engl J Med. 2018;378(3):230–240.
- Brouquet A, Goéré D, Lefèvre JH, et al. The second procedure combining complete cytoreductive surgery and intraperitoneal chemotherapy for isolated peritoneal recurrence: postoperative course and long-term outcome. Ann Surg Oncol. 2009;16(10):2744–2751.
- Martinez-Balibrea E, Martínez-Cardús A, Ginés A, et al. Tumor-related molecular mechanisms of oxaliplatin resistance. Mol Cancer Ther. 2015;14(8):1767–1776.
- Alzahrani NA, Valle SJ, Fisher OM, et al. Iterative cytoreductive surgery with or without hyperthermic intraperitoneal chemotherapy for colorectal peritoneal metastases: a multi‐institutional experience. J Surg Oncol. 2018;119(3):336–346.
- Mogal H, Chouliaras K, Levine EA, et al. Repeat cytoreductive surgery with hyperthermic intraperitoneal chemotherapy: review of indications and outcomes. J Gastrointest Oncol. 2016;7(1):129–142.
- Graham EG, Macneill CM, Levi-Polyachenko NH. Review of metal, carbon and polymer nanoparticles for infrared photothermal therapy. Nano Life. 2013;03(03):1330002.
- Levi-Polyachenko NH, Stewart I, John H. Clinical relevance of nanoparticle induced hyperthermia for drug delivery and treatment of abdominal cancers. The Open Nanomed Nanotechnol J. 2011;3(1):24–37.
- Kobayashi T. Cancer hyperthermia using magnetic nanoparticles. Biotechnol J. 2011;6(11):1342–1347.
- Burke AR, Singh RN, Carroll DL, et al. The resistance of breast cancer stem cells to conventional hyperthermia and their sensitivity to nanoparticle-mediated photothermal therapy. Biomaterials. 2012;33(10):2961–2970.
- Levi-Polyachenko NH, Merkel EJ, Jones BT, et al. Rapid photothermal intracellular drug delivery using multiwalled carbon nanotubes. Mol Pharm. 2009;6(4):1092–1099.
- Graham-Gurysh E, Kelkar S, McCabe-Lankford E, et al. Hybrid donor-acceptor polymer particles with amplified energy transfer for detection and on-demand treatment of breast cancer. ACS Appl Mater Interfaces. 2018;10(9):7697–7703.
- Tian Z, Yu J, Wu C, et al. Amplified energy transfer in conjugated polymer nanoparticle tags and sensors. Nanoscale. 2010;2(10):1999–2011.
- Engelmann UM, Roeth AA, Eberbeck D, et al. Combining bulk temperature and nanoheating enables advanced magnetic fluid hyperthermia efficacy on pancreatic tumor cells. Sci Rep. 2018;8(1):13210.
- Qin Z, Etheridge M, Bischof JC. Nanoparticle heating: nanoscale to bulk effects of electromagnetically heated iron oxide and gold for biomedical applications. Paper presented at the SPIE BiOS conference; 2011 January 22–27; San Fransisco, CA.
- Bischof JC. Micro and nanoscale phenomenon in bioheat transfer. Heat Mass Transfer. 2006;42(10):955–966.
- Sapareto SA, Dewey WC. Thermal dose determination in cancer therapy. Int J Radiati Oncol Biol Phys. 1984;10(6):787–800.
- Dewhirst MW, Viglianti B, Lora-Michiels M, et al. Basic principles of thermal dosimetry and thermal thresholds for tissue damage from hyperthermia. Int J Hyperthermia. 2003;19(3):267–294.
- Hoopes PJ, Petryk AA, Giustini AJ, et al. Nanoparticle based cancer treatment: can delivered dose and biological dose be reliably modeled and quantified? Proc SPIE–the Int Soc Optic Eng. February 23rd, 2011: 79010A.
- Tarapacki C, Karshafian R. Enhancing laser therapy using PEGylated gold nanoparticles combined with ultrasound and microbubbles. Ultrasonics. 2015;57:36–43.
- Pearce JA. Comparative analysis of mathematical models of cell death and thermal damage processes. Int J Hyperthermia. 2013;29(4):262–280.
- Raoof M, Zhu C, Kaluarachchi WD, et al. Luciferase-based protein denaturation assay for quantification of radiofrequency field-induced targeted hyperthermia: developing an intracellular thermometer. Int J Hyperthermia. 2012;28(3):202–209.
- Ubink I, Bolhaqueiro A, Elias S, et al. Organoids from colorectal peritoneal metastases as a platform for improving hyperthermic intraperitoneal chemotherapy. Br J Surg. 2019;106(10):1404–1414.
- Jensen NF, Stenvang J, Beck MK, et al. Establishment and characterization of models of chemotherapy resistance in colorectal cancer: towards a predictive signature of chemoresistance. Mol Oncol. 2015;9(6):1169–1185.
- Yan X-D, Li M, Yuan Y, et al. Biological comparison of ovarian cancer resistant cell lines to cisplatin and Taxol by two different administrations. Oncol Rep. 2007;17(5):1163–1169.
- Barr MP, Gray SG, Hoffmann AC, et al. Generation and characterisation of cisplatin-resistant non-small cell lung cancer cell lines displaying a stem-like signature. PLoS One. 2013;8(1):e54193.
- Mukherjee A, Castanares M, Hedayati M, et al. Monitoring nanoparticle-mediated cellular hyperthermia with a high-sensitivity biosensor. Nanomedicine. 2014;9(18):2729–2743.
- Roper DK, Ahn W, Hoepfner M. Microscale Heat Transfer Transduced by Surface Plasmon Resonant Gold Nanoparticles. J Phys Chem C. 2007;111(9):3636–3641.
- Andrews PA, Murphy MP, Howell SB. Characterization of cisplatin-resistant COLO 316 human ovarian carcinoma cells. Eur J Cancer Clin Oncol. 1989;25(4):619–625.
- Boyer J, McLean EG, Aroori S, et al. Characterization of p53 wild-type and null isogenic colorectal cancer cell lines resistant to 5-fluorouracil, oxaliplatin, and irinotecan. Clin Cancer Res. 2004;10(6):2158–2167.
- McCabe‐Lankford EE, Brown TL, Levi‐Polyachenko NH. Assessing fluorescence detection and effective photothermal therapy of near-infrared polymer nanoparticles using alginate tissue phantoms. Lasers Surg Med. 2018;50(10):1040–1049.
- McCabe-Lankford E, Peterson M, McCarthy B, et al. Murine models of intraperitoneal perfusion for disseminated colorectal cancer. J Surg Res. 2019;233:310–322.
- Sarkar S, Levi-Polyachenko N. Conjugated polymer nano-systems for hyperthermia, imaging and drug delivery. Adv Drug Deliv Rev . 2020;163–164:40–64.
- Chen H, Shao L, Ming T, et al. Understanding the photothermal conversion efficiency of gold nanocrystals. Small. 2010;6(20):2272–2280.
- Cole JR, Mirin NA, Knight MW, et al. Photothermal efficiencies of nanoshells and nanorods for clinical therapeutic applications. J Phys Chem C. 2009;113(28):12090–12094.
- Hettinga J, Konings A, Kampinga H. Reduction of cellular cisplatin resistance by hyperthermia-a review. Int J Hyperthermia. 1997;13(5):439–457.
- Spiliotis J, Halkia E, Lianos E, et al. Cytoreductive surgery and HIPEC in recurrent epithelial ovarian cancer: a prospective randomized phase III study. Ann Surg Oncol. 2015;22(5):1570–1575.
- Deraco M, Virzi S, Iusco D, et al. Secondary cytoreductive surgery and hyperthermic intraperitoneal chemotherapy for recurrent epithelial ovarian cancer: a multi-institutional study. BJOG. 2012;119(7):800–809.
- Bakrin N, Cotte E, Golfier F, et al. Cytoreductive surgery and hyperthermic intraperitoneal chemotherapy (HIPEC) for persistent and recurrent advanced ovarian carcinoma: a multicenter, prospective study of 246 patients. Ann Surg Oncol. 2012;19(13):4052–4058.
- Bakrin N, Bereder J-M, Decullier E, et al. Peritoneal carcinomatosis treated with cytoreductive surgery and Hyperthermic Intraperitoneal Chemotherapy (HIPEC) for advanced ovarian carcinoma: a French multicentre retrospective cohort study of 566 patients. Eur J Surg Oncol. 2013;39(12):1435–1443.
- McCabe-Lankford E, McCarthy B, Berwick MA, et al. Binding of targeted semiconducting photothermal polymer nanoparticles for intraperitoneal detection and treatment of colorectal cancer. Nanotheranostics. 2020;4(3):107–118.
- Odéen H, Parker DL. Non‐invasive thermometry with magnetic resonance imaging. Theory Appl Heat Transf Humans. 2018;1:267–299.
- Wang X-d, Wolfbeis OS, Meier RJ. Luminescent probes and sensors for temperature. Chem Soc Rev. 2013;42(19):7834–7869.
- Baffou G, Rigneault H, Marguet D, et al. A critique of methods for temperature imaging in single cells. Nat Methods. 2014;11(9):899–901.
- Creixell M, Bohorquez AC, Torres-Lugo M, et al. EGFR-targeted magnetic nanoparticle heaters kill cancer cells without a perceptible temperature rise. ACS Nano. 2011;5(9):7124–7129.
- Gremonprez F, Willaert W, Ceelen W. Intraperitoneal chemotherapy (IPC) for peritoneal carcinomatosis: review of animal models. J Surg Oncol. 2014;109(2):110–116.
- Michels AA, Nguyen VT, Konings AW, et al. Thermostability of a nuclear-targeted luciferase expressed in mammalian cells. Destabilizing influence of the intranuclear microenvironment. Eur J Biochem. 1995;234(2):382–389.