Abstract
Purpose
To evaluate the targetability of late-stage cervical cancer by magnetic resonance-guided high-intensity focused ultrasound (MRgHIFU)-induced hyperthermia (HT) as an adjuvant to radiation therapy (RT).
Methods
Seventy-nine cervical cancer patients (stage IIIB–IVA) who received RT with lesions visible on positron emission tomography-computed tomography (PET-CT) were retrospectively analyzed for targetability using a commercially-available HT-capable MRgHIFU system. Targetability was assessed for both primary targets and/or any metastatic lymph nodes using both posterior (supine) and anterior (prone) patient setups relative to the transducer. Thirty-four different angles of rotation along subjects’ longitudinal axis were analyzed. Targetability was categorized as: (1) Targetable with/without minimal intervention; (2) Not targetable. To determine if any factors could be used for prospective screening of patients, potential associations between demographic/anatomical factors and targetability were analyzed.
Results
72.15% primary tumors and 33.96% metastatic lymph nodes were targetable from at least one angle. 49.37% and 39.24% of primary tumors could be targeted with patient laying in supine and prone positions, respectively. 25°–30° rotation and 0° rotation had the highest rate of the posterior and anterior targetability, respectively. The ventral depth of the tumor and its distance to the coccyx were statistically correlated with the anterior and posterior targetability, respectively.
Conclusion
Most late-stage cervical cancer primaries were targetable by MRgHIFU HT requiring either no/minimal intervention. A rotation of 0° or 25°–30° relative to the transducer might benefit anterior and posterior targetability, respectively. Certain demographic/anatomic parameters might be useful in screening patients for treatability.
Introduction
Cervical cancer is the fourth most prevalent cancer of women worldwide after breast, lung, and colorectal cancer [Citation1,Citation2]. Patients with locally advanced cervical cancer (FIGO stage IB2–IVA [Citation3]) have worse survival and higher rates of recurrence compared to early stage (IA–IB1) [Citation4]. The 5-year survival is 89.1%, 41.5%, and 22.0% for stages IB1, IIIB and IVA, respectively [Citation4]. While the first-line treatment is typically chemotherapy in combination with radiation therapy (RT) to the primary cancer and metastatic lymph nodes [Citation5], a study of 238 cervical cancer patients receiving chemo-RT found that 38% experienced a recurrence within three months, as detected by positron emission tomography (PET) [Citation6]. Additionally, chemo-RT is associated with a negative impact on the quality-of-life [Citation7–10]. For example, one clinical study reported bowel dysfunction (49%), sexual dysfunction (55%), and sense of femininity loss and/or divorce (42%) in cervical cancer patients (N = 98) receiving chemo-RT [Citation10]. Innovative treatment modalities could potentially improve the response rate, survival, and/or quality-of-life.
Hyperthermia (HT), which refers to increasing the temperature to 40–45 °C for an extended duration, is a clinically proven sensitizer for RT [Citation11,Citation12] and chemotherapy [Citation13]. The addition of HT to RT substantially improves local control and overall survival without affecting treatment-related grade 3–4 toxicity, according to a meta-analysis [Citation14] from the Cochrane Database from six phase III/IV clinical trials [Citation15–21], although target temperatures in HT studies varied over a range of 41.0 °C–43.0 °C [Citation22–28] and HT duration varied over a range of 15 min [Citation26]–80 min [Citation29,Citation30]. In one example from this meta-analysis, a randomized multicenter trial reported an increased complete response rate of 26% without enhanced toxicity when using HT + RT compared to RT alone (p = 0.003) [Citation21]. The most widely used approach for validating the adjuvant effect of HT + RT is to keep RT dose consistent and observe a significantly higher complete response rate, local control [Citation11,Citation16,Citation31–34], and/or a longer overall survival [Citation16,Citation32] when combining RT with HT compared to RT alone. However, a past clinical trial [Citation35] did investigate the use of HT with a reduction of RT dose with the goal of achieving a similar efficacy, and the results suggested that the addition of HT could help reduce about 33% of the XRT dose compared to XRT alone. For the timing of RT and HT, simultaneous application or a relatively short (<4 h) time interval between the RT and HT, was reported to be able to maximize the radiosensitization effect of HT [Citation36].
Clinically, HT can be delivered by electromagnetic wave-based techniques or ultrasound. Due to the large wavelengths and/or high energy absorption rate in tissue, electromagnetic heating is hard to be localized when targeting deep sites, hence it is mostly limited to superficial disease or regional heating for deep targets [Citation37]. Ultrasound can provide increased localization to depths ranging from less than 1 cm to 10 cm or deeper by adjusting the frequency and/or utilizing focused beams [Citation38,Citation39]. Additionally, in most clinical studies, thermocouples were used for temperature monitoring, which is invasive and only provides temperatures at the location of the monitor probe(s) [Citation16,Citation18–21].
Magnetic resonance-guided high intensity focused ultrasound (MRgHIFU) has emerged as a novel and alternative modality for inducing HT [Citation40,Citation41]. As a noninvasive technique providing dynamic three-dimensional temperature measurement and real-time temperature feedback control, MRgHIFU is able to create localized and volumetric HT for an extended duration with satisfactory accuracy and precision [Citation40–42]. MRgHIFU-induced HT has been shown to be feasible and safe in living animals [Citation40–42] and is being evaluated in ongoing clinical trials for rectal cancer [Citation43] and pediatric sarcoma [Citation44]. However, the accessibility of targets by MRgHIFU depends on a clear ultrasound beam-path without bone and/or air-containing structures due to the potential for acoustic mismatches and/or energy absorption-related heating injury of adjacent normal tissues (at the interfaces of air/bone and tissue). Therefore, a careful examination of the target anatomy is required to assess the safe and effective targetability by HT.
While there have been some limited studies that analyzed the targetability of MRgHIFU-induced thermal ablation [Citation45–49] and/or HT [Citation48,Citation50]. None of these studies investigated, factors that could potentially influence targetability (i.e., demographics, anatomical factors, or patient setups). In terms of analyzing targetability for thermal ablation, previous studies have been performed on a number of sites, including pediatric sarcoma [Citation48], osteoid osteoma, bone metastases [Citation45], recurrent gynecological tumors [Citation46], and abdominal neuroblastoma [Citation47]. The data is even more limited for cervical cancer with only two case reports [Citation51,Citation52] and a virtual planning study (n = 6 in recurrent lesions) [Citation46] demonstrating feasible targeting by thermal ablation. For HT, targetability has only been assessed in pediatric sarcoma [Citation48] and osteomyelitis [Citation50] and no analysis of the targetability of MRgHIFU-induced HT in primary cervical cancer or metastatic lymph node(s) has yet been performed.
Thus, the objectives of this study were to: (1) evaluate the percentage of primary lesions and metastatic lymph node(s) in late-stage cervical cancer (stage IIIB–IVA) patients that can be targeted by a MRgHIFU device; (2) assess optimal patient positioning for the highest targetability rate; and (3) analyze any statistical correlation between the patients’ anatomical geometries/demographics and targetability. To achieve these aims, we retrospectively reviewed treatment imaging from stage IIIB-IVA cervical cancer patients receiving RT [external beam radiation therapy (EBRT) or high dose rate brachytherapy (HDR brachytherapy)] at our institution over a 9-year period. We determined the targetability of each patient using commercial MRgHIFU planning software with ultrasound beam-path targeting from both anteriorly (patients in prone position) and posteriorly (patients in supine position) at different angles relative to the transducer. An array of anatomic and demographic parameters for each patient was tabulated and their association with targetability was evaluated.
Methods
Ethical approval
The study protocol was approved by the Institutional Review Board at the Washington University School of Medicine. All patient-identifying information was kept confidential. Patient anonymity was preserved, and the principles of the Declaration of Helsinki were followed. All procedures followed the guidelines set forth by the Health Insurance Portability and Accountability Act Privacy rule.
Patient selection
This study selected patients with a pathological diagnosis of late stage cervical cancer (stage IIIB–IVA) who received EBRT, HDR brachytherapy, or the combination of these two modalities at the Siteman Cancer Center at Barnes Jewish Hospital in Saint Louis from 1 January 2010 to 1 April 2019. From a database 118 consecutive patients, 39 were excluded due to a lack of PET-CT images and/or recognizable tumor lesions. The remaining 79 patients were analyzed. Demographic parameters including patients’ age, weight, height, BMI, tumor stage, and RT modalities/dosages were recorded.
Image examination and processing
For each patient, co-registered PET-CT images in the Eclipse treatment planning system (Varian Medical Systems, Palo Alto, CA) were reviewed. In the FDG-PET images, ‘metabolic tumor volumes’ from both the primary tumor lesion (MTV) and any metastatic lymph node(s) (MTV-LN, if present) were contoured by selecting the regions equal to or greater than a standardized uptake value (SUV) of 40% of the peak tumor intensity (the standard value for quantifying tumor volume on PET-CT) [Citation53,Citation54] as shown in . The MTV/MTV-LN represents the metabolically active tumor region(s), and is an independent prognostic factor for disease-free survival in cervical cancer patients [Citation55]. They were also used as the target tumor lesions in this study. All delineated MTVs and MTV-LNs were drawn and approved by experienced radiation oncologists and utilized for the treatment planning of RT.
Figure 1. The schematic illustration of pelvic bony landmarks on a representative PET-CT imaging and the anatomical measurements. Figure caption: (A) Axial view that presents the largest cross-section of MTV, MHF, MRL, DMA, DMP, AF, PF, and DAP. (B) Axial view that presents RIS, LIS, coccyx, LISC, RISC, and DIS. (C) Sagittal view that presents the coccyx, PS, MTV, bladder, MC, MPS, and MAP. (D) 3-dimensional reconstruction of bony structures of CT images that illustrates the LIS, RIS, coccyx, PS, DIS, RISC, and LISC. (E) Coronal view demonstrates MTV and MTV-LN. (F) Axial view illustrates the MTV-LN, D-LN, and DB-LN.
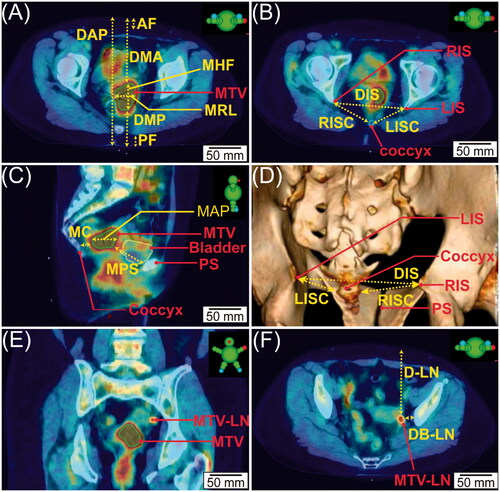
On each PET-CT image series, four bony pelvic landmarks were identified and marked: the left ischial spine (LIS), the right ischial spine (RIS), the pubic symphysis (PS), and the coccyx, as shown in . Next, a number of anatomical measurements were tabulated (): the largest dimension of MTV in the head-foot axis (MHF), the largest dimension of the MTV in the right-left axis (MRL), the largest dimension of MTV in the anterior-posterior axis (MAP), the anterior (ventral) depth of the MTV (DMA), the posterior (dorsal) depth of the MTV posteriorly (DMP), the thickness of the anterior subcutaneous fat layer at the level of the MTV (AF), the thickness of the posterior subcutaneous fat layer at the level of the MTV (PF), and the distance of the body from the anterior to the posterior skin surface (DAP). Other metrics shown in include: the distance from the MTV to the coccyx (MC), the distance from the MTV to the pubic symphysis (MPS), the distance from the left ischial spine to the coccyx (LISC), the distance from the right ischial spine to the coccyx (RISC), the average of the LISC and RISC (ISCavg), and the distance from the right ischial spine to the left ischial spine (DIS). For patients having MTV-LN(s), the depth of the MTV-LN (D-LN) and its closest distance to bony structure (DB-LN) were evaluated, as shown in .
The distances between specific pelvic landmarks, as well as the distances between the tumor targets and some of the landmarks were measured. These distances were selected because they were amenable to measurement by use of CT or MR imaging (and/or palpated intraoperatively) [Citation56]. Furthermore, they could be potentially useful for predicting targetability, as these landmarks are relatively adjacent to the MTV and are on a similar scale (cm) with the ultrasound beam-path.
On the co-registered PET-CT images, the MTV structure was fused into all the CT images so that the MTV can be visibly recognized on the CT images, as indicated in . These CT images were then exported as DICOM images and processed using MATLAB (R2018a, Math Works, Natick, MA, USA) and Python 2 (Anaconda3-2019.10). This procedure included the anonymization of patient information, modification of the DICOM header to be compatible with the MRgHIFU treatment planning system, and rotation of the images. Specifically, CT images were first flipped along the patients’ coronal plane to generate images with patients in both supine and prone positions. Then, rotations of the CT images along the patients’ longitudinal axis relative to the transducer were applied in degrees of 0°, ±5°, ±10°, ±15°, ±20°, ±25°, ±30°, ±35°, and ±40°, resulting in image sets representing 34 angles of rotation.
Figure 2. Representative image processing. (A) PET-CT in the axial view with the green contour indicating the location of MTV. (B) Corresponding axial CT with MTV fused as highly weighted volume. (C&D) Axial (C) and sagittal (D) view of targeting posteriorly. The white line indicates the HIFU beam-path and green cone-shaped area indicates the heating volume; the coccyx highlighted in yellow dashed line blocked the HIFU beam-path. (E&F) Axial (E) and sagittal (F) view of targeting anteriorly. The depth of the MTV represented by the yellow dashed line is longer than 8 cm; the PS highlighted by the yellow dashed contour blocked the HIFU beam-path. (G&H) Axial (G) and sagittal (H) views of the CT images rotated clockwise for 30°. The depth of the MTV represented by the yellow dashed line is less than 8 cm, and the distance between the bone and HIFU beam-path is longer than 1 cm.
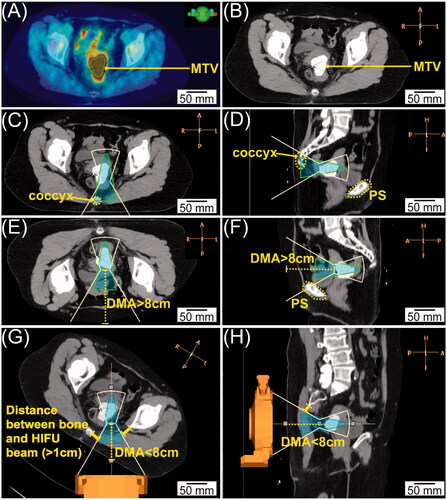
MRgHIFU treatment planning system
Each processed CT image set was then imported into a clinical MRgHIFU software program (Sonalleve® Treatment Planning System R3.5.955.1215 L2; Profound Medical, Mississauga, Canada). The Sonalleve® treatment planning program models the approximate geometries of the HIFU-induced HT beam-path, and the dimensions of heating cross-sectional beam (cell) diameter based on the vendor’s measurements. Treatment planning utilized HT cells of diameter 18 mm, 32 mm, and 44 mm corresponding to heating volumes of 16.5 ml, 44.1 ml, and 101.1 ml, respectively [Citation42]. Different cross-sectional beam diameters were used in this study since the Sonalleve® is capable of administering HT with all of the above-mentioned cross-sectional diameters [Citation41,Citation42].
Targetability evaluation
The CT image at each specific angle was evaluated in three dimensions to identify a potential acoustic beam-path that accommodated the targeting of the MTV and/or MTV-LN(s) by MRgHIFU-induced HT. HT cells were placed to cover the MTV as much as possible under the prerequisite that no bony structure was within 1 cm of the range of the acoustic window.
The MTV was not considered targetable if:
The depth of MTV was more than 9 cm from the skin surface. The Sonalleve® software had a depth limitation of 8 cm for the placement of HT cell on the centermost plane used for the feedback control, and the effective HT heating zone can extend 1 cm beyond the target plane [Citation48,Citation57].
The HIFU beam-path was blocked by or within 1 cm of any bony structures/calcifications [Citation48,Citation49,Citation58].
The whole MTV was less than 1 cm away from the skin surface. Prohibiting targeting this shallow would minimize the risk of skin burns. However, if part of the MTV was less than 1 cm from the skin but other portions of the MTV are more than 1 cm, then this MTV was considered targetable.
When administering HIFU anteriorly, the distance between the lower end of the MTV/MTV-LN and the bladder was more than 2.39 cm [Citation59]. This was based on previous reports that the interposed bowel loops could potentially be displaced out of the acoustic pathway by bladder filling, bowel massage, and/or uterine anteflexion maneuver [Citation59–65]. 2.39 cm was reported as the median distance that a bladder could distend by filling per Ju et al. [Citation59].
Duration targeting evaluation, the largest possible cell size was first evaluated. Next, if there was bone blocking the beam path or any other criteria was violated, we switched to a smaller size. If the tumor could not be targeted by the smallest 18 mm cell (i.e., comply with any of the abovementioned untargetable criteria), then those tumors were defined as ‘untargetable’. All assessments were performed in this way. Treatment plans were examined in three dimensions to assess if any of the above rules were violated. The MTV/MTV-LN was considered targetable if at least one HT cell could be placed to cover the MTV/MTV-LN without violating any one of the criteria above. Of note, for anterior targeting, the existence of intestine, bowel, or rectum within the beam-path was regarded as potentially targetable, since in clinical practice with MRgHIFU treatments, there are several potential ways to displace the interposing bowel loops out of the ultrasound beam-path, including: (1) sequential bladder filling, rectal filling, and bladder emptying (referred as bladder-rectum-bladder bowel manipulation technique) [Citation60,Citation61,Citation63,Citation66], (2) Trendelenburg position with bowel massage [Citation60], (3) use of a convex gel pad [Citation63,Citation67,Citation68], and (4) manual uterine manipulation, i.e., repositioning of the uterus into anteflexion by bladder filling, rectal filling and/or use of a vaginal speculum [Citation60]. For posterior targeting, similarly, a safe ultrasound beam-path could potentially be constructed by the use of a rectal balloon filled with degassed ultrasound gel, as done in a clinical study for rectal cancer patients treated with MRgHIFU-induced HT [Citation41]. Based on the criteria stated above, for each CT image set at a specific rotated angle, cervical cancer targets were categorized into 2 types: potential targetable or non-targetable. Due to the potential need for intervention in almost all cases, we did not include a class of ‘unconditionally targetable’.
Statistical analysis
The statistical correlation between the demographic and/or anatomical factors (height, weight, BMI, MTV, MHF, MAP, MRL, DMA, DMP, MC, MPS, AF, PF, DAP, RISC, LISC, ISCavg, and DIS) and targetability was evaluated. Additionally, the correlations between the usable cross-sectional beam diameter and all other parameters (including the above-mentioned anatomical measurements and rotated angle) were also analyzed using a logit regression model.
Mean and standard deviation (SD) of covariates such as height, weight, BMI, MTV, MHF, MAP, MRL, DMA, DMP, MC, MPS, AF, PF, DAP, RISC, LISC, ISCavg, and DIS were calculated. The Mann–Whitney U test was then applied to compare between the targetable patients and non-targetable patients. The significance level was chosen at 0.05. Additionally, nonlinear additive transformation and restricted cubic splines were applied to impute the missing BMI values in the dataset due to non-reported height values of ten patients [Citation69].
Variable selection was conducted by parametric additive models, which were determined by how well each variable could be predicted from the remaining variables. Variables were dropped in a stepwise fashion, removing the most predictable variable at each step. The remaining variables were used to predict. We selected our objective covariates by setting R2 equal to or lower than 0.3 beforehand. Next, logit regression was applied to check the significance between each selected covariate and anterior/posterior targetability. The odds ratio (OR) and the corresponding 99%, 95%, and 90% confidence interval (CI) of each variable for the anterior/posterior targetability were provided. Finally, the mixed effect model was used to check the correlation between the useable cross-sectional beam diameter and the aforementioned parameters and targeting angles.
Results
Patient demographics and lesion characteristics
shows the patient demographics. Sixty-four out of 79 patients received a combination of EBRT and HDR; 13 patients received EBRT alone; and 2 patients received HDR alone. The total dose of HDR ranged from 4.5 Gy–43.8 Gy in 1–8 fractions; and the total dose of EBRT ranged from 19.8 Gy–70.2 Gy in 10–39 fractions. Except for a lack of 10 patients’ height information, all other information was retrieved from the 79 patients. Eighty-three percent of cases were stage IIIB and 17% of cases were stage IVA. The lesion characteristics of the anatomical measurements in all patients were also presented in Supplementary Table 1.
Table 1. Patient demographics.
Targetability at different angles
Of the 79 patients, 57 (72.15%) were targetable from at least one angle. Among them, 39 (49.37%) were targetable posteriorly and 31 (39.24%) were targetable anteriorly. presents a representative CT image, which is not targetable with 0° rotation both posteriorly () and anteriorly (), but targetable posteriorly with a clockwise rotation of 30° (). After evaluation of 34 different angles of rotation for each patient, the targetability of patients rotated from 0 to 40° along the longitudinal axis in prone (targeting from anterior, ) and supine (targeting from posterior, ) orientations were calculated. Due to the reasonable symmetry of the pelvic girdle anatomy, we averaged the number of targetable patients by clockwise and counterclockwise rotations, as shown in . In the prone position, 0° rotation resulted in the maximum number of targetable patients. In the supine position, rotations of 25° and 30° resulted in the maximum number of targetable patients.
Figure 3. Targetability analysis in different HIFU angles. (A, B) Targetability of patients in different angles (rotated from 0° to 40° in both clockwise and counterclockwise directions) in both prone position (targeting from anterior, A) and supine position (targeting from posterior, B). (C) The average number of targetable MTV at each clockwise and counterclockwise rotated angle.
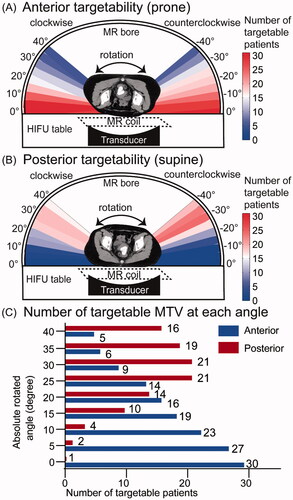
There are 53 patients that have the MTV-LN(s), among whom the average number of MTV-LN(s) in each patient is 2.60 ± 2.17. Of the 53 patients with at least one MTV-LN, 18 patients (33.96%) had targetable MTV-LN(s). Of the 18 patients who have targetable MTV-LN(s), 16 patients also had a targetable MTV. There are 4 patients whose MTV and all MTV-LN(s) were targetable. 34 patients presented MTV-LN(s) only within the pelvic region; 9 patients presented MTV-LN(s) only adjacent to the lumbar vertebrae; and 10 patients had MTV-LN(s) that were located both in the pelvic region and close to the lumbar vertebrae region. No MTV-LN(s) adjacent to the lumbar vertebrae region were targetable. All 18 cases with targetable MTV-LN(s) were located within the pelvic regions. The D-LN of the targetable (65.4 ± 31.7 mm) and non-targetable patients (95.6 ± 28.7 mm) were significantly different (p < 0.05). The DB-LN of the targetable (6.7 ± 7.6 mm) and non-targetable patients (9.7 ± 6.9 mm) were not significantly different (p = 0.96).
Logit correlations of the anatomical measurements and targetability
presents the comparisons of the parameters (height, weight, BMI, MTV, MHF, MAP, MRL, DMA, DMP, MC, MPS, AF, PF, DAP, RISC, LISC, ISCavg, and DIS) between the targetable patients and non-targetable patients. For anterior targeting, there was a significant difference (p < 0.05) in weight, BMI, MTV, DMA, AF, and DAP between the targetable and non-targetable patients. For posterior targeting, there was a significant difference (p < 0.05) in height, weight, BMI, DMP, MC, AF, PF, DAP, LISC, ISCavg, between the targetable and non-targetable patients.
Table 2. Characteristics of cervical cancer lesions in all patients.
and Supplementary Figures 1 and 2 show the statistical correlations between the anatomical measurements and targetability. Supplementary Figure 1 depicts the OR with 90%, 95%, and 99% CI of each variable. As shown in and Supplementary Figure 2, for targeting anteriorly, the DMA was significantly correlated with anterior targetability (χ2=14.3, p < 0.001, OR =1.064 with 95% CI (1.034, 1.103)). This represents that the odds of anterior targetability significantly increase when the DMA decreases. Neither MPS (χ2=3.1, p = 0.080) nor MHF (χ2=1.2, p = 0.278) were significantly correlated with the anterior targetability. For posterior targetability, MC had a significant correlation with the posterior targetability (χ2=12.7, p < 0.001, OR = 1.101 with 95% CI (1.049, 1.167)). This represents that the odds of posterior targetability decrease when MC increases. The posterior targetability was not significantly correlated with PF (χ2=1.4, p = 0.232), DIS (χ2=0, p = 0.973), or MHF (χ2=0.1, p = 0.738).
Table 3. Correlation between anatomical landmarks/demographic information and the targetability/usable cross-sectional beam diameter.
Logit correlations between the anatomical measurements and targeting cross-section diameter
and Supplementary Figure 3 present the statistical correlations between the anatomic/demographic/angle information and useable cross-sectional beam diameters. For anterior targetability, angle (p < 0.001, OR= 0.856 with 95% CI (0.835–0.878)) and DMA (p = 0.001, OR= 0.901 with 95% CI (0.846–0.959)) were significantly correlated with the anterior usable cross-section beam diameter. Neither MHF (p = 0.247, OR = 1.046 with 95% CI (0.969–1.129)) nor MPS (p = 0.248, OR= 0.947 with 95% CI (0.862–1.039)) were significantly correlated with the anterior targetability. These results illustrate that when increasing the targeting angle by 5°, the odds of being able to use a greater size of the cross-sectional beam diameter decreased by a factor of 0.856 compared to using a smaller targeting diameter. When the DMA increased by 1 mm, the odds of using a larger cross-sectional beam diameter decreased by a factor of 0.901 compared to the odds of using a smaller targeting diameter.
For the posterior targetability, the MRL (p < 0.001, OR = 1.028 with 95% CI (1.011–1.044)) and MC (p = 0.026, OR = 0.968 with 95% CI (0.942–0.996)) were significantly correlated with the usable cross-sectional beam diameter, while neither the rotated angle (p = 0.264, OR = 1.004 with 95% CI (0.997–1.012)) nor weight (p = 0.355, OR = 0.997 with 95% CI (0.990–1.004)) were significantly correlated with the posterior useable cross-sectional beam diameter. The results indicate that for posterior targeting, when the MRL increased by 1 mm, the odds of being able to use a larger beam diameter increase by a factor of 1.028 compared to the odds of using a smaller targeting diameter. When increasing the MC by 1 mm, the odds of using a larger targeting diameter decrease by a factor of 0.968 compared to the odds of using a smaller beam cross-sectional diameter.
Discussion
For the cases analyzed in this study, over 70% of the cervical cancer patients (stage IIIB–IVA) had a potentially targetable primary lesion by MRgHIFU-induced HT from at least one angle with no or minimal intervention. These results support proceeding into clinical trials to further evaluate the efficacy of MRgHIFU HT in the treatment of late-stage cervical cancer patients. No rotation (in the prone orientation) or a rotation of 25°–30° (in the supine orientation) had the highest anterior or posterior targetability rate, respectively. Additionally, the anterior targetability was statistically correlated with the DMA. The posterior targetability was statistically correlated with the MC. In an anterior setup, the usable beam cross-sectional diameter was statistically correlated with the rotated angle and DMA. In the posterior setup, the usable cross-sectional beam diameter was statistically correlated with the MC and the MRL. This analysis provides potential guidance for optimal patient setup and treatment workflow, as well as metrics which could be useful for screening patients from pretreatment images.
Our study provides a method for virtual treatment planning utilizing the preexisting PET-CT images in the place of standard MRI for MRgHIFU-induced HT in the evaluation of both primary cervical cancer lesions and metastatic lymph nodes. By reviewing the diagnostic/pretreatment images, this strategy potentially spares patients from additional imaging or administration of sedative drugs or contrast agents. In this way, we created a potentially useful workflow for determining an optimal treatment setup that could facilitate the evaluation of the targetability of the MRgHIFU HT in late-stage cervical cancer patients. Additionally, we analyzed the potential correlation between anatomical landmarks of the pelvic girdle and the targetability, so that some simple measurements might provide useful information to clinicians when screening candidates of MRgHIFU HT or gauging the targetability. Although the approach and the predictability of selected anatomical measurements still need to be tested on a treated patient population, the accurate delineation of the target lesion (MTV) by PET and the stark contrast of bony structures in CT facilitate the recognition of any obstructive bony structures within ultrasound beam-path.
Overall, our study showed that 72.15% of patients have targetable primary pelvic lesion, higher than reported in a previous study by Giles et al. [Citation46], which evaluated recurrent gynecological tumors, reporting that 55% of patients were targetable by MRgHIFU thermal ablation when bladder and/or rectum filling were assumed. Several factors might be related to this variation. First, the treatment objective of Giles et al. [Citation46] was ablation, which differed from this current study (HT). Usable beam sizes are different, with the Sonalleve® system providing 4 mm, 8 mm, 12 mm, or 16 mm as the axial diameters for the ablation therapy and 18 mm, 32 mm, 44 mm, or 58 mm the selectable diameters for HT. The HIFU beam-path requirements (i.e., useable cross-sectional beam diameter, angle) also differ between thermal ablation and HT. Furthermore, the allowed set-up position(s) in the previous study were more limited than this one. Specifically, Giles et al. only analyzed one treatment position per patient by minimizing the distance between the target lesion and the transducer. In contrast, our study systematically evaluated the targetability at 34 different angles, which subsequently increased the probability of obtaining a targetable cell. The previous study also covered all recurrent gynecological tumors, while our study only focused on late-stage cervical cancer. Finally, the previous prospective study recruited a smaller cohort than this study, which might have introduced a higher degree of sample bias.
Another study by J Shim et al. [Citation48] addressed virtual treatment planning of MRgHIFU thermal ablation in pediatric tumors, including a sub-cohort of pelvic tumors. The targetability of the pelvis tumor was 95% (N = 28, primary sarcoma) [Citation48]. The difference between J Shim et al. and our study could be the intrinsic difference in anatomical locations or sizes between sarcoma (surrounding the bone) and cervical cancer (within the pelvic girdle), as well as differences in the treatment regimen (HIFU thermal ablation vs. HT) as discussed above. Additionally, J Shim et al. only evaluated two orthogonal (two-dimensional) images for assessing the ultrasound beam-path compared to our three-dimensional evaluation.
Our results also demonstrated that a rotation of 0°–5°and 25°–30° had the highest anterior and posterior targetability rate, respectively. These results imply that when targeting from the anterior direction, the optimal prone position might be lying flat. In contrast, when targeting posteriorly, a slight decubitus rotation was needed to optimize the accommodation of an acoustic beam-path without blockage, and to provide a good coupling of the patients’ skin surface with the transducer. Both setups are potentially feasible to implement in the clinic.
In addition, this study further evaluated possible relationships between anatomical structures and targetability by statistical comparison. Two different statistical analyses were performed. The Mann–Whitney U test evaluated statistical differences between targetable vs. non-targetable cohorts. This analysis revealed statistically significant differences in the weight, BMI, MTV, DMA, AF, and DAP between targetable and non-targetable patients when focusing on the anterior targetability (). Most anteriorly targetable patients either had a relatively smaller body mass/dimension and/or a larger MTV. For posterior targetability, the height, weight, BMI, DMP, MC, AF, PF, DAP, LISC, and ISCavg all showed statistically significant differences between targetable and non-targetable patients. We could explain these differences in two ways. Similar to the anterior targetability, a lower weight, height, BMI, DMP, AF, and PF might reflect the ability of the ultrasound beam-path to reach the MTV target. Furthermore, a shorter MC and/or a larger LISC/ISCavg implied a larger acoustic window between the bony structures, thus allowing greater treatment access to the target.
As opposed to the Mann–Whitney U test, the logit regression model illustrated a statistical correlation for assessing the predictability of targetability/utilizable cross-sectional diameter by anatomical measurement/demographic information. A larger DMA or MC was statistically correlated with lower odds of targetability from an anterior or posterior orientation, respectively. A smaller rotated angle or a shorter DMA was statistically correlated with a larger usable cross-sectional beam diameter. A shorter MC or a larger MRL were statistically correlated with a larger usable cross-sectional beam diameter. The results were as expected, since a larger MRL implies an increased dimension of the MTV in the coronal axis, which in turn, allows for a larger useable cross-sectional beam diameter. Similarly, a shorter DMA, smaller rotated angle, and/or shorter MC all potentially shorten the relative distance from the transducer to the MTV, thus allowing a larger usable beam diameter. These results illustrate a consistent statistical correlation between geometrical measurements and targetability, thus implying that a careful evaluation of the bony anatomy relative to the primary tumor could potentially provide useful information to clinicians when determining targetability by MRgHIFU-mediated HT.
This study also demonstrated that, in patients with MTV-LNs, 33.6% were targetable by MRgHIFU-induced HT, lower than the primary MTV. Possible reasons might include that the MTV-LN were more likely to be located adjacent to the lumbar vertebra (N = 19) or the pelvic girdle (N = 44), for which an adequate acoustic pathway was difficult to define. Bing et al. [Citation45] analyzed the targetability of metastases lesions located in proximity to the vertebra [Citation45], and included additional interventions (articular hydro-dissection, bone-consolidation) that we did not consider. Such interventions could potentially increase the targetability of the MTV-LNs located adjacent to the lumbar vertebra. We did not include assessment of patient rotation in the targetability of the MTV-LNs because 35.84% of the MTV-LNs were located close to the lumbar vertebrae, thus no amount of rotation could enhance the targetability. Furthermore, a smaller sample size (53 patients) and location variability of MTV-LNs decreased the applicability of the logit regression model, so we did assess the logit correlation between the targetability of the lymph nodes and the geometrical measurements. Furthermore, due to the extended duration required for HT, it might be infeasible and inefficient to perform HT to all MTV-LNs with the current system. With that said, as the 10-year overall survival rate for locally advanced cervical cancer patients receiving chemo-RT with LN size < 10 mm and ≥ 10 mm was 89.2% and 64.1% (p < 0.05), respectively [Citation70], applying HT to the primary lesion and lymph nodes ≥10 mm could potentially reduce the tumor burden and have clinical benefit.
Our study has several limitations. First, the analyzed cohort was limited to late-stage cervical cancer patients receiving RT at a single treatment center, so this might limit the generalizability of our results and applicability to the greater cervical cancer patient population at different stages and/or other institutions. However, this study was reasonably representative of a specific patient population (stage IIIB to IVA) that could benefit from additional treatment options, in contrast to early stage cervical cancer patients, who have relatively satisfactory prognosis after surgery and/or RT [Citation71,Citation72]. Second, our analysis did not calculate the targetable volumes of each tumor but only calculated the percentage of the targetable patients by the treatment planning system. While calculating the targetable volume might be useful, the heating distributions of HT can differ in various scenarios. For example, neighboring structures, physiological motion, and the utilized interventions might all exert different effects on the heating dimensions in practice. Hence the achievable heating volume needs to be assessed on a case-by-case basis. Other advanced possible interventions, such as respiratory/cardiovascular motion compensation, adjunctive hydro-carbo-dissection, or consolidation, etc., were also not considered. This was due to the fact that previous studies had shown that MRgHIFU induced HT could be performed in the pelvic region without the above-mentioned interventions in porcine models [Citation40,Citation41] and human patients [Citation43]. MRgHIFU HT with minimal intervention was preferable as it limits the patients’ risk and potentially increases patient comfort, which might lead to higher compliance.
Furthermore, we analyzed the targetability under the assumption that heating part of the tumor might have therapeutic benefits for the tumor response, so we did not intend to cover the whole tumor volume in one HT session. This method was selected for several reasons. In a previous clinical trial [Citation73], when heating tumors, HT was only directed to the most bulky area. This targeting strategy (aiming to heat only part of the tumor in one HT session) is also adopted in an ongoing clinical trial using MRgHIFU induced HT for rectal cancer therapy [Citation43]. Furthermore, the irregular shape (different from the cone shape of the heating volume of the heated cell of Sonalleve®), depth of the lesion, and pelvic bone all make it challenging to cover the whole tumor without heating the surrounding normal tissues in one session. It is also well-established in pre-clinical studies that ultrasound induced HT is able to activate the immune system by accelerating antigens presentation and releasing of anti-tumor cytokines [Citation74]. Finally, in our planned subsequent phase I clinical study, we intend to cover the target lesions in separate HT sessions and treat different areas throughout the patients’ course of treatment. Therefore, we choose to only heat a portion of the tumor as a constant criteria in this study. Nevertheless, further studies should be performed to evaluate the percentage of the heatable tumor volume and/or the impact of partial target therapy.
Another issue is that, as ultrasound waves cannot penetrate air, acoustic mismatches near the interfaces between air (or air containing structures) and tissue can cause unwanted heating of sensitive tissue layers [Citation41,Citation68]. As this can result in undesired burning of the surrounding normal tissue, potentially air-containing bowel loops cannot be present within the acoustic beampath and have to be displaced out of ultrasound pathway [Citation60–62]. However, we assumed that patients with digestive tracts present in the acoustic pathway were targetable after bowel manipulation [Citation60–63,Citation66]. This assumption was based on previous studies which have proposed and used an array of bowel manipulation techniques that could displace the bowel loops out of the way of the ultrasound beampath and increase patients’ eligibility rates from 66.7%–82% to 89.3%–100% [Citation60,Citation61]. While it is still possible that a small portion of patients might have bowels that could not be displaced out of the beam path by any manipulation technique, we believe our analysis represents the majority of the patient population. With that said, the feasibility and effectiveness of these relatively newly emerged bowel manipulation techniques require further validation and optimization, which are out of the scope of this study.
Furthermore, in this study, we also did not evaluate any practical method of applying RT and HT simultaneously, but only discussed the targetability of MRgHIFU induced HT. In past clinical studies utilizing HT, pre-RT [Citation25–27,Citation35,Citation75,Citation76], simultaneous [Citation22,Citation24,Citation29,Citation30,Citation77–80], and post-RT [Citation23,Citation27,Citation34,Citation75,Citation81–84] HT have all been applied and radiosensitization effects have been demonstrated in all of these sequences. Thus, to exert radiosensitization, HT is not required to be applied with RT simultaneously, as long as the time interval between RT and HT is relatively short (<4 h). As for the time of the transmission from one area to another area, it could depend on a number of factors. Moving from one site to another depends on the time needed to adjust the patients’ position with satisfactory compliance and comfort, as well as the time the operators need to find an accessible ultrasound pathway to the tumor. This includes performing a coupling using gel pads, acquiring the planning MR images, and making positional adjustments/manipulations based on any structures in the ultrasound beampath). For multiple HT sessions in the same patient, different sessions could be performed on different days throughout the treatment course [Citation22]. It should also be noted that to apply RT + HT simultaneously, an MR-guided RT capable treatment unit would need to be merged with MRgHIFU induced HT devices. Currently, no such device exists on the market, and, although we believe this hardware combination deserves further study (i.e., design of the equipment room, building materials, etc.), this is out of the scope of our current study.
Another issue that worth mentioning is that the posterior potion of the pelvic cavity is rich in nerve bundles, so targeting from the posterior direction requires extra caution (good coupling, careful examination of acoustic pathway); otherwise, nerve injury is possible, especially when ablative temperatures (∼80 °C) are required for protein denaturation. Despite this, we expect that MRgHIFU induced HT can feasibly be performed to late stage cervical cancer for a number of reasons. First, we utilized a relatively mild temperature (40 °C–45 °C), a continuous ultrasound wave, and passive cavitation monitoring in the transducer that disables sonication when detecting cavitation above a threshold, all of which create multiple overlapping layers of protection to decrease the probability of accidental burning injuries/mechanical damage to occur. Another reason is that, in line with other clinical trials [Citation43,Citation67,Citation68], we intend to treat patients consciously. Patient alertness decreases the likelihood of nerve burning injury, since the sonication power can be immediately cut off if they feel any uncomfortable sensation via a patient held trigger which immediately stops the sonication. Additionally, the relative safety of heating patients posteriorly has been evaluated by a group of researchers who have adopted this position and noted its feasibility in the treatment of recurrent rectal cancer patients without reporting severe nerve complications [Citation41,Citation43,Citation85]. Furthermore, in our previous study, 30-min HT was also performed to porcine pelvic muscles [Citation40] in an orientation reflective of a human patient prone position without showing any burning injury to the surrounding tissue or nerve injury. These results thus imply the feasibility and safety of targeting in the supine position (posteriorly) in clinical practice. Finally, we did not take into account the possible tumor volume shrinkage during/after RT, but only evaluated the targetability based on the pretreatment/diagnostic PET-CT images. Future work is required to assess the targetability during the course of RT due to potential tumor regression/shrinkage.
Despite these limitations, the results of this study provide important evidence that most late-stage cervical cancer primaries are targetable by MRgHIFU HT, which supports the progression into a clinical trial. We have evaluated the feasibility and safety of applying MRgHIFU induced HT for a variety of clinically mimicking pelvic geometries in a porcine model [Citation40], and also characterized the heating volume of the MRgHIFU-induced HT [Citation86]. Furthermore, the feasibility of using MRgHIFU for treating rectal cancer patients was also evaluated by Chu et al. [Citation43]. Based on these results [Citation40,Citation43], it is our intention to perform a subsequent phase I clinical trial for safety evaluation, applying HT for 30 min–60 min in locally advanced cervical cancer patients who are also receiving RT. Targeting the metastatic lymph nodes by MRgHIFU-induced HT might require further evaluation or a different approach. Our results also provide clinical guidance for potentially optimal patient setup and alignment for the MRgHIFU HT. The results also suggest that simple measurements from the patients’ images/demographics might be useful as a screening tool to assess patients’ targetability by MRgHIFU HT. Overall, the results of this study promote the use of MRgHIFU to treat late-stage cervical cancer.
Conclusion
Most late-stage cervical cancer was targetable by MRgHIFU-induced HT in at least one orientation/angle assuming minimal intervention. No rotation had the highest chance of anterior targetability, while a rotation of 25°–30° had the highest chance of posterior targetability. Patient characteristics and anatomical geometric factors could be used to predict the anterior or posterior targetability and the targeting cross-sectional diameter. The results of this study support proceeding into human clinical trials to further evaluate the efficacy of MRgHIFU HT in the treatment of late-stage cervical cancer patients.
Supplemental Material
Download PDF (437.7 KB)Acknowledgments
The authors thank Dr. Jochen Cammin for the providing Matlab code and Ari Partanen at Profound Medical Corp. for technical assistance.
Disclosure statement
The authors have no interest to declare.
Additional information
Funding
Reference
- Bray F, Ferlay J, Soerjomataram I, et al. Global cancer statistics 2018: GLOBOCAN estimates of incidence and mortality worldwide for 36 cancers in 185 countries. CA Cancer J Clin. 2018;68:394–424.
- Bhatla N, Aoki D, Sharma DN, et al. Cancer of the cervix uteri. Int J Gynecol Obstet. 2018;143:22–36.
- Bhatla N, Berek JS, Cuello Fredes M, et al. Revised FIGO staging for carcinoma of the cervix uteri. Int J Gynecol Obstet. 2019;145:129–135.
- Quinn MA, Benedet JL, Odicino F, et al. Carcinoma of the cervix uteri. Int J Gynecol Obstet. 2006;95:S43–S103.
- Koh W-J, Abu-Rustum NR, Bean S, et al. Cervical cancer, version 3.2019, NCCN clinical practice guidelines in oncology. J Natl Compr Canc Netw. 2019;17:64–84.
- Schwarz JK, Siegel BA, Dehdashti F, et al. Metabolic response on post-therapy FDG-PET predicts patterns of failure after radiotherapy for cervical cancer. Int J Radiat Oncol Biol Phys. 2012;83:185–190.
- Vistad I, Fosså SD, Dahl AAA. A critical review of patient-rated quality of life studies of long-term survivors of cervical cancer. Gynecol Oncol. 2006;102:563–572.
- Greimel ER, Winter R, Kapp KS, et al. Quality of life and sexual functioning after cervical cancer treatment: a long-term follow-up study. Psychooncology. 2009;18:476–482.
- Kirwan JM, Symonds P, Green JA, et al. A systematic review of acute and late toxicity of concomitant chemoradiation for cervical cancer. Radiother Oncol. 2003;68:217–226.
- Elghamrawi KA, Haggag MH, Habib EE. Treatment complications among long-term survivors of cervical cancer: treated by surgery or radiotherapy. Oncol Rev. 2011;5:261–266.
- Mallory M, Gogineni E, Jones GC, et al. Therapeutic hyperthermia: the old, the new, and the upcoming. Crit Rev Oncol Hematol. 2016;97:56–64.
- Datta NR, Ordóñez SG, Gaipl US, et al. Local hyperthermia combined with radiotherapy and-/or chemotherapy: recent advances and promises for the future. Cancer Treat Rev. 2015;41:742–753.
- Issels RD, Lindner LH, Verweij J, et al. Neo-adjuvant chemotherapy alone or with regional hyperthermia for localised high-risk soft-tissue sarcoma: a randomised phase 3 multicentre study. Lancet Oncol. 2010;11:561–570.
- Lutgens L, van der Zee J, Pijls-Johannesma M, et al. Combined use of hyperthermia and radiation therapy for treating locally advanced cervix carcinoma. Cochrane Database Syst Rev. 2010:CD006377.
- Datta NR, Bose AK, Kapoor HK, et al. Thermoradiotherapy in the management of carcinoma cervix (stage IIIB): a controlled clinical study. Indian Med Gaz. 1987;121:68–71.
- Harima Y, Nagata K, Harima K, et al. A randomized clinical trial of radiation therapy versus thermoradiotherapy in stage IIIB cervical carcinoma. Int J Hyperth. 2001;17:97–105.
- Sharma S, Singhal S, Sandhu APS, et al. Local thermo-radiotherapy in carcinoma cervix: improved local control versus increased incidence of distant metastasis . Asia Oceania J Obstet Gynaecol. 1991;17:5–12.
- van der Zee J, González González D, van Rhoon GC, et al. Comparison of radiotherapy alone with radiotherapy plus hyperthermia in locally advanced pelvic tumours: a prospective, randomised, multicentre trial. Dutch Deep Hyperthermia Group. Lancet. 2000;355:1119–1125.
- Vasanthan A, Mitsumori M, Park JH, et al. Regional hyperthermia combined with radiotherapy for uterine cervical cancers: a multi-institutional prospective randomized trial of the international atomic energy agency. Int J Radiat Oncol Biol Phys. 2005;61:145–153.
- Hong-Wei C. A randomized trial of hyperthermia-radiochemotherapy for uterine cervix cancer. Clin J Clin Oncol. 1997;24:249–251.
- Van Der Zee J, González González D. The Dutch Deep Hyperthermia trial: results in cervical cancer. Int J Hyperthermia. 2002;18:1–12.
- Varma S, Myerson R, Moros E, et al. Simultaneous radiotherapy and superficial hyperthermia for high-risk breast carcinoma: a randomised comparison of treatment sequelae in heated versus non-heated sectors of the chest wall hyperthermia. Int J Hyperthermia. 2012;28:583–590.
- Kapp DS, Petersen IA, Cox RS, et al. Two or six hyperthermia treatments as an adjunct to radiation therapy yield similar tumor responses: results of a randomized trial. Int J Radiat Oncol Biol Phys. 1990;19:1481–1495.
- Algan O, Fosmire H, Hynynen K, et al. External beam radiotherapy and hyperthermia in the treatment of patients with locally advanced prostate carcinoma. Cancer. 2000;89:399–403.
- Harari PM, Hynynen KH, Roemer RB, et al. Development of scanned focussed ultrasound hyperthermia: clinical response evaluation. Int J Radiat Oncol Biol Phys. 1991;21:831–840.
- Guthkelch AN, Carter LP, Cassady JR, et al. Treatment of malignant brain tumors with focused ultrasound hyperthermia and radiation: results of a phase I trial. J Neurooncol. 1991;10:271–284.
- Marmor JB, Hahn GM. Combined radiation and hyperthermia in superficial human tumors. Cancer. 1980;46:1986–1991.
- Corry PM, Spanos WJ, Tilchen EJ, et al. Combined ultrasound and radiation therapy treatment of human superficial tumors. Radiology. 1982;145:165–169.
- Hurwitz MD, Kaplan ID, Hansen JL, et al. Hyperthermia combined with radiation in treatment of locally advanced prostate cancer is associated with a favourable toxicity profile. Int J Hyperthermia. 2005;21:649–656.
- Hurwitz MD, Kaplan ID, Hansen JL, et al. Association of rectal toxicity with thermal dose parameters in treatment of locally advanced prostate cancer with radiation and hyperthermia. Int J Radiat Oncol Biol Phys. 2002;53:913–918.
- Van Der Zee J. Heating the patient: a promising approach? Ann Oncol. 2002;13:1173–1184.
- Vernon CC, Hand JW, Field SB, et al. Radiotherapy with or without hyperthermia in the treatment of superficial localized breast cancer: results from five randomized controlled trials. International Collaborative Hyperthermia Group. Int J Radiat Oncol Biol Phys. 1996;35:731–744.
- Huilgol NG, Gupta S, Sridhar CR. Hyperthermia with radiation in the treatment of locally advanced head and neck cancer: a report of randomized trial. J Can Res Ther. 2010;6:492–496.
- Dunlop PR, Hand JW, Dickinson RJ, et al. An assessment of local hyperthermia in clinical practice. Int J Hyperthermia. 1986;2:39–50.
- Woeber K. Combination of ultrasound and X-ray radiation in the treatment of cancer. Int J Phys Med. 1960;4:10–19.
- Crezee H, Van Leeuwen CM, Oei AL, et al. Thermoradiotherapy planning: integration in routine clinical practice. Int J Hyperthermia. 2016;32:41–49.
- Fessenden P, Hand JW. Hyperthermia therapy physics. In: Smith AR, editor. Radiat Ther Physics Med Radiol (Diagnostic Imaging Radiat Oncol). Berlin: Springer-Verlag; 1995. p. 315–363.
- Diederich CJ, Hynynen K. Ultrasound technology for hyperthermia. Ultrasound Med Biol. 1999;25:871–887.
- Zhu L, Altman MB, Laszlo A, et al. Ultrasound hyperthermia technology for radiosensitization. Ultrasound Med Biol. 2019;45:1025–1043.
- Zhu L, Partanen A, Talcott MR, et al. Feasibility and safety assessment of magnetic resonance-guided high-intensity focused ultrasound (MRgHIFU)-mediated mild hyperthermia in pelvic targets evaluated using an in vivo porcine model. Int J Hyperthermia. 2019;36:1147–1159.
- Chu W, Staruch RM, Pichardo S, et al. Magnetic resonance-guided high-intensity focused ultrasound hyperthermia for recurrent rectal cancer: MR thermometry evaluation and preclinical validation. Int J Radiat Oncol Biol Phys. 2016;95:1259–1267.
- Tillander M, Hokland S, Koskela J, et al. High intensity focused ultrasound induced in vivo large volume hyperthermia under 3D MRI temperature control. Med Phys. 2016;43:1539–1549.
- Chu W, Staruch RM, Pichardo S, et al. Safety and feasibility of MR-HIFU mild hyperthermia with radiation and chemotherapy for recurrent rectal cancer. Society for Thermal Medicine – 2018 Annual Meeting; Tucson, Arizona. 2018. p. 82.
- Kim A. A phase I study of lyso-thermosensitive liposomal doxorubicin and MR-HIFU for pediatric refractory solid tumors. Clinicaltrials.Gov. 2015:2015–2020.
- Bing F, Vappou J, de Mathelin M, et al. Targetability of osteoid osteomas and bone metastases by MR-guided high intensity focused ultrasound (MRgHIFU). Int J Hyperthermia. 2018;35:471–479.
- Giles SL, Imseeh G, Rivens I, et al. MR guided high intensity focused ultrasound (MRgHIFU) for treating recurrent gynaecological tumours: a pilot feasibility study. Br J Radiol. 2019;92:20181037.
- Tung S, Fahy AS, Lamberti-Pasculli M, et al. Magnetic resonance-guided high-intensity focused ultrasound (MRgHIFU) virtual treatment planning for abdominal neuroblastoma utilizing retrospective diagnostic 3D CT images. J Pediatr Hematol Oncol. 2019;41:e443–e449.
- Shim J, Staruch RM, Koral K, et al. Pediatric sarcomas are targetable by MR-guided high intensity focused ultrasound (MR-HIFU): anatomical distribution and radiological characteristics. Pediatr Blood Cancer. 2016;63:1753–1760.
- Seward MC, Daniel GB, Ruth JD, et al. Feasibility of targeting canine soft tissue sarcoma with MR-guided high-intensity focused ultrasound. Int J Hyperth. 2018;35:205–215.
- Beserra A, Pichardo S, Kisselgoff D, et al. Targeting feasibility evaluation of magnetic resonance-guided focused ultrasound in the management of osteomyelitis: a virtual treatment planning study in 75 patients. Int J Hyperth. 2019;36:1012–1023.
- Machtinger R, Inbar Y, Ben-Baruch G, et al. MRgFUS for pain relief as palliative treatment in recurrent cervical carcinoma: a case report. Gynecol Oncol. 2008;108:241–243.
- Abel M, Ahmed H, Leen E, et al. Ultrasound-guided trans-rectal high-intensity focused ultrasound (HIFU) for advanced cervical cancer ablation is feasible: a case report. J Ther Ultrasound. 2015;3:3–6.
- Kim BS, Kim IJ, Kim SJ, et al. The prognostic value of the metabolic tumor volume in FIGO stage IA to IIB cervical cancer for tumor recurrence: Measured by F-18 FDG PET/CT. Nucl Med Mol Imaging. 2011;45:36–42.
- Miller TR, Grigsby PW. Measurement of tumor volume by PET to evaluate prognosis in patients with cervical cancer. Int J Radiat Oncol. 2001;51:222.
- Chung HH, Kim JW, Han KH, et al. Prognostic value of metabolic tumor volume measured by FDG-PET/CT in patients with cervical cancer. Gynecol Oncol. 2011;120:270–274.
- Maldonado PA, Stuparich MA, McIntire DD, et al. Proximity of uterosacral ligament suspension sutures and S3 sacral nerve to pelvic landmarks. Int Urogynecol J. 2017;28:77–84.
- Huisman M, Lam MK, Bartels LW, et al. Feasibility of volumetric MRI-guided high intensity focused ultrasound (MR-HIFU) for painful bone metastases. J Ther Ultrasound. 2014;2:16.
- Hurwitz MD, Ghanouni P, Kanaev SV, et al. Magnetic resonance-guided focused ultrasound for patients with painful bone metastases: phase III trial results. J Natl Cancer Inst. 2014;106:1–9.
- Ju SG, Huh SJ, Shin JS, et al. Different effects of bladder distention on point A-based and 3D-conformal intracavitary brachytherapy planning for cervical cancer. J Radiat Res. 2013;54:349–356.
- Verpalen IM, van 't Veer-Ten Kate M, de Boer E, et al. Development and clinical evaluation of a 3-step modified manipulation protocol for MRI-guided high-intensity focused ultrasound of uterine fibroids. Eur Radiol. 2020;30:3869–3878.
- Park MJ, Kim YS, Rhim H, et al. Technique to displace bowel loops in MRI-guided high-intensity focused ultrasound ablation of fibroids in the anteverted or anteflexed uterus. Am J Roentgenol. 2013;201:761–765.
- Kim YS, Lim HK, Rhim H. Magnetic resonance imaging-guided high- intensity focused ultrasound ablation of uterine fibroids: effect of bowel interposition on procedure feasibility and a unique bowel displacement technique. PLoS One. 2016;11:e0155670.
- Duc NM, Keserci B. Review of influential clinical factors in reducing the risk of unsuccessful MRI-guided HIFU treatment outcome of uterine fibroids. Diagn Interv Radiol. 2018;24:283–291.
- Stewart AJ, Cormack RA, Lee H, et al. Prospective clinical trial of bladder filling and three-dimensional dosimetry in high-dose-rate vaginal cuff brachytherapy. Int J Radiat Oncol Biol Phys. 2008;72:843–848.
- Kim RY, Shen S, Lin HY, et al. Effects of bladder distension on organs at risk in 3D image-based planning of intracavitary brachytherapy for cervical cancer. Int J Radiat Oncol Biol Phys. 2010;76:485–489.
- Kim Y. Sun Clinical application of high-intensity focused ultrasound ablation for uterine fibroids. Biomed Eng Lett. 2017;7:99–105.
- Nyapathy V, Polina L. MRgFUS treatment of uterine fibroid in a nulliparous woman with acute retention of urine. J Radiol Case Rep. 2012;6:1–8.
- Hesley GK, Felmlee JP, Gebhart JB, et al. Noninvasive treatment of uterine fibroids: early mayo clinic experience with magnetic resonance imaging-guided focused ultrasound. Mayo Clin Proc. 2006;81:936–942.
- Harrell FE. Regression modeling strategies: with applications to linear models, logistic and ordinal regression, and survival analysis. 2nd ed. New York, NY: Springer; 2017.
- Oh J, Seol KH, Choi YS, et al. Clinical significance of lymph node size in locally advanced cervical cancer treated with concurrent chemoradiotherapy. Yeungnam Univ J Med. 2019;36:115–123.
- Landoni F, Maneo A, Colombo A, et al. Randomised study of radical surgery versus radiotherapy for stage Ib-IIa cervical cancer. Lancet. 1997;350:535–540.
- Suprasert P, Srisomboon J, Charoenkwan K, et al. Twelve years experience with radical hysterectomy and pelvic lymphadenectomy in early stage cervical cancer. J Obstet Gynaecol. 2010;30:294–298.
- Dharmaiah S, Zeng J, Rao VS, et al. Clinical and dosimetric evaluation of recurrent breast cancer patients treated with hyperthermia and radiation. Int J Hyperth. 2019;36:986–992.
- Ho YJ, Li JP, Fan CH, et al. Ultrasound in tumor immunotherapy: current status and future developments. J Control Release. 2020;323:12–23.
- Corry PM, Barlogie B, Tilchen EJ, et al. Ultrasound-induced hyperthermia for the treatment of human superficial tumors. Int J Radiat Oncol Biol Phys. 1982;8:1225–1229.
- Bornstein BA, Zouranjian PS, Hansen JL, et al. Local hyperthermia, radiation therapy, and chemotherapy in patients with local-regional recurrence of breast carcinoma. Int J Radiat Oncol Biol Phys. 1993;25:79–85.
- Diederich CJ, Wootton J, Prakash P, et al. Catheter-based ultrasound hyperthermia with HDR brachytherapy for treatment of locally advanced cancer of the prostate and cervix. Proc SPIE Int Soc Opt Eng. 2011;7901:79010O.
- Hurwitz MD, Hansen JL, Prokopios-Davos S, et al. Hyperthermia combined with radiation for the treatment of locally advanced prostate cancer: long-term results from Dana-Farber Cancer Institute study 94-153. Cancer. 2011;117:510–516.
- Fosmire H, Hynynen K, Drach GW, et al. Feasibility and toxicity of transrectal ultrasound hyperthermia in the treatment of locally advanced adenocarcinoma of the prostate. Int J Radiat Oncol Biol Phys. 1993;26:253–259.
- Myerson RJ, Straube WL, Moros EG, et al. Simultaneous superficial hyperthermia and external radiotherapy: report of thermal dosimetry and tolerance to treatment. Int J Hyperth. 1999;15:251–266.
- Xia T, Sun Q, Shi X, et al. Relationship between thermal parameters and tumor response in hyperthermia combined with radiation therapy. Int J Clin Oncol. 2001;6:138–142.
- Emami B, Myerson RJ, Cardenes H, et al. Combined hyperthermia and irradiation in the treatment of superficial tumors: results of a prospective randomized trial of hyperthermia fractionation (1/wk vs 2/wk). Int J Radiat Oncol Biol Phys. 1992;24:145–152.
- Kapp DS, Cox RS, Fessenden P, et al. Parameters predictive for complications of treatment with combined hyperthermia and radiation therapy. Int J Radiat Oncol Biol Phys. 1992;22:999–1008.
- Jones EL, Oleson JR, Prosnitz LR, et al. Randomized trial of hyperthermia and radiation for superficial tumors. J Clin Oncol. 2005;23:3079–3085.
- Chu W, Staruch R, Pichardo S, et al. MR-HIFU mild hyperthermia for locally recurrent rectal cancer: temperature mapping and heating quality in first patient. 12th International Congress of Hyperthermic Oncology; New Orleans, LA; 2016. p. 144.
- Zhu L, Lam D, Pacia CP, et al. Characterization of magnetic resonance-guided high-intensity focused ultrasound (MRgHIFU)-induced large-volume hyperthermia in deep and superficial targets in a porcine model. Int J Hyperthermia. 2020;37:1159–1173.