Abstract
Background
Metastatic prostate cancer in bone is difficult to treat as the tumor cells are relatively resistant to hormonal or chemotherapies when compared to primary prostate cancer. Irreversible electroporation (IRE) is a minimally invasive ablation procedure that has potential applications in the management of prostate cancer in bone. However, a common limitation of IRE is tumor recurrence, which arises from incomplete ablation that allows remaining cancer cells to proliferate. In this study, we combined IRE with radium-223 (Ra-223), a bone-seeking radionuclide that emits short track length alpha particles and thus is associated with reduced damage to the bone marrow and evaluated the impact of the combination treatment on bone-forming prostate cancer tumors.
Methods
The antitumor activity of IRE and Ra-223 as single agents and in combination was tested in vitro against three bone morphogenetic protein 4 (BMP4)-expressing prostate cancer cell lines (C4-2B–BMP4, Myc-CaP–BMP4, and TRAMP-C2–BMP4). Similar evaluation was performed in vivo using a bone-forming C4-2B–BMP4 tumor model in nude mice.
Results
IRE and Ra-223 as monotherapy inhibited prostate cancer cell proliferation in vitro, and their combination resulted in significant reduction in cell viability compared to monotherapy. In vivo evaluation revealed that IRE with single-dose administration of Ra-233, compared to IRE alone, reduced the rate of tumor recurrence by 40% following initial apparent complete ablation and decreased the rate of proliferation of incompletely ablated tumor as quantified in Ki-67 staining (53.58 ± 16.0% for IRE vs. 20.12 ± 1.63%; for IRE plus Ra-223; p = 0.004). Histological analysis qualitatively showed the enhanced killing of tumor cells adjacent to bone by Ra-223 compared to those treated with IRE alone.
Conclusion
IRE in combination with Ra-223, which enhanced the destruction of cancer cells that are adjacent to bone, resulted in reduction of tumor recurrence through improved clearance of proliferative cells in the tumor region.
Introduction
Bone metastasis is frequently observed in advanced prostate cancer. The development of bone metastases is often indicative of poor prognosis [Citation1] and is associated with debilitating pain that drastically reduces quality of life [Citation2–4]. Current approaches to the management of bone metastases are largely palliative [Citation5] and typically involve the eradication of invading tumor cells to stabilize bone structure, alleviate pain, and improve quality of life [Citation6]. External beam radiotherapy remains the most accepted first-line treatment for metastatic bone disease although pain relief is often temporary [Citation7]. However, potential complications from external beam radiotherapy, such as bone marrow suppression, may make focal therapies preferable. Surgery as well as a variety of ablation modalities can be employed when reduction of tumor burden is required as an adjunct to radiotherapy or when fixation is necessary to avert impending pathologic fracture [Citation8,Citation9]. While the most well-established indication for the ablation of bone metastases is palliation of pain from a solitary focal lesion [Citation10], ablation of oligometastatic disease is becoming more common as a way to postpone or avoid systemic therapies and their potential side effects [Citation10–12]. For this purpose, minimally invasive percutaneous approaches, such as radiofrequency ablation, microwave ablation, cryoablation, and irreversible electroporation (IRE), have been increasingly used clinically because they cause less morbidity than open surgical methods [Citation8,Citation13,Citation14]. While deemed safe and often efficacious, many ablation methods employ thermal properties, which may result in thermal injuries to the spinal cord and nerves [Citation15]. Additionally, ablation modalities such as microwave ablation and radiofrequency ablation are susceptible to the heat sink or cooling effect of blood flow when used in areas near blood vessels, as is often the case in bones [Citation16].
Among these percutaneous approaches, IRE is unique because it is considered primarily nonthermal and does not depend on temperature to be effective, thus avoiding heat-related limitations [Citation17]. IRE relies on high-voltage pulses of electrical current that cause irreversible damage to the cell membrane [Citation18,Citation19] by creating nanopores that lead to the loss of homeostasis and eventual cell death while preserving noncellular stroma [Citation10,Citation20,Citation21]. This approach also reduces the risk of permanent damage to proximal vital tissues, such as nerves and vascular bundles, [Citation13,Citation18,Citation22–24], which is important in the management of bone metastases due to their proximity to these structures. Some hyperthermia has been observed with IRE use as thermal ablative temperatures (≥ 50 °C) and histological signs of thermal damage within the IRE ablative zone have been reported [Citation19,Citation25,Citation26], although simulations suggest that this effect is largely mild hyperthermia (40°C ≤ T < 50 °C) with very limited thermal ablation (5%) [Citation27]. Yet, optimization of the ablative zone to minimize exposure of vital structures to the IRE field is an important preparatory step in IRE treatment [Citation28]. However, the same adjustments to the IRE field that protects vital structures may also lead to incomplete elimination of tumor cells. As a result, recurrence remains a significant limitation of currently available ablation therapies [Citation29–31] with the rate of local tumor control ranging widely from 36 to 97% [Citation11,Citation32–35].
Prostate cancer bone metastasis is unique in that the lesions tend to be bone forming rather than bone lysing [Citation36]. Induction of endothelial-to-osteoblast transition by tumor-secreted bone morphogenetic protein 4 (BMP4) was shown to be one of the mechanisms underlying development of bone-forming metastases in prostate cancer [Citation37]. Prostate cancer-stimulated bone formation has been shown to release factors from bone, termed osteocrines, that activate survival pathways in tumor cells and contribute to resistance to chemotherapies or targeted therapies [Citation37,Citation38]. Radiopharmaceuticals, such as strontium-89, have been used to control prostate tumor-induced osteoblasts, but patients treated with radiopharmaceutics have limited survival benefit [Citation39]. Radium 223 dichloride (Ra-223, Xofigo) is the first alpha-emitting radionuclide approved by the US Food and Drug Administration for men with symptomatic bone metastases and castration-resistant prostate cancer. Ra-223 has been shown to increase the median survival of patients with prostate cancer by 3.6 months and reduce bone pain [Citation40]. Ra-223 acts as a calcium analog and is incorporated into the sites of active mineralization [Citation41]. As a result, Ra-223 concentrates in areas of high bone-forming activity [Citation42]. This results in a much more energetic and localized area of effect [Citation43]. Unlike β-emitters, Ra-223 has a short particle track length [Citation44] and exerts potent cell killing activity through non-reparable double-strand DNA breaks [Citation45]. Its short range causes fewer complications, such as bone marrow suppression or destruction, of adjacent normal tissue than are observed with β-emitters.
Combining ablation techniques with other anticancer treatments may improve the effectiveness of treatment. We hypothesized that Ra-223 incorporated into the calcifying bone matrix within the tumor will eradicate cells in the area adjacent to bone, resulting in enhanced antitumor activity following IRE. In this study, we explored the potential antitumor benefit of combining IRE with Ra-223 for treating bone-forming prostate cancer tumors.
Materials AND methods
Preparation of radium-223
Radioactivity of Ra-223 solution was quantified using Capintec CRC-25R (Florham Park, NJ) dose calibrator upon receipt and immediately before use. Solutions were stored at room temperature and diluted with culture medium or sterile saline to desired concentration immediately prior to use.
Cell lines
To generate C4-2B cells expressing BMP4 and a luciferase reporter (LT), cDNAs encoding human BMP4 were inserted into the bicistronic retroviral vector pBMN-I-Neo was used to generate retroviruses containing BMP4. The resulting retroviruses were used to infect C4-2B–LT cells. The cells were then selected by G418 (Corning 30-234-CR). BMP4-expressing Myc-CaP and TRAMP-C2 cells were produced by transfection with a bicistronic retroviral vector containing the mouse BMP4 cDNA and floxed-green fluorescent protein (GFP) through internal ribosome entry sites (IRES). Cells were selected by fluorescence-activated cell sorting through GFP. GFP was then removed using an adenoviral vector containing cre recombinase.
C4-2B–BMP4 cells were maintained in RPMI-1640 medium (Corning) supplemented with 10% fetal bovine serum (Sigma Life Science) and 1% penicillin-streptomycin solution (Corning). Myc-CaP–BMP4 cells were maintained in Dulbeco’s Modified Eagle’s Medium (DMEM) (ATCC 30-2002) that was supplemented with 10% fetal bovine serum (Sigma Life Science), 1% penicillin-streptomycin solution (Corning), and 0.02% G418 sulfate (Corning 30-234-CR). TRAMP-C2– BMP4 cells were maintained in DMEM (ATCC 30-2002) that was supplemented with 0.005 mg/ml bovine insulin (Sigma Life Science), 10 nM trans-dehydroandrosterone (Sigma Life Science), 5% fetal bovine serum (Sigma Life Science), 5% NuSerumTM IV Culture Supplement (Corning 355104), and 1% penicillin-streptomycin solution (Corning 30-002-CI). Cultures were grown in 75 cm3 or 175 cm3 Nunc EasyYFlask cell culture flasks (Thermo Fisher Scientific) and kept at 37 °C inside a 5% CO2 incubator (Heracell, Thermo Fisher Scientific). Cultures were maintained at 60 to 80% confluence with media replaced two to three times per week.
In vitro study
The three prostate cancer cell lines were evaluated for response to different doses of Ra-223 radioactivity. Cells were washed with phosphate-buffered saline (PBS) (Corning) and digested by addition of 2 ml of 0.01% w/v trypsin with 0.53 mM EDTA solution (Corning) for 2 to 5 min prior to addition of 4X complete culture medium. Cell suspension was dispensed into 96-well plates (Corning) at 5000 cells/well. Ra-223 was prepared in complete medium and added to the cells with radioactivity ranging from 25 to 3600 Bq. Cell viability was measured for 7 days using alamarBlue HS cell viability assay (Thermo Fisher Scientific). Briefly, alamarBlue HS reagent was added to complete culture medium to make a 10% solution. Media in wells were replaced with alamarBlue-medium preparation and allowed to incubate at 37 °C inside a 5% CO2 incubator (Heracell, Thermo Fisher Scientific). After 1 h of incubation, fluorescence measurements were taken with excitation at 540 nm and emission at 590 nm using a Cytation 5 cell imaging multi-mode reader (BioTek Instruments, Vermont).
To determine the effect of electroporation on the cell viability of the three prostate cancer cell lines, cell cultures were resuspended and prepared at a density of 1000 cells/µL. Then, 400/800 µL of cell suspension was transferred to electroporation cuvettes (BTX, Harvard Apparatus). Cuvettes were positioned in a safety stand (BTX, Harvard Apparatus) and individually subjected to different field strengths from 250 to 2500 V/cm delivered across 99 pulses at 100-µs intervals and 10-Hz frequency using an ECM 830 Electro Square Porator (BTX, MA). Following electroporation, the cell suspensions in cuvettes were dispensed into 96-well plates (Corning). Cell viability after 24, 48, and 72 h was determined using alamarBlue HS cell viability assay.
To explore the combinatorial effect of IRE and Ra-223, cells in suspension were subjected to a reversible field strength of 500 V/cm using the same parameters as described previously and then plated on 96-well plates at 5000 cells/well. Cell suspensions that were not electroporated were dispensed similarly. Ra-223 was prepared and dispensed to treatment groups at 1,600 Bq/well. Cell viability at 24, 72, and 120 h was determined using an alamarBlue HS cell viability assay.
Tumor model
All animal studies were performed in compliance with relevant ethical regulations for animal testing and research and were approved by the Institutional Animal Care and Use Committee of The University of Texas MD Anderson Cancer Center.
The bone-forming subcutaneous tumor model was developed using 6- to 8-week-old athymic nude mice (Experimental Radiation Oncology, MD Anderson Cancer Center, Houston, TX). C4-2B–BMP4 cells were washed and resuspended in PBS at a density of 10,000 cells/µL. Cell suspension was mixed with Matrigel (Corning, NY) in a 1:1 preparation and kept cold on ice. Sedated mice were subcutaneously inoculated in the right lower flank with 200 µL of cell suspension containing 1 million cells. Mouse body weight and tumor size were measured weekly. Calcification in tumors was monitored using X-ray (Faxitron, IL) and micro-computed tomography imaging (GE Healthcare, IL). Mice with tumors at least 5 mm in diameter were assigned to one of four treatment groups: control, Ra-223, IRE, and IRE plus Ra-223. Prior to treatment, mouse body weight and tumor size were documented. Tumor size was recorded by caliper and in vivo luciferase bioluminescence was documented using an IVIS 200 system (PerkinElmer, MA).
Radioactivity of the Ra-223 solution was determined, and the solution was diluted with sterile saline to administer 7.4 kBq [∼300 kBq/kg] per mouse via intravenous lateral tail vein injection. Mice selected for IRE were sedated under 2% isoflurane (Isothesia, Henry Schein, NY). The tumor site was sterilized with a chlorhexidine swab and allowed to dry. A 5-mm 2-needle array electrode (BTX, MA) was positioned along the long axis of the tumor and inserted percutaneously. IRE was administered across 99 pulses at 1-s intervals and 1-Hz frequency using an ECM 830 Square Wave Electroporation System. A 10-s rest interval was inserted after every 10 pulses. Mice were given 0.1 mg/kg buprenorphine (Buprenex) intramuscularly for analgesia before waking from sedation. For mice in the combination therapy group, IRE was performed within an hour following administration of Ra-223. Mouse weight, tumor size, and in vivo tumor bioluminescence were measured 1 and 7 days after treatment then weekly for 4 weeks (Supplementary Figure 1).
To observe tumor-free survival, mice treated with IRE and IRE plus Ra-223 were grouped based on the completeness of ablation. This was based on the presence (incomplete) or absence (complete) of bioluminescence 1 day after treatment. Reemergence of bioluminescence in completely ablated tumors was considered tumor recurrence.
Histology
Half of the mice in each group were euthanized 1- and 4-weeks following treatment through CO2 asphyxiation followed by cervical dislocation. Tumors were excised and fixed in 10% formaldehyde before being embedded in paraffin. Tissue slides were prepared from the paraffin-embedded specimens and stained with Von Kossa, hematoxylin-eosin (H&E), and Ki-67 to evaluate calcification, necrosis, and cell proliferation, respectively. Slides were imaged digitally via Aperio Digital Pathology Slide Scanner (Leica Biosystems, IL). Using grid analysis, we counted the number of stained regions of interest and the number of regions with tumor presence. We divided the number of regions of interest by the total tumor coverage to determine the percentage of the tumors that were calcified (% calcification), were necrotic (% necrosis), or had cell proliferation (% cell proliferation).
Statistical analysis
Statistical analysis was performed with GraphPad Prism software (version 4.0). Unless otherwise stated, measurements are expressed as mean ± SD with group differences evaluated using the Student’s t-test or one-way analysis of variance (ANOVA). Groups with a P value less than 0.05 were considered significant.
Results
Effect of Ra-233 on prostate cancer cell lines
When the BMP4-expressing prostate cancer cell lines C4-2B–BMP4, TRAMP-C2–BMP4, and Myc-CaP–BMP4 were treated with increasing doses of Ra-223 in vitro, a dose-dependent reduction of cell proliferation was observed in all three cell lines. A Ra-223 dose of ≥ 800 Bq/mL resulted in significant reduction in cell proliferation at day 3 for all three cell lines with effects persisting up to 5 days following treatment ().
Figure 1. Effect of different Ra-223 concentrations (0 to 6400 Bq/mL) on prostate cancer cell proliferation. Data points represent mean, and error bars represent standard deviation. All treatment groups were compared with the control group using a linear model and two-sided statistical tests. In all cell lines, 5-day exposure to Ra-223 produced dose-dependent reduction in cell proliferation. A Ra-223 dose of ≥ 800 Bq/mL resulted in significant reduction in cell proliferation at day 3 for all three cell lines.
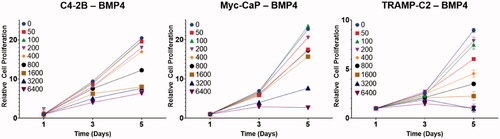
Effect of electroporation on prostate cancer cells
When prostate cancer cells were exposed to electroporation, we observed a decrease in cell viability with increasing field strength (). A significant reduction in cell viability was first observed at ≥ 1000 V/cm after 48 h. For C4-2B–BMP4 cells, viability following exposure to 1000 V/cm was reduced to 59.98 ± 13.92% and then decreased further to below 20% at 1250, 1500, and 2000 V/cm. Complete inhibition was achieved at 2500 V/cm, at which point virtually no cells were found to be viable. Similar trends were observed for TRAMP-C2–BMP4 and Myc-CaP–BMP4 cells.
Figure 2. Response of prostate cancer cell lines to in vitro electroporation. Cell viability was not significantly affected at field strengths up to 500 V/cm. Effect on viability was detected at ≥750 V/cm. Viable cells were nearly undetectable for TRAMP-C2–BMP4 and Myc-CaP–BMP4 at 2000 V/cm and for C4-2B–BMP4 at 2500 V/cm.
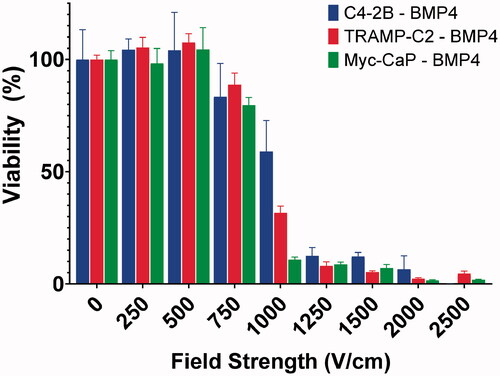
Effect of reversible electroporation and Ra-223 combination on prostate cancer cells
To examine the effect of electroporation and Ra-223 combination therapy on prostate cancer cell lines, we used a field strength of 500 V/cm, which was shown to have minimal to no cytotoxicity (). We found that the cell viability was significantly lower following treatment with both agents than it was following reversible electroporation or Ra-223 treatment alone (). C4-2B–BMP4 cells were the most susceptible to combination therapy such that relative viability was 10.63 ± 2.33% with combination therapy versus 43.03 ± 5.29% with Ra-233 alone (p < 0.001) and 88.95 ± 11.15% with reversible electroporation alone (p < 0.001). TRAMP-C2–BMP4 cells had a similarly significant decrease in viability following combination treatment: relative viability was 44.23 ± 5.25% with combination therapy versus 75.33 ± 9.85% with Ra-223 alone (p < 0.001) and 87.54 ± 8.14% with reversible electroporation alone (p < 0.001). For Myc-CaP–BMP4 cells, the overall reduction in viability was low, with relative viability remaining over 50% with combination treatment, although combination treatment did show improvement over single-agent treatment (p values < 0.05).
Figure 3. Combinatorial effect of Ra-223 and electroporation on the viability of prostate cancer cell lines. Ra-223 (1600 Bq/mL) in combination with reversible electroporation (RE; 500 V/cm) produced significantly greater reduction in cell viability in three BMP4-expressing prostate cancer cell lines than electroporation or Ra-223 alone.
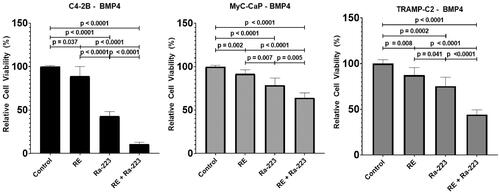
In vivo efficacy of IRE with Ra-223
To evaluate the efficacy of IRE plus Ra-223 in an in vivo model, we first characterized our tumor model by inoculating BMP4 and luciferase-expressing C4-2B cells (C4-2B–BMP4) on the right flank of athymic nude mice. Flatbed X-ray imaging of the tumor site demonstrated enhanced opacity, suggesting mineralization. Calcium deposition was confirmed by Von Kossa staining of tumor sections, suggesting that C4-2b–BMP4 cells generated bone-forming tumors in vivo (Supplementary Figure 2).
Tumor induction was successful in 83% of mice. Tumor-bearing mice were assigned to three treatment groups when their tumor diameters reached ≥ 5 mm: Ra-223 only, IRE only, and IRE plus Ra-223. An untreated control group was also maintained. Tumor size and in vivo bioluminescence from luciferase were used to monitor tumor growth over 4 weeks (). One week following treatment, tumors treated with Ra-223 were significantly smaller from baseline (relative size, 116.27 ± 44.96%) than tumors in the untreated control group (relative size, 170.28 ± 34.22%; p = 0.004, ). However, at 4 weeks, tumors in the Ra-223 group and control group had similar relative tumor sizes: 299.98 ± 218.81% for the Ra-223-treated mice and 206.10 ± 127.20% for the control mice (p = 0.395). Similarly, average bioluminescence () did not differ significantly at 1 or 4 weeks.
Figure 4. In vivo combinatorial effect of Ra-223 and IRE. Tumor growth was measured over the course of 28 days in nude mice bearing C4-2B-BMP4 xenografts. Tumor size was measured using bioluminescence and direct measurement by caliper. (A) Photographs overlaid with bioluminescence imaging of subcutaneously inoculated C4-2B–BMP4 cells. (B) Tumor size measured by caliper. (C) Measurement of total flux in tumor site indicating significant decline of bioluminescence signals in IRE and IRE plus Ra-223 tumors compared to tumors treated with Ra-223 or control.
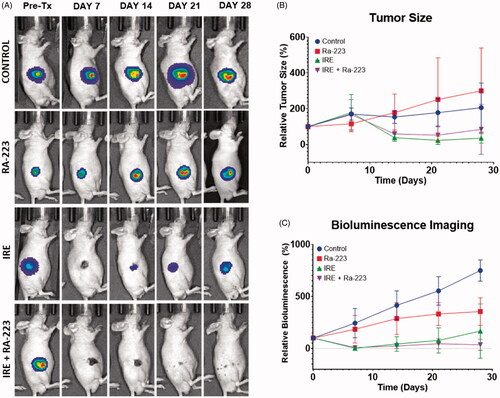
One week following treatments, tumor size in the IRE group (relative size, 178.73 ± 96.34%) and combination treatment group (relative size, 167.49 ± 80.58%) were not significantly different from the control group (relative size, 170.29 ± 32.6%) (). At 4 weeks, the mean relative tumor sizes in the IRE-treated and combination groups were 35.43 ± 28.09% and 84.87 ± 139.31%, respectively. Of these, only IRE-treated tumors were significantly smaller than the control tumors (p = 0.013) or Ra-223-treated (p = 0.023) tumors. Tumor size did not differ significantly between the IRE-treated and combination treatment groups (p = 0.902).
Contrary to direct observation of size by caliper, bioluminescence can detect the presence of cancer cells independent of structural changes due to ablation. Quantification of bioluminescence signals () were significantly lower for IRE and combination treatment groups compared to the control and Ra-223 groups. The addition of Ra-223 to IRE did not result in a significant difference in mean bioluminescence compared to IRE alone throughout four weeks of observation. We further investigated the difference in tumor recurrence between IRE and combination treatment groups among mice whose tumors seemed to be completely ablated as indicated by the absence of bioluminescence 1 day after IRE treatment (). We documented the reemergence of bioluminescence signal to indicate tumor recurrence and calculated the percentage (%) tumor-free survival. Five of the 10 mice in each group had apparently complete ablation, and the remaining 5 had incomplete ablation (). Among the 5 mice with apparently complete ablation (no bioluminescence at 1 day after IRE treatment), tumor recurred in 2 out of 5 (40%) IRE-treated mice, and IRE plus Ra-223-treated mice remained tumor-free (). Bioluminescence for incompletely ablated tumors was persistent throughout 28 days in both IRE and combination-treated groups.
Figure 5. Tumor-free survival in IRE-treated mice. (A) Bioluminescence imaging of all mice (n = 5/group) with apparently complete ablation with IRE 1 day post-treatment. Four weeks after treatment, 2 out of 5 IRE-treated mice showed reemergence of signal while no signal reemergence was observed in mice treated with a combination of IRE and Ra-223. (B) All mice treated with IRE plusRa-223 were tumor-free 28 days after treatment, compared to only 60% in IRE-treated mice.
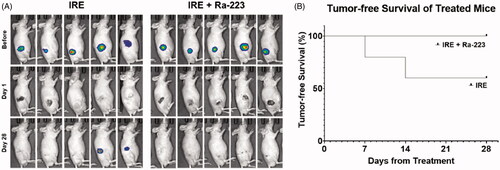
Histologic evaluation of tumors
In the untreated C4-2B–BMP4 tumors, calcification was observed more frequently in areas with loose cellular configuration, intercellular spaces, and areas toward the periphery of the tumor (Supplementary Figure 3), consistent with the mechanism of BMP4-induced bone formation through recruitment of endothelial cells that undergo endothelial-to-osteoblast transition in the tumors [Citation37, Citation46]. One week following treatment, the untreated tumors had a mean % calcification of 8.0 ± 6.67%, which was not significantly different from the mean % calcification of the tumors treated with Ra-223 (6.67 ± 2.58%; p = 0.963), IRE (12.0 ± 6.71%; p = 0.534), or IRE plus Ra-223 (11 ± 4.1%; p = 0.737) (). Four weeks after treatment, the mean % calcification in the treatment groups were lower than those in the untreated controls (16.43 ± 12.82% versus 10.0 ± 7.07% in the Ra-223 group [p = 0.554], 2.5 ± 4.18% in the IRE group [p = 0.030], and 3.33 ± 4.08% in the IRE plus Ra-223 group [p = 0.044]. Representative Von Kossa staining for each of the groups is shown in Supplementary Figure 4.
Figure 6. Quantification of (A) calcification and (B) necrosis in C4-2B-BMP4 tumors at 1 and 4 weeks after treatment. Percentage calcification and necrosis from the control (no treatment), Ra-223, IRE, and Ra-223 plus IRE groups were calculated from Von Kossa- and H&E-stained tumor sections, respectively.
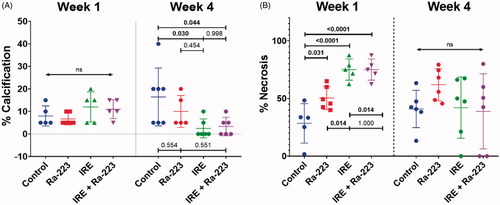
To evaluate the effect of the treatments on cell killing, the extent of tumor necrosis was measured. Representative H&E-stained slides are shown in Supplementary Figure 5. The mean % necrosis for untreated controls (), which was associated with tumor volume and time since inoculation (6 weeks), was 28.48 ± 17.11%. This was significantly higher in all treatment groups: Ra-223, 50.42 ± 10.0% (p = 0.031); IRE, 75.08 ± 9.19% (p < 0.001); and IRE plus Ra-223, 75.08 ± 9.19% (p < 0.001). Comparing treatment groups, significantly higher % necrosis was observed with IRE alone (p = 0.014) and IRE plus Ra-223 (p = 0.014) compared to Ra-223 alone. However, no significant differences were observed between IRE alone and IRE plus Ra-223 (p > 0.999).
In Ra-223-treated tumors, necrosis occurred with greater frequency in areas of calcification observed by Von Kossa staining (). Tumors treated with IRE had more areas of necrosis toward the center of the tumor and fewer areas of necrosis toward the periphery. The addition of Ra-223 to IRE enhanced necrosis in the peripheral region proximal to areas of calcification.
Figure 7. Patterns of necrosis following treatment showing Ra-223 enhances necrosis proximal to regions of calcification. Histology of C4-2B–BMP4 tumors (T) 1 week after treatment shows higher necrosis (N) in regions immediately proximal to sites of calcification (C) when treated with Ra-223. Necrosis in IRE-treated tumors shows more necrosis toward the center of tumor with more viable cells toward the tumor boundary (demarcated by dashed red line). Similar to Ra-223 alone, IRE plus Ra-223 shows enhancement of necrosis adjacent to sites of calcification.
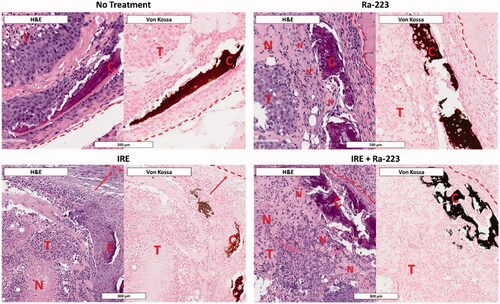
At week 4, the extent of necrosis no longer differed significantly among the different treatment groups (p = 0.317) and could not be meaningfully compared. The % necrosis recorded for the different groups were as follows: 62.03 ± 13.98% for the Ra-223 group; 42.08 ± 26.56% for the IRE group; 38.85 ± 32.59%; for IRE plus Ra-223 and 40.97 ± 16.22% for the untreated control.
To better evaluate the efficacy of the treatments, cell proliferation was determined using Ki-67 staining (). The untreated control group exhibited high percentages of Ki-67-positive tumor areas at 1 week (46.54 ± 14.82%) and 4 weeks (39.81 ± 10.56%) after treatment (). Treatment with Ra-223 did not result in a significant difference in Ki-67 positivity from the control at 1 week (36.85 ± 4.92%, p = 0.230) or 4 weeks (33.36 ± 14.93%, p = 0.437). Treatment with IRE and IRE plus Ra-233 resulted in a significant decrease in Ki-67 positivity at 1 week (16.74 ± 5.56% for IRE and 15.78 ± 6.95% for IRE plus Ra-223; p < 0.001). In contrast, % proliferative cells in tumor sections at 4 weeks had greater variability compared to bioluminescence data. These variances resulted in means that did not differ significantly from untreated controls (24.36 ± 24.21%; p = 0.480 for IRE and 12.84 ± 6.20%; p = 0.036 for IRE plus Ra-223). IRE-treated groups were disaggregated based on bioluminescence at 1 day posttreatment, and tumors were classified as having either complete or incomplete ablation (). Results show that even though there was no difference in % proliferative cells among groups at week 1, differences were observed at week 4. In the complete ablation IRE group, % proliferative cells was slightly higher (12.22 ± 6.22%) compared to the IRE plus Ra-223 group (6.81 ± 1.68%), although the difference was not statistically significant (p = 0.572). This may be caused by the reduction in the mean that was masked by the high variability associated with tumor recurrence in the IRE-only group. The incomplete ablation group treated with IRE only had a more pronounced % proliferative cells (53.58 ± 16.0%) compared to the incomplete ablation group treated with IRE plus Ra-223 (20.12 ± 1.63%, p = 0.004).
Figure 8. Cell proliferation in C4-2B–BMP4 tumors at 1 week and 4 weeks after treatment. (A) Representative Ki67-stained tumor sections from the control, Ra-223, IRE, and Ra-223 plus IRE groups. The % cell proliferation was calculated as the percentage of tumor area with Ki-67 staining. (B) Comparison of % cell proliferation by Ki-67 staining at 1 week using a one-way ANOVA followed by Holm-Sidak post-hoc analysis were as follows: control versus Ra-223, p = 0.300; control versus IRE, p < 0.001; control versus IRE plus Ra-223, p < 0.001; Ra-223 versus IRE, p = 0.008; Ra-223 versus IRE plus Ra-223, p = 0.005; and IRE versus IRE plus Ra-223, p = 0.998. Comparison of % cell proliferation at 4 weeks were as follows: control versus Ra-223, p = 0.437; control versus IRE, p = 0.480; control versus IRE plus Ra-223, p = 0.036; Ra-223 versus IRE, p = 0.999; Ra-223 versus IRE plus Ra-223, p = 0.611; and IRE vs. IRE plus Ra-223, p = 0.485. (C) Comparison of % cell proliferation between tumors treated with IRE and IRE plus Ra-223 after segregating based on completeness of ablation. Statistically significant increase in % proliferative cells is noted for incompletely ablated tumors treated with IRE only (53.58 ± 16.0%) compared to IRE plus Ra-223 (20.12% ± 1.63%; p = 0.004).
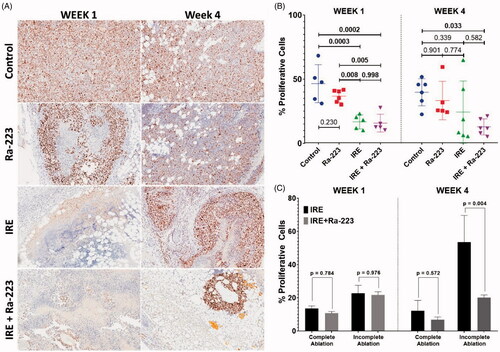
Discussion
In this study, we evaluated the combined effect of IRE and Ra-223, a therapeutic agent with favorable specificity for metastatic bone lesions, on a model using bone-forming prostate cancer tumors. Our findings suggest that the addition of Ra-223 may enhance the antitumor activity of IRE by killing tumor cells that are adjacent to bone, therefore decreasing tumor cell proliferation and recurrence.
Use of percutaneous ablation of bone metastasis is increasing because it is less invasive than surgical resection. Percutaneous bone metastasis ablation is presently accomplished through modalities such as radiofrequency ablation, cryoablation, and microwave ablation [Citation13]. These have clinically demonstrated therapeutic applications while minimizing the risks associated with open surgery [Citation8]. However, the use of these locally destructive methods is contraindicated in patients with tumors that are adjacent to major blood vessels or nerves, which is a common occurrence in bone metastasis. For both radiofrequency ablation and microwave ablation, heat-associated injury is a significant concern because it can cause permanent damage to neurovascular tissue and has associated postoperative inflammation and pain [Citation14]. Compared to radiofrequency ablation, cryoablation, which uses extreme cold temperatures to destroy tumor tissue through crystal formation and osmotic shock [Citation13], is preferred because it can better deliver energy through sclerotic bone tissue [Citation47,Citation48]. While cryoablation reduces the risk of heat-associated tissue damage by limiting tissue-killing effects to a visualized field, permanent damage is still a possibility due to the proximity of nearby critical tissue [Citation47].
IRE is mainly a non-thermal modality that allows precise control of ablation while evading the destruction of non-pathologic tissue. IRE uses high-voltage pulses of electrical current to cause irreversible damage to the cell membrane [Citation19] by creating pores leading to the loss of homeostasis and eventual cell death while preserving noncellular stroma [Citation10,Citation20,Citation21]. Compared to thermal ablative modalities, IRE is not susceptible to heat sink from nearby vessels, has a shorter treatment time, and can be more precisely controlled [Citation13]. The generated ablative zone in IRE has well-defined margins that demarcate ablated and non-ablated tissue, allowing for precise control [Citation49,Citation50]. This ablative zone resolves rapidly with little scarring or damage to the underlying noncellular matrix including preservation of adjacent vessels, ducts, and nerves [Citation49–51]. More recently, studies have shown that mild hyperthermia results from IRE [Citation19,Citation27,Citation52–54]. However, hyperthermia is a desirable treatment characteristic and has been utilized to enhance the efficacy of IRE when combined with chemotherapeutics to prevent recurrence [Citation55–59].
Tumor recurrence is possible following incomplete ablation in IRE [Citation60–62]. In an analysis of 150 patients who received IRE for soft tissue tumors, 22 to 24% had disease recurrence [Citation61]. IRE is expected within the ablative zone defined by the placement of probes. Beyond this area, the effective field strength becomes reversible and does not lead to cell death [Citation28, Citation63]. Because of this, larger tumors are expected to have a higher rate of recurrence [Citation63]. Variations within the ablative zone may also occur due to heterogeneity in the tumor, which is often the case in tumors adjacent bone. This may affect the impedance distribution that in turn affects the distribution of the electric field [Citation64]. Cancer cells that fall outside the ablative zone will survive ablation, leading to recurrence [Citation57]. Thus, to improve tumor clearance, IRE has been combined with anticancer therapies, including drugs [Citation65–70], immunotherapeutic agents [Citation59], and nanoparticles [Citation56–58].
In this study, we combined IRE with the radiopharmaceutical, Ra-223, to enhance treatment efficacy by killing tumor cells adjacent to bone, thus preventing recurrence. Ra-223 is a radionuclide that has beneficial effects against castration-resistant prostate cancer bone metastasis [Citation71,Citation72]. The bone-seeking activity of Ra-223 is a result of it being a calcium analog resulting in its incorporation in the bone matrix during osteogenesis [Citation41]. Unlike other bone metastases that are osteolytic, prostate cancer-induced bone lesions are osteogenic with increased osteoblastic activity [Citation46]. Previous studies have shown that the parts of the bone with active remodeling and that are vascular are the main site of Ra-223 accumulation. In mice with prostate cancer growing in bone, Ra-223 accumulated on the bone surface next to the malignant site [Citation73]. A murine xenograft study on metastatic prostate cancer, has shown that Ra-223 exhibits a dual targeting mode-of-action that induces tumor cell death and suppresses tumor-induced pathologic bone formation in tumor microenvironment of osseous castration-resistant prostate cancer growth in mice [Citation74]. We believe that with IRE effectively debulking most of the tumor, the addition of Ra-223 will result in further clearance of straggling tumor cells near the calcified tissue.
Using three prostate cancer cell lines, we observed that Ra-223 and electroporation have independent, direct cell-killing effects on prostate cancer cells in vitro, with these effects directly related to increasing radioactivity(in the case of Ra-223) and increasing electrical field strength (in the case of electroporation). Combining electroporation at reversible field strength of ≤500 V/cm enhanced the cell killing effects of Ra-223. This enhancement in cell-killing activity may be directly related to cell proliferation. In a previous study, it was observed that electroporation with low field strength may result in increased cell viability compared to the viability of untreated cells [Citation56]. Such increase in viability suggests that there is increased cell proliferative activity. In the presence of Ra-223, increased cell division may make cells more susceptible to the non-reparable double-strand DNA breaks by alpha particles, leading to cell death [Citation41]. However, our in vitro model does not completely recapitulate the relationship of Ra-223 and metastatic bone tumors, in which Ra-223 accumulates in bone in areas close to tumor cells [Citation73]. In our model, prostate cancer cells are bathed in culture media containing Ra-223, making exposure of the cells uniform rather than dependent on proximity to the radioactive source [Citation75]. In this regard, electropermeabilization may have contributed to enhancing the cell-killing activity of Ra-223 [Citation76]. This strategy of combining electroporation with chemotherapeutics has been employed to enhance their cellular uptake to increase their potency [Citation20,Citation56–58].
From the in vitro results, we determined that C4-2B–BMP4 was the most sensitive to the synergistic effects of electroporation and Ra-223. Therefore, in the succeeding animal experiments, we induced bone-forming C4-2B–BMP4 xenografts and assessed treatment effects at 1 and 4 weeks following intervention. C4-2B cells have been modified to have enhanced expression of BMP4, which facilitates the conversion of endothelial cells to osteoblast-like cells, leading to ectopic bone formation under subcutaneous implantation [Citation37]. Although the xenograft has limitations in terms of irregular calcification patterns, with frequent occurrence toward the periphery and with occasional patches within the tumor, the subcutaneous model allowed us to grow calcified tumors larger than 5 mm, which was the minimum distance required by IRE probes used in this study. Tumors larger than 5 mm would not have been possible in an orthotopic model without breaking the bone. The superficial nature of subcutaneous xenografts also allowed easier and more reliable technical manipulation of the IRE probes to maximize tumor coverage without requiring the use of radiographic imaging equipment.
At week 1 post-treatment, there were no apparent differences in tumor size among the treatment groups. The observed transient increase in apparent tumor size at 1 week could be attributed to inflammatory response typical of IRE treatment [Citation77]. In addition, we observed that measurements of tumors by caliper were also affected by structural changes in tumor site (Supplementary Figure 6), including the formation of scar tissue in IRE-treated tumors that was not easily distinguishable from tumors [Citation78–80]. Therefore, we relied on bioluminescence imaging, which is indicative of the cancer cells present in the region and excludes the effect of inflammation. Our results show that Ra-223 treatment at 1 week showed less bioluminescence signal compared to control and histological evaluation showed necrosis in the tumors adjacent to where calcification was observed on Von Kossa staining (), confirming the short-distance, cytotoxic effect of Ra-223 [Citation73]. However, a single-dose (300 kBq/kg) of Ra-223 did not result in a significant decrease in proliferative cells on histology despite increase in necrosis following treatment. It is possible that the limited calcification in the tumors also limited the efficacy of Ra-223. In contrast, IRE resulted in significant tumor clearance based on bioluminescence imaging. Similar to what was observed in previous studies [Citation56,Citation58], histologic evaluation of these tumors revealed that necrosis was concentrated toward the center of the tumor where the electroporation probes were positioned and dispersed outwards, reflective of the depreciating field strength away from the central ablative zone. Similarly, proliferative cells were observed mainly toward the periphery or were densely clustered outside the ablative zone. When IRE and Ra-223 were combined, there was low cell proliferation and a high level of necrosis, but these findings were not significantly different when compared to those of IRE alone. The histological pattern of necrosis was more uniform across the tumor area with similar central and peripheral distribution, reflecting the combined area of effect of the two modalities.
Four weeks after treatment or 10 weeks after tumor induction, single-dose administration of Ra-223 did not result in significant tumor elimination in terms of tumor size, necrosis, and cell proliferation compared to controls. However, the bioluminescence signal was reduced significantly, suggesting alternative causation for the inconsistent observations. Necrosis is a potential confounder and may be a consequence of increased tumor volumes, which were relatively large for the controls and Ra-223 group by this time point. As tumor volumes increase, the central areas become increasingly hypoxic [Citation81] and necrotic [Citation82,Citation83]. Our study was also limited by a single-dose administration of Ra-223 compared to a similar study of Suominen et al. in which they found that Ra-223 effectively reduces tumor area in Ra-223-treated mice bearing intratibial prostate tumors following two doses 4 weeks apart [Citation74]. In contrast, treatment with IRE alone or combination therapy was observed to result in dramatic reduction in tumor size and bioluminescent signal at 4 weeks. However, there was no apparent difference in tumor size and bioluminescence signal between IRE and combination therapy. Tumor size is not a predictive measure of tumor reduction since temporal remodeling at former tumor sites is prone to inflammation and scar formation, which may confound caliper measurements as previously discussed [Citation84–86]. On the other hand, bioluminescence represents measurable tumor cells, but no statistically significant difference was observed between the IRE and combination therapy groups due to the wide variability in signals. We attribute this variability to an initial inability to completely ablate large or irregularly shaped tumors during IRE resulting in incomplete elimination of tumor cells allowing for tumor persistence and regrowth. Among the mice treated with IRE or combination therapy, half (n = 5) had incomplete ablation, and the other half had complete ablation despite similar initial tumor size prior to treatment. The effect of combined therapy is evident at 4 weeks with Ra-223 enhancing the efficacy of IRE by: (1) reducing proliferative cells in incompletely ablated tumor as shown in Ki-67 staining where the calculated % proliferative cells was double for IRE as compared to the combination group; and (2) reducing tumor recurrence in completely ablated tumors as shown by 40% of the IRE-treated mice in IRE group having tumor recurrence and none of the IRE plus Ra-223-treated mice having reemergence of bioluminescent signal. These results suggest that IRE is enhanced by Ra-223, which may decrease the risk of cancer recurrence in prostate cancer bone lesions.
In conclusion, we documented that bone-forming metastatic prostate cancer cells were responsive to treatment with IRE and Ra-223 in vitro with possible enhancement when used in combination. In vivo evaluation in a subcutaneous murine model showed that IRE with Ra-223 behaved similar to IRE alone but had demonstrable benefits in terms reducing the risks of tumor recurrence. These findings add Ra-223 to potential combinatorial options for IRE. It is notable that single-dose administration of Ra-223 may be suboptimal, and repeat administration may enhance the treatment benefit [Citation74]. Further preclinical studies using orthotopic models that recapitulate the tumor microenvironment in the bone are needed to determine if the combination of IRE with Ra-223 and possibly a third agent [Citation75] is effective for prostate cancer bone metastasis.
Supplemental Material
Download PDF (1.1 MB)Acknowledgements
The authors thank Stephanie Deming, Senior Scientific Editor, and Ashli Nguyen-Villarreal, Associate Scientific Editor, in the Research Medical Library at The University of Texas MD Anderson Cancer Center, for editing this article.
Disclosure statement
No potential conflict of interest was reported by the author(s).
Additional information
Funding
References
- Bubendorf L, Schöpfer A, Wagner U, et al. Metastatic patterns of prostate cancer: an autopsy study of 1,589 patients. Hum Pathol. 2000;31(5):578–583.
- DePuy V, Anstrom KJ, Castel LD, et al. Effects of skeletal morbidities on longitudinal patient-reported outcomes and survival in patients with metastatic prostate cancer. Support Care Cancer. 2007;15(7):869–876.
- Janjan NA, Payne R, Gillis T, et al. Presenting symptoms in patients referred to a multidisciplinary clinic for bone metastases. J Pain Symptom Manage. 1998;16(3):171–178.
- Rustøen T, Moum T, Padilla G, et al. Predictors of quality of life in oncology outpatients with pain from bone metastasis. J Pain Symptom Manage. 2005;30(3):234–242.
- Coleman R, Brown J, Terpos E, et al. Bone markers and their prognostic value in metastatic bone disease: clinical evidence and future directions. Cancer Treat Rev. 2008;34(7):629–639.
- Jehn CF, Diel IJ, Overkamp F, et al. Management of Metastatic Bone Disease Algorithms for Diagnostics and Treatment. Anticancer Res. 2016;36(6):2631–2637.
- Wu JS-Y, Wong R, Johnston M, et al.; Cancer Care Ontario Practice Guidelines Initiative Supportive Care Group. Meta-analysis of dose-fractionation radiotherapy trials for the palliation of painful bone metastases. Int J Radiat Oncol Biol Phys. 2003;55(3):594–605.
- Agarwal MG, Nayak P. Management of skeletal metastases: An orthopaedic surgeon's guide. Indian J Orthop. 2015;49(1):83–100.
- Foster RC, Stavas JM. Bone and soft tissue ablation. Semin Intervent Radiol. 2014;31(2):167–179.
- Kurup AN, Morris JM, Callstrom MR. Ablation of musculoskeletal metastases. AJR Am J Roentgenol. 2017;209(4):713–721.
- Erie AJ, Morris JM, Welch BT, et al. Retrospective review of percutaneous image-guided ablation of oligometastatic prostate cancer: a single-institution experience. J Vasc Interv Radiol. 2017;28(7):987–992.
- McMenomy BP, Kurup AN, Johnson GB, et al. Percutaneous cryoablation of musculoskeletal oligometastatic disease for complete remission. J Vasc Interv Radiol. 2013;24(2):207–213.
- Knavel EM, Brace CL. Tumor ablation: common modalities and general practices. Tech Vasc Interv Radiol. 2013;16(4):192–200.
- Vroomen LGPH, Petre EN, Cornelis FH, et al. Irreversible electroporation and thermal ablation of tumors in the liver, lung, kidney and bone: What are the differences? Diagn Interv Imaging. 2017;98(9):609–617.
- Tomasian A, Jennings JW. Percutaneous minimally invasive thermal ablation of osseous metastases: evidence-based practice guidelines. AJR Am J Roentgenol. 2020;215(2):502–510.
- Pillai K, Akhter J, Chua TC, et al. Heat Sink effect on tumor ablation characteristics as observed in monopolar radiofrequency, bipolar radiofrequency, and microwave, using ex vivo calf liver model. Medicine. 2015;94(9):e580.
- Maor E, Ivorra A, Rubinsky B. Non thermal irreversible electroporation: novel technology for vascular smooth muscle cells ablation. PLoS ONE. 2009;4(3):e4757.
- Davalos RV, Mir IL, and, Rubinsky B. Tissue ablation with irreversible electroporation. Ann Biomed Eng. 2005;33(2):223–231.
- Faroja M, Ahmed M, Appelbaum L, et al. Irreversible electroporation ablation: is all the damage nonthermal? Radiology. 2013;266(2):462–470.
- Melancon MP, Appleton Figueira T, Fuentes DT, et al. Development of an electroporation and nanoparticle-based therapeutic platform for bone metastases. Radiology. 2018;286(1):149–157.
- Tam AL, Abdelsalam ME, Gagea M, et al. Irreversible electroporation of the lumbar vertebrae in a porcine model: is there clinical-pathologic evidence of neural toxicity? Radiology. 2014;272(3):709–719.
- Blazevski A, Scheltema MJ, Amin A, et al. Irreversible electroporation (IRE): a narrative review of the development of IRE from the laboratory to a prostate cancer treatment. BJU Int. 2020;125(3):369–378.
- Edd JF, Horowitz L, Davalos RV, et al. In vivo results of a new focal tissue ablation technique: irreversible electroporation. IEEE Trans Biomed Eng. 2006;53(7):1409–1415.
- Vogel JA, van Veldhuisen E, Agnass P, et al. Time-dependent impact of irreversible electroporation on pancreas, liver, blood vessels and nerves: a systematic review of experimental studies. PLoS One. 2016;11(11):e0166987.
- van den Bos W, Scheffer HJ, Vogel JA, et al. Thermal energy during irreversible electroporation and the influence of different ablation parameters. J Vasc Interv Radiol. 2016;27(3):433–443.
- Wagstaff PGK, de Bruin DM, van den Bos W, et al. Irreversible electroporation of the porcine kidney: Temperature development and distribution. Urol Oncol. 2015;33(4):168.e1–168.e7.
- Agnass P, van Veldhuisen E, van Gemert MJC, et al. Mathematical modeling of the thermal effects of irreversible electroporation for in vitro, in vivo, and clinical use: a systematic review. Int J Hyperthermia. 2020;37(1):486–505.
- Miklavcic D, Davalos RV. Electrochemotherapy (ECT) and irreversible electroporation (IRE) -advanced techniques for treating deep-seated tumors based on electroporation. BioMed Eng Online. 2015;14(Suppl 3):I1.
- Dodd GD, Frank MS, Aribandi M, 3rd, et al. Radiofrequency thermal ablation: computer analysis of the size of the thermal injury created by overlapping ablations. AJR Am J Roentgenol. 2001;177(4):777–782.
- Komorizono Y, Oketani M, Sako K, et al. Risk factors for local recurrence of small hepatocellular carcinoma tumors after a single session, single application of percutaneous radiofrequency ablation. Cancer. 2003;97(5):1253–1262.
- Solbiati L, Ierace T, Tonolini M, et al. Radiofrequency thermal ablation of hepatic metastases. Eur J Ultrasound. 2001;13(2):149–158.
- Bang HJ, Littrup PJ, Currier BP, et al. Percutaneous cryoablation of metastatic lesions from colorectal cancer: efficacy and feasibility with survival and cost-effectiveness observations. ISRN Minim Invasive Surg. 2012;2012:1–10.
- Bang HJ, Littrup PJ, Goodrich DJ, et al. Percutaneous cryoablation of metastatic renal cell carcinoma for local tumor control: feasibility, outcomes, and estimated cost-effectiveness for palliation. J Vasc Interv Radiol. 2012;23(6):770–777.
- Welch BT, Callstrom MR, Morris JM, et al. Feasibility and oncologic control after percutaneous image guided ablation of metastatic renal cell carcinoma. J Urol. 2014;192(2):357–363.
- White ML, Atwell TD, Kurup AN, et al. Recurrence and survival outcomes after percutaneous thermal ablation of oligometastatic melanoma. Mayo Clin Proc. 2016;91(3):288–296.
- Logothetis CJ, Lin SH. Osteoblasts in prostate cancer metastasis to bone. Nat Rev Cancer. 2005;5(1):21–28.
- Lin S-C, Lee Y-C, Yu G, et al. Endothelial-to-Osteoblast Conversion Generates Osteoblastic Metastasis of Prostate Cancer. Dev Cell. 2017;41(5):467–480.e3.
- Lee Y-C, Lin S-C, Yu G, et al. Identification of bone-derived factors conferring de novo therapeutic resistance in metastatic prostate cancer. Cancer Res. 2015;75(22):4949–4959.
- Bilen MA, Johnson MM, Mathew P, et al. Randomized phase 2 study of bone-targeted therapy containing strontium-89 in advanced castrate-sensitive prostate cancer. Cancer. 2015;121(1):69–76.
- Parker C, Nilsson S, Heinrich D, et al. Alpha emitter radium-223 and survival in metastatic prostate cancer. N Engl J Med. 2013;369(3):213–223.
- Deshayes E, Roumiguie M, Thibault C, et al. Radium 223 dichloride for prostate cancer treatment. Drug Des Devel Ther. 2017;11:2643–2651.
- Lassmann M, Nosske D. Dosimetry of 223Ra-chloride: dose to normal organs and tissues. Eur J Nucl Med Mol Imaging. 2013;40(2):207–212.
- Henriksen G, et al. Targeting of osseous sites with alpha-emitting 223Ra: comparison with the beta-emitter 89Sr in mice. Journal of Nuclear Medicine: Official Publication, Society of Nuclear Medicine. 2003;44(2):252–259.
- Bruland ØS, Nilsson S, Fisher DR, et al. High-linear energy transfer irradiation targeted to skeletal metastases by the alpha-emitter 223Ra: adjuvant or alternative to conventional modalities? Clin Cancer Res. 2006;12(20 Pt 2):6250s–6257s.
- Ritter MA, Cleaver JE, Tobias CA. High-LET radiations induce a large proportion of non-rejoining DNA breaks. Nature. 1977;266(5603):653–655.
- Lin S-C, Yu-Lee L-Y, Lin S-H. Osteoblastic factors in prostate cancer bone metastasis. Curr Osteoporos Rep. 2018;16(6):642–647.
- Callstrom MR, Dupuy DE, Solomon SB, et al. Percutaneous image-guided cryoablation of painful metastases involving bone: multicenter trial. Cancer. 2013;119(5):1033–1041.
- Dupuy DE, Hong R, Oliver B, et al. Radiofrequency ablation of spinal tumors: temperature distribution in the spinal canal. AJR Am J Roentgenol. 2000;175(5):1263–1266.
- Lee EW, Chen C, Prieto VE, et al. Advanced hepatic ablation technique for creating complete cell death: irreversible electroporation. Radiology. 2010;255(2):426–433.
- Lee EJ, Lee YH, Jung K-J, et al. Pseudohypertrophy of the calf muscles in a patient with diabetic neuropathy: a case report. J Korean Radiol Soc. 2007;57(3):287–289.
- Li W, Fan Q, Ji Z, et al. The effects of irreversible electroporation (IRE) on nerves. PLOS One. 2011;6(4):e18831.
- Fesmire CC, Petrella RA, Fogle CA, et al. Temperature dependence of high frequency irreversible electroporation evaluated in a 3D tumor model. Ann Biomed Eng. 2020;48(8):2233–2246.
- Edelblute CM, Hornef J, Burcus NI, et al. Controllable moderate heating enhances the therapeutic efficacy of irreversible electroporation for pancreatic cancer. Sci Rep. 2017;7(1):11767.
- Song J, Joshi RP, Schoenbach KH. Synergistic effects of local temperature enhancements on cellular responses in the context of high-intensity, ultrashort electric pulses. Med Biol Eng Comput. 2011;49(6):713–718.
- Fini M, Salamanna F, Parrilli A, et al. Electrochemotherapy is effective in the treatment of rat bone metastases. Clin Exp Metastasis. 2013;30(8):1033–1045.
- Tian L, Qiao Y, Lee P, et al. Antitumor efficacy of liposome-encapsulated NVP-BEZ 235 in combination with irreversible electroporation. Drug Deliv. 2018;25(1):668–678.
- Tian L, Wang L, Qiao Y, et al. Antitumor efficacy of liposome-encapsulated NVP-BEZ235 Combined with Irreversible electroporation for head and neck cancer. Molecules. 2019;24(19):3560.
- Zhao J, Qiao J, Zhou M, et al. Anti-tumor efficacy study using irreversible electroporation and doxorubicin-loaded polymeric micelles. ACS Macro Lett. 2015;4(10):1081–1084.
- Zhao J, Wen X, Tian L, et al. Irreversible electroporation reverses resistance to immune checkpoint blockade in pancreatic cancer. Nat Commun. 2019;10(1):899.
- Langan RC, Goldman DA, D'Angelica MI, et al. Recurrence patterns following irreversible electroporation for hepatic malignancies. J Surg Oncol. 2017;115(6):704–710.
- Philips P, Hays D, Martin RCG. Irreversible electroporation ablation (IRE) of unresectable soft tissue tumors: learning curve evaluation in the first 150 patients treated. PLoS ONE. 2013;8(11):e76260.
- Stillstrom D, et al. Initial experience with irreversible electroporation of liver tumours. Eur J Radiol Open. 2019;6:62–67.
- Cannon R, Ellis S, Hayes D, et al. Safety and early efficacy of irreversible electroporation for hepatic tumors in proximity to vital structures. J Surg Oncol. 2013;107(5):544–549.
- Golberg A, Bruinsma BG, Uygun BE, et al. Tissue heterogeneity in structure and conductivity contribute to cell survival during irreversible electroporation ablation by "electric field sinks. Sci Rep. 2015;5(1):8485.
- Engström PE, et al. Electrically mediated drug delivery for treatment of an adenocarcinoma transplanted into rat liver. Anticancer Res. 2001;21(3b):1817–1822.
- Jaroszeski MJ, Coppola D, Pottinger C, et al. Treatment of hepatocellular carcinoma in a rat model using electrochemotherapy. Eur J Cancer. 2001;37(3):422–430.
- Kitamura A. Bleomycin-mediated electrochemotherapy in mouse NR-S1 carcinoma. Cancer Chemother Pharmacol. 2003;51(4):359–362.
- Mir LM, Orlowski S, Belehradek J, et al. Electrochemotherapy potentiation of antitumour effect of bleomycin by local electric pulses. Eur J Cancer. 1991;27(1):68–72.
- Soden DM, Larkin JO, Collins CG, et al. Successful application of targeted electrochemotherapy using novel flexible electrodes and low dose bleomycin to solid tumours. Cancer Lett. 2006;232(2):300–310.
- Zheng MH. Effects and possible anti-tumor immunity of electrochemotherapy with bleomycin on human colon cancer xenografts in nude mice. WJG. 2005;11(16):2426–2430.
- Logothetis C, Morris MJ, Den R, et al. Current perspectives on bone metastases in castrate-resistant prostate cancer. Cancer Metastasis Rev. 2018;37(1):189–196.
- U.S. Food and Drug Administration, Highlights of Prescribing information for Xofigo (radium Ra 223 dichloride) Injection for intravenous use. 2013.
- Abou DS, Ulmert D, Doucet M, et al. Whole-body and microenvironmental localization of radium-223 in naïve and mouse models of prostate cancer metastasis. JNCIJ. 2016;108(5):djv380.
- Suominen MI, Fagerlund KM, Rissanen JP, et al. Radium-223 inhibits osseous prostate cancer growth by dual targeting of cancer cells and bone microenvironment in mouse models. Clin Cancer Res. 2017;23(15):4335–4346.
- Morris MJ, Corey E, Guise TA, et al. Radium-223 mechanism of action: implications for use in treatment combinations. Nat Rev Urol. 2019;16(12):745–756.
- Rols M-P. Electropermeabilization, a physical method for the delivery of therapeutic molecules into cells. Biochim Biophys Acta. 2006;1758(3):423–428.
- Vogel JA, van Veldhuisen E, Alles LK, et al. Time-dependent impact of irreversible electroporation on pathology and ablation size in the porcine liver: a 24-hour experimental study. Technol Cancer Res Treat. 2019;18:1533033819876899.
- Golberg A, Khan S, Belov V, et al. Skin rejuvenation with non-invasive pulsed electric fields. Sci Rep. 2015;5:10187–10187.
- Golberg A, Villiger M, Felix Broelsch G, et al. Skin regeneration with all accessory organs following ablation with irreversible electroporation. J Tissue Eng Regen Med. 2018;12(1):98–113.
- Golberg A, Villiger M, Khan S, et al. Preventing Scars after Injury with Partial Irreversible Electroporation. J Invest Dermatol. 2016;136(11):2297–2304.
- Marignol L, Coffey M, Lawler M, et al. Hypoxia in prostate cancer: a powerful shield against tumour destruction? Cancer Treatment Reviews. 2008;34(4):313–327.
- Euhus DM, Hudd C, LaRegina MC, et al. Tumor measurement in the nude mouse. J Surg Oncol. 1986;31(4):229–234.
- Milross CG, Tucker SL, Mason KA, et al. The effect of tumor size on necrosis and polarographically measured pO2. Acta Oncologica. 1997;36(2):183–189.
- DiPietro LA. Angiogenesis and scar formation in healing wounds. Curr Opin Rheumatol. 2013;25(1):87–91.
- Wong VW, Rustad KC, Akaishi S, et al. Focal adhesion kinase links mechanical force to skin fibrosis via inflammatory signaling. Nat Med. 2011;18(1):148–152.
- Hotker AM, et al. Temporal changes in MRI appearance of the prostate after focal ablation. Abdom Radiol. 2019;44(1):272–278.