Abstract
Background: One of the most challenging environmental extremes is immersion in cold/icy water, and consequent common assumption is that even a brief exposure to cold can lead to cold-related illnesses. The increase in the concentrations of the stress hormones cortisol, epinephrine (Epi), and norepinephrine (NE) in response to acute cold stress are thought to suppress the release of proinflammatory cytokines. No previous study has explored the residual consequences of whole-body short-term cold-water immersion (CWI; 14 °C for 10 min) on the immune response in healthy non-acclimated young adult men (aged 20–30 years).
Materials and methods: In the current study, we tested the hypothesis that short-term acute whole-body CWI would induce high blood levels of cortisol, NE, and Epi, which in turn would increase circulating leukocyte numbers and delay the production of proinflammatory cytokines (tumor necrosis factor α (TNF-α), interleukin 1β (IL-1β), and IL-6).
Results: Short-term whole-body CWI produced a stressful physiological reaction, as manifested by hyperventilation and increased muscle shivering, metabolic heat production, and heart rate. CWI also induced the marked release of the stress hormones Epi, NE, and cortisol. The change in IL-6 concentration after CWI was delayed and TNF-α production was decreased, but IL-1β was not affected within 48 h after CWI. A delayed increase in neutrophil percentage and decrease in lymphocyte percentage occurred after CWI.
Conclusion: These findings suggest that, even though CWI caused changes in stress and immune markers, the participants showed no predisposition to symptoms of the common cold within 48 h after CWI.
Introduction
Accidental falls into cold/icy water are relatively common in some recreational and occupational activities. The worldwide popularity of wintertime recreational activities, open cold-water swimming, and cold/icy water immersion immediately after acute exercise has led to an increase in the total number of people exposed to extreme cold environments [Citation1–5]. In non-cold-adapted people, initial head-out cold-water immersion (CWI) at a water temperature below 15 °C is a physiologically stressful event that induces a response known as the “cold shock” response, which can be particularly hazardous [Citation2,Citation6,Citation7]. This response occurs exclusively via stimulation of low-temperature-sensitive receptors (e.g. TRPM8 and/or TRPA1) in cutaneous nerve endings [Citation8–10], which evokes inspiratory gasps, hyperventilation, tachycardia, peripheral vasoconstriction, and increased blood pressure.
Interestingly, respiratory cold shock is maximal during naked immersion at a water temperature of 10–15 °C but is no greater during immersion in water at 5 °C [Citation11]. Cold shock subsides during the first 2–3 min after the initial peak, after which there is a gradual thermoregulatory activation shift via a decrease in discharge frequency of cold-sensitive fibers to a less stressful physiological condition, which can be tolerated for hours [Citation7,Citation12]. The available evidence suggests that even brief exposure to acute cold stress leads to increased circulatory levels of the catecholamine hormones norepinephrine (NE) and epinephrine (Epi), and the glucocorticoid hormone cortisol [Citation13,Citation14]. Immersion in 10 °C water for only 2 min produces a large (2-fold) increase in plasma NE concentration [Citation13].
Low levels of glucocorticoids are generally permissive or stimulatory, whereas higher levels of glucocorticoids are inhibitory, of physiological processes [Citation15]. The increase in the concentrations of NE, Epi, and cortisol has been proposed to suppress the production of proinflammatory cytokines tumor necrosis factor α (TNF-α), interleukin 1β (IL-1β), and IL-6 [Citation16–18]. These immune-specific inflammation-modifying cytokines are essential for the optimal activation of host defenses and survival in response to infection [Citation19,Citation20]. However, exposure to a dangerous combination of proinflammatory cytokines (e.g. TNF-α and IL-1β) [Citation21] and dysregulated overexpression of these cytokines can lead to capillary leakage, shock, and tissue damage [Citation22,Citation23].
Whole-body CWI at 14 °C for 60–170 min maintains at normal level the responses of these cytokines despite evoked stress [Citation24–27]. Suppressed cytokine response may be explained by the observation that exposure to cold stress for more than 40 min decreases body temperature, which then delays and prolongs cytokine expression at a higher level [Citation28] and causes the release of cortisol, Epi, and NE [Citation24,Citation26]. Cortisol then binds to the glucocorticoid receptors and Epi and NE bind to the β2-adrenoceptors [Citation29], which suppresses the synthesis of proinflammatory cytokines via interference with the function of the proinflammatory transcription activator nuclear factor kappa-light-chain-enhancer of activated B-cell signaling (NF-κB) [Citation30].
Adrenalectomy, which eliminates the glucocorticoid and catecholamine stress response, also eliminates the stress-induced increase in cell-mediated immunity [Citation30], which suggests an important role of these stress hormones in immunomodulation. Glucocorticoid [Citation31] and catecholamine [Citation32] hormones induce rapid and significant changes in leukocyte distribution [Citation33]. These findings suggest that immune cells can be affected by acute stress. Therefore, it is reasonable to suggest that the return of these stress hormones to normal levels after cold stress may gradually (re)activate the expression of proinflammatory cytokines.
The research performed to date on the effects of whole-body short-term acute CWI on the natural physiological stress and immune responses is limited to observations of participants who were experienced (adapted) cold-water swimmers, athletes who had exercised acutely in the cold, or athletes who were immersed in cold/icy water as recovery after acute exercise [Citation2,Citation5,Citation34–39]. Given these complex conditions, it is not possible to separate the effects of, for example, acute exercise and cold adaptation [Citation2,Citation40]. Both exercise and cold cause physiological stress, and their combined effects might exceed the individual effects of either [Citation40].
The extent to which acute whole-body short-term CWI alone triggers a residual immune response based on a systematic cascade of blood markers has not been studied in healthy nonacclimated young adults. Therefore, the purpose of this study was to investigate the 48 h kinetics of stress and markers of innate and specific immunity in response to 10 min of CWI at 14 °C in young healthy adult men. The time and temperature were chosen because they correspond to the most hazardous aspect of CWI (i.e. likely to induce cold shock). Given the relationship between changes in body temperature, stress hormone levels, complete blood count, and proinflammatory cytokines, short-term acute CWI may significantly affect the post-CWI kinetics of these markers of stress (cortisol, Epi, and NE levels), and innate immunity (leukocyte, neutrophil, lymphocyte, and monocyte counts and distribution), and specific immunity (TNF-α, IL-1β, and IL-6 levels). We hypothesized that CWI would induce high circulating levels of cortisol, NE, and Epi, which in turn would increase circulating leukocyte numbers and delay the production of proinflammatory cytokines.
Materials and methods
Participants
Participants were excluded from this study if they smoked or had Raynaud’s syndrome, asthma, a neurological pathology, or another condition that could be worsened by acute exposure to cold (14 °C) water. The inclusion criteria were as follows: i) men; ii) age between 20 and 30 years; iii) no excessive regular sport activities (i.e. <3 times per week and ≤150 min of moderate intensity or ≤75 min of vigorous intensity activity per week); iv) no involvement in any temperature-manipulation program or extreme temperature exposure for ≥3 months; v) no medications and dietary supplements that could affect experimental variables; vi) no blood/needle phobia; and vii) had a regular sleep schedule (7–9 h of sleep per night). Considering that most workers at risk of cold-water exposure (fishermen, sailors, military personnel, ice road truckers, etc.) are male, we chose to include only male subjects. Twelve men met the inclusion criteria and agreed to participate in this study. The physical characteristics of the participants are presented in . Written informed consent was obtained from all participants after explanation of all details of the experimental procedures and the associated discomforts and risks. All procedures were approved by the Human Research Ethics Committee and were conducted according to the guidelines of the Declaration of Helsinki. The participants were in self-reported good health, and this was confirmed by a medical history and physical examination.
Table 1. Physical characteristics of the participants in the study.
Experimental design
The study comprised a control (CON) trial and an experimental (EXP) trial involving whole-body CWI (14 °C for 10 min). These trials were performed in a balanced random order (cross-over design) at least 1 week apart. We used the IBM Statistical Package for the Social Sciences (SPSS) for Windows version 22.0 (IBM Corp., Armonk, NY, USA) to determine which trial each participant underwent first. The experiment was designed to evaluate the residual effects (within 48 h after cold exposure) on immune and blood markers of stress after acute short-term whole-body CWI.
One week before the experiment, the participants were familiarized with the laboratory setting and test procedures. They were instructed to sleep for >8 h the night before the experiment and to refrain from strenuous exercise, alcohol, and caffeine for at least 24 h before the experiment. It is established that sleeping less than 7 h per night is associated with impaired immune function [Citation41]. Thus, any participant who mentioned that he had slept less than 7 h the night before the experiment was excluded from participation. To avoid the effect of diet-induced thermogenesis, the participants fasted from 10 h before the onset of the trial [Citation42] until 2 h after the cold exposure.
The experiments began in June and continued for 6 months. A schematic representation of the experimental design is presented in . In the EXP trial, the acute cold-stress procedure was performed in the morning (08:00–10:00 AM). The participant donned swimming briefs covering the genitals and buttocks (∼7% of body surface area [Citation43]) and self-inserted a rectal probe, after which the strap used for recording heart rate (HR) was attached to the chest. Next, for blood sampling, a 20-gauge 32 mm intravenous catheter was inserted into the right antecubital vein and covered with waterproof protector while the participant sat quietly at rest. Catheter patency was maintained by flushing the device with heparinized saline (10 U/ml). The participant was then asked to lie in the semirecumbent position for 30 min at an ambient temperature of 24 °C and 60% relative humidity. The resting pulmonary gas exchange values and HR were recorded during the last 20 min of this period. The resting preimmersion values of skin (Tsk), muscle (Tmu), and rectal (Tre) temperatures were measured, and blood samples were collected and stored for later analysis.
An electromyography (EMG) sensor was attached to the pectoralis muscle, and the CWI procedure began (). The participant was fully immersed in a semirecumbent position up to the level of the manubrium in a 14 °C stirred-water bath. The water bath temperature was adjusted with the addition of 8 °C water. Tre, pulmonary gas exchange values, and HR were recorded continuously throughout CWI. After 10 min of immersion, the participant exited from the bath and was towel dried, Tsk, Tre, and Tmu were measured, and blood samples were collected. The participant then lay in the semirecumbent position for 1 h in a controlled laboratory environment at ambient (24 °C) temperature. Resting Tre was measured, and HR, pulmonary gas exchange values, and thermoregulatory muscle activation (EMG) were recorded every 5 min. Tsk was measured 5, 15, and 30 min, and 1 h after CWI, and Tmu was measured 1 h after CWI. Each participant was asked to evaluate his subjective perception every 5 min throughout the immersion and recovery period. The blood samples were collected at 15 and 30 min, and 1, 2, 4, 6, 12, 24, and 48 h after CWI. The participants were fed a light meal (warm soup and fresh green salad) 2 and 6 h after CWI.
In the CON trial, the participant remained at an ambient temperature of 24 °C and 60% relative humidity without CWI for 10 min, and all aspects related to nutrition, time of day, and time points of blood sampling, temperature, EMG-based measurement, pulmonary gas exchange, and HR measurements were the same as those used in the EXP trial.
Experimental measurements
Anthropometric measurements
Body mass and body fat were measured, and the body mass index was calculated. Body mass was measured as the nude body mass using a body composition analyzer (Tanita, TBF-300, Arlington Heights, IL). Body surface area was estimated in m2 according to the formula 128.1 × weight 0.44 × height 0.60 [Citation44]. Skinfold thickness was calculated as the average thickness of 10 skinfold sites (chin, subscapular, chest, side, suprailium, abdomen, triceps, thigh, knee, and calf) [Citation45] using a medical skinfold caliper (Seahan, SH5020, Masan, South Korea).
Body temperature measurements
Tre was measured before CWI and then every 5 min until 1 h after CWI (). Tre was measured using a thermocouple (Rectal Probe; Ellab, Hvidovre, Denmark; accuracy, ±0.01 °C) inserted to a depth of 12 cm past the anal sphincter. The rectal thermistor sensor was placed by each participant. Tsk was measured before, immediately after, and 5, 15, 30, and 60 min after CWI with thermistors (Skin/Surface Probe, DM852, Ellab; accuracy, ±0.01 °C) taped at three sites: back, thigh, and forearm. The mean Tsk was calculated using the Burton [Citation46] equation as Tsk = 0.5back + 0.36thigh + 0.14forearm. Tmu was measured before, immediately after, and 1 h after CWI with a needle microprobe (Intramuscular Probe, MKA, Ellab; accuracy, ±0.01 °C) inserted at a depth of ∼3.5 cm under the skin covering the lateral portion of the gastrocnemius muscle in the right leg. For skin preparation before each Tmu measurement, the skin was shaved and disinfected before and after the insertion of the microprobe using a cotton wool pad soaked with medicinal alcohol. No local anesthesia was administered before insertion. After the first measurement, the insertion area was marked with a circle with a diameter of 0.5 cm to ensure that the same insertion point was used in later measurements.
Spirometry and HR measurements
A mobile spirometry system (Oxycon Mobile, Jaeger/VIASYS Healthcare, Hochberg, Germany) was used to measure changes in ventilation (VE), oxygen consumption (VO2) and carbon dioxide production (VCO2) on a breath-by-breath basis. Automatic calibration of the gas analyzer and delay time was performed before the measurements, as described by the manufacturer; a calibration gas at 180 kPa (15.2% O2, 5.02% CO2, and 79.62% N2) was supplied to attain gain, offset, and delay times within 1%. Metabolic heat production (MHP) (in W) was calculated from the respiratory gas exchange measurements of VO2 (in l/min) and the respiratory exchange ratio (RER = VCO2/VO2) according to Peronnet and Massicotte [Citation47] as MHP = (281.65 + 80.65 × RER) × VO2. HR was measured at rest, throughout CWI, and during the 1 h post-CWI recovery period using an HR monitor (S-625X, Polar Electro, Kempele, Finland).
EMG-based measurement of thermoregulatory muscle activation
Because heat-generating muscle activation is pronounced in the chest region, we measured EMG signals in the pectoralis major muscle [Citation48,Citation49] during CWI and during the 1 h post-CWI recovery period (). After careful preparation of the skin (shaving, abrading, and cleaning with alcohol wipes) to obtain a low impedance (<10 kΩ), a surface EMG sensor (SX230W, Biometrics Co., Ltd., Gwent, UK) with integrated bipolar Ag–AgCl electrodes (diameter, 10 mm; center-to-center distance, 20 mm) and a differential amplifier (gain, 1000; input impedance, 100 MΩ; input noise, <5 µV; common-mode rejection ratio, >96 dB) was placed on the right pectoralis major muscle. The ground electrode (R206, Biometrics Co., Ltd.) was positioned on the wrist of the right hand. The EMG sensor and ground electrode were connected to a portable data-acquisition unit (DataLOG P3X8, Biometrics Co., Ltd.). Before the measurements, the channel sensitivity was set to 3 V, and the excitation output was set to 4600 mV, as recommended by the manufacturer. The EMG signals were digitized, and files were stored on a computer for subsequent analyses of the root mean square (RMS, in mV) and mean frequency (MnF, in Hz) using dedicated software (Biometrics DataLOG, Gwent, UK).
Subjective ratings
The method described by Cernych et al. [Citation50] was used to measure subjective ratings for thermal and shivering sensations. Thermal sensation ratings ranged from 1 (very cold) to 5 (being neutral). Shivering ratings ranged from 4 (being neutral) to 7 (vigorously shivering). Subjective ratings were recorded at the same time points as the Tre and HR measurements.
Blood variables
Venous blood samples were collected before, immediately after, 15 and 30 min after, and 1, 2, 4, 6, 12, 24, and 48 h after CWI ().
Blood samples were collected by venipuncture into vacuum tubes (EDTA-K3, 3 ml), mixed gently by inverting 8–10 times, and kept at room temperature until the analysis of differential white blood cell counts of leukocytes and subgroups neutrophils, lymphocytes, and monocytes. Blood samples were analyzed 1–2 h after blood collection using an automated hematology analyzer (XE-5000, Sysmex Corp., Kobe, Japan).
Blood samples for the measurement of Epi and NE concentrations were collected in vacuum tubes using EDTA as an anticoagulant (EDTA-K3, 3 ml), mixed gently by inverting 8–10 times, and kept at 2–8 °C until centrifugation. Blood samples were centrifuged at 1200 × g for 15 min within 30 min of blood collection. Plasma samples were separated as soon as possible (maximum, 10–15 min) from the red blood cells after centrifugation and kept at −70 °C until analysis. The NE and Epi concentrations were measured in duplicate using a CatCombi enzyme-linked immunosorbent assay (ELISA) kit (Gemini Analyzer, Stratec Biomedical GmbH, Birkenfeld, Germany).
Blood samples for the measurement of total cortisol and proinflammatory cytokine IL-1β, IL-6, and TNF-α concentrations were collected by venipuncture into vacuum tubes for serum separation using a gel separator (5 ml). Blood samples were collected in the morning (8:00–8:30 AM) about 1 h after awakening when the cortisol concentration in the blood was expected to be close to the peak of the natural cortisol cycle [Citation51]. Blood samples were allowed to clot, and the serum was separated by centrifugation at 1200 × g for 15 min. The serum samples were aliquoted and stored at −70 °C until analysis. The concentrations of IL-1β, IL-6, and TNF-α were measured in duplicate using an ELISA (Gemini Analyzer, Stratec Biomedical GmbH, Birkenfeld, Germany), and cortisol concentration was measured in duplicate using an automated enzyme immunoassay analyzer (AIA-2000; Tosoh Corp., Tokyo, Japan).
Statistical analysis
The data were tested for normality using the Shapiro–Wilk test before parametric statistical analyses, and all data were found to be normally distributed. Descriptive data are presented as mean ± standard deviation (SD). Two-way analysis of variance (ANOVA) for repeated measures was used to analyze the effects of the two temperature conditions (EXP vs. CON) as the between-trial factors and the 11 sampling time points (before and immediately after, 15 and 30 min after, and 1, 2, 4, 6, 12, 24, and 48 h after CWI) as the within-subject factors on the dependent blood variables. Two-way ANOVA for repeated measures was also performed to study the effects of the two temperature conditions (EXP vs. CON) as the between-trial factors and appropriate time points as the within-subject factor on changes in body temperature, EMG muscle activity, HR, and spirometry markers. When significant main effects were found, Sidak’s post hoc adjustment was used for multiple comparisons across a set of conditions within each repeated-measures ANOVA. The nonparametric Wilcoxon’s signed-rank test for two related samples (preimmersion value vs. values obtained at 5, 10, 15, 20, 25, 30, 35, 40, 45, 50, 55, 60, 65, and 70 min) was used to compare changes in subjective ratings of cold perception (thermal sensation and shivering). The partial eta squared (ηp2) was estimated as a measure of the condition and time effect size. Statistical significance was set at p < 0.05. Statistical analyses were performed using IBM SPSS Statistics software (v. 22).
Results
Spirometry and HR responses
In the EXP trial, the initial first minute of whole-body immersion in 14 °C water resulted in ∼2.5-fold increase in VO2 (p < 0.001; ηp2 = 0.42; ), ∼3.5-fold increase in VCO2 (p < 0.001; ηp2 = 0.54; ), ∼3-fold increase in MHP (p < 0.001; ηp2 = 0.46; ), and ∼4-fold increase in VE (p < 0.001; ηp2 = 0.64; ). The trial × time interaction was significant for all variables (p < 0.001; ηp2 > 0.5; ). Participants adjusted to this initial cold shock response within a few minutes of immersion, as shown by a lower spirometry activity response during the remaining (1–10 min) CWI time. However, this value remained significantly higher than the preimmersion value (p < 0.001; ηp2 > 0.35). During the post-CWI recovery period, compared with the preimmersion values, none of the spirometry parameters returned to their preimmersion level within the 1 h recovery period in the thermoneutral environment (p < 0.05; ηp2 > 0.25; ). HR increased in response to CWI, with a significant trial × time interaction effect (p < 0.001; ηp2 = 0.52; ) and returned to the preimmersion value 15 min after CWI (p > 0.05; ηp2 < 0.1).
Figure 2. Time-dependent changes in oxygen consumption (VO2) (A), carbon dioxide production (VCO2) (B), metabolic heat production (MHP) (C), and ventilation (VE) (D) in the experimental (EXP) and control (CON) trials. The black-outlined yellow dots represent the data during cold-water immersion (CWI). Immediately after CWI, 5–7 min was needed to obtain the body temperature measurements, take blood samples, transfer the participant to the chair, and restart and set the gas analyzer; therefore, respiratory gas exchange measurements were not taken during this period. *p < 0.05 compared with the preimmersion value; #p < 0.05 compared with the CON trial. The data are expressed as the mean ± SD.
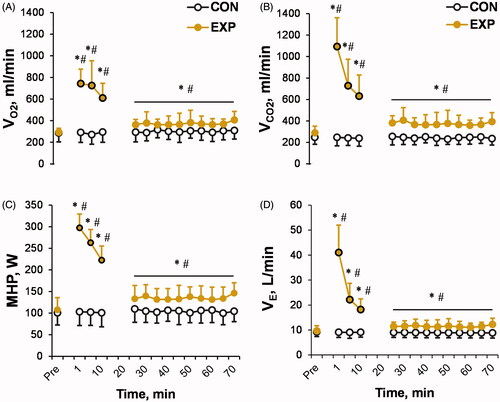
Figure 3. Time-dependent changes in rectal (A), skin (C), and muscle (D) temperatures (T), and heart rate (B) in the experimental (EXP) and control (CON) trials. The black-outlined yellow dots represent the data during the cold-water immersion (CWI). *p < 0.05 compared with the preimmersion value; #p < 0.05 compared with the control (CON) trial. The data are expressed as the mean ± SD.
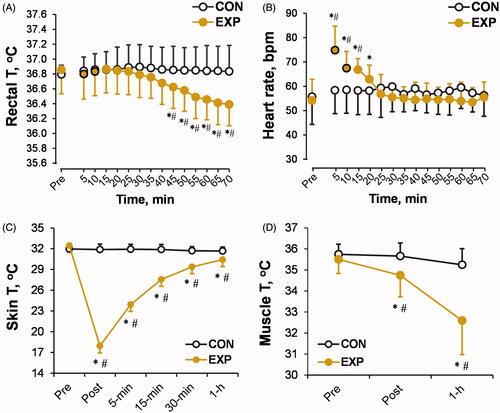
Body temperature response
At the end of CWI, Tsk (p < 0.001; ηp2 = 0.71; ) and Tmu (p < 0.001; ηp2 = 0.48; ) decreased, with a significant trial × time interaction (p < 0.001; ηp2 > 0.45), but Tre remained unaffected (p > 0.05; ). Interestingly, Tmu and Tre decreased progressively in the post-cooling thermoneutral environment as measured during the 1 h recovery after CWI (trial × time interaction effect: p < 0.001; ηp2 > 0.48). Tsk showed a trend toward recovery, but its values did not reach the preimmersion level 1 h after CWI (p < 0.01; ηp2 > 0.3).
EMG-based heat-generating muscle activation response
As shown in , the muscle EMG root mean square (RMS) and EMG mean frequency (MnF) activity changed during and after CWI, with a significant trial × time interaction (p < 0.001; ηp2 > 0.45). During the post-CWI recovery period, the EMG RMS was lower and the muscle EMG MnF was higher than their respective values obtained during CWI (main time effect: compared with the 1, 5, and 10 min time points during CWI: p < 0.05; ηp2 > 0.25).
Figure 4. Time-dependent changes in the root mean square (RMS) (A) and mean frequency (MnF) (B) of electromyography (EMG)-based pectoralis major muscle activity in the experimental (EXP) and control (CON) trials, and subjective thermal (C) and shivering (D) sensations in the EXP trial. The black-outlined yellow dots represent the data during the cold-water immersion (CWI). The dashed line represents the mean baseline value. Immediately after CWI, 5–7 min was needed to obtain the measurements, take blood samples, and transfer the participant to the chair; therefore, EMG activity was not measured during this period. *p < 0.05 compared with the preimmersion value; †p < 0.05 compared with the immersion time points (1, 5, or 10 min); #p < 0.05 compared with the CON trial. The data are expressed as the mean ± SD.
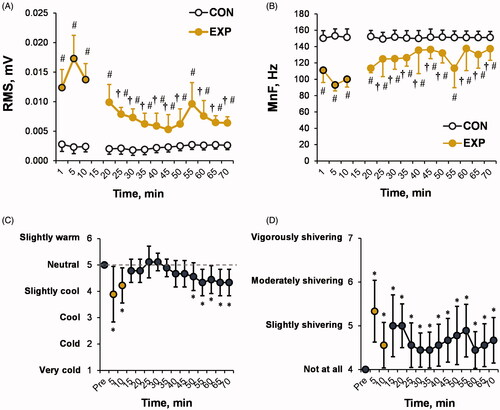
Perception score response
At 5 and 10 min of CWI, the thermal sensation score () was lower (p < 0.01) and the shivering perception score () was higher than their respective preimmersion values (p < 0.01). The participants reported “slightly cool” and “slightly shivering”, respectively. The thermal sensation score returned to the preimmersion value (i.e. neutral) 5 min after CWI, after which, in parallel with the post-CWI decrease in Tre, the participants reported feeling “slightly cool” from 40 to 60 min after CWI (compared with the pre-CWI value: p < 0.05). The perception of “slightly shivering” persisted throughout the post-CWI recovery period (compared with the preimmersion value: p < 0.05).
Blood markers
The baseline concentrations of all blood markers measured in this study did not differ between the CON and EXP trials (p < 0.05).
Stress hormones
The preimmersion concentration of cortisol was measured at the expected diurnal peak (8:00 AM). CWI triggered an increase in cortisol concentration and prolongation of this diurnal peak until 2 h after cold exposure, with a significant trial × time interaction (p < 0.05; ηp2 = 0.22; ). A significantly higher concentration of cortisol was also observed at 12 h after CWI (compared with CON trial: p < 0.01; ηp2 > 0.21). Thereafter, cortisol returned to the value expected for the natural diurnal cycle.
Figure 5. Time-dependent changes in cortisol (A), epinephrine (B), and norepinephrine (C) concentrations in the experimental (EXP) and control (CON) trials. *p < 0.05 compared with the preimmersion value; #p < 0.05 compared with the CON trial. The data are expressed as the mean ± SD.
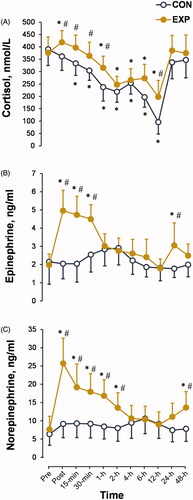
In the EXP trial, CWI resulted in ∼1.5-fold increase in Epi () and ∼2.5-fold increase in NE () concentrations immediately after CWI (time effect: p < 0.001; ηp2 > 0.5), with a significant trial × time interaction (p < 0.001; ηp2 > 0.4). Epi and NE concentrations were restored to their preimmersion levels 1 and 4 h after CWI, respectively. However, a second peak of Epi was observed at 24 h and a second peak of NE at 48 h after CWI (p < 0.01; ηp2 > 0.25).
White blood cell count
The leukocyte count () and monocyte percentage () were not significantly affected by cold exposure. However, CWI increased the neutrophil percentage and decreased the lymphocyte percentage, with a significant trial × time interaction (p < 0.01; ηp2 > 0.3). The peak changes in these two markers were observed 1 and 2 h after CWI. Both markers returned to the diurnal cycle 4 h after CWI.
Figure 6. Time-dependent changes in leukocyte count (A) and neutrophil (B), lymphocyte (C), and monocyte (D) percentages in the experimental (EXP) and control (CON) trials. *p < 0.05 compared with the preimmersion value; #p < 0.05 compared with the CON trial. The data are expressed as the mean ± SD.
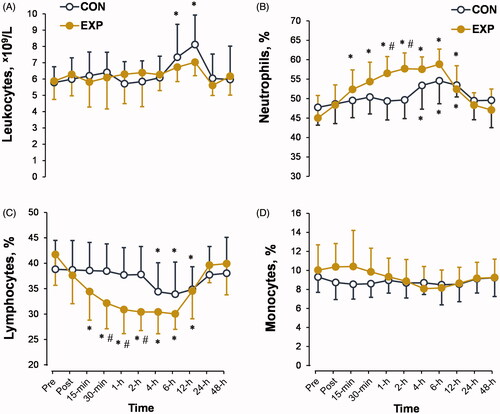
Cytokines
The TNF-α response showed a significant interaction of CWI in the EXP trial × time period (p < 0.01; ηp2 = 0.27; ). The TNF-α concentration decreased from 15 min to 24 h after CWI (main time effect: p < 0.01; ηp2 = 0.27). The peak release of IL-6 was observed at 6 and 12 h after CWI, with a significant trial × time interaction (p < 0.05; ηp2 = 0.21; ). The IL-6 concentration recovered to the preimmersion level 24 h after CWI. IL-1β concentration was unaffected by short-term CWI (p > 0.05; ).
Figure 7. Time-dependent changes in the blood concentrations of proinflammatory cytokines tumor necrosis factor α (TNF-α) (A), interleukin 6 (IL-6) (B), and IL-1β (C) in the experimental (EXP) and control (CON) trials. *p < 0.05 compared with the preimmersion value; #p < 0.05 compared with the CON trial. The data are expressed as the mean ± SD.
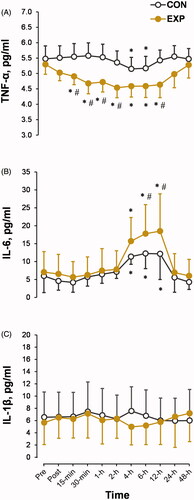
Discussion
Perhaps one of the most challenging environmental extremes is immersion in cold/icy water, which is frequently encountered by athletes, workers in extreme environments, and soldiers [Citation52–54]. CWI causes the release of stress hormones [Citation13,Citation55]. Dhabhar [Citation33] argues that short-term stress readies the immune system to deal with injury or infection. Accordingly, in the present study, we examined whether short-term whole-body immersion (for 10 min) in cold water (14 °C) is a sufficient noninfectious stimulus to modulate the residual kinetic responses of markers of stress and innate and specific immunity within 48 h after cold exposure. We found that short-term whole-body CWI produced a stressful physiological reaction, which was manifested as hyperventilation, increased muscle EMG-based shivering, MHP, and HR. In addition to these stressful physiological responses, CWI induced the marked release of the stress hormones Epi, NE, and cortisol. We found a delayed increase in IL-6 concentration after CWI and a decrease in TNF-α level; however, IL-1β concentration was not affected within 48 h after CWI. A delayed increase in neutrophil percentage and decrease in lymphocyte percentage was also found.
Cortisol, NE, and Epi strongly alter the release of proinflammatory cytokines. The transcription factor NF-κB is a crucial regulator of proinflammatory cytokines, and the glucocorticoid and β2-adrenergic receptors expressed on a variety of immune cells appear to play prominent roles in the regulation of NF-κB activity [Citation56,Citation57]. The release of cortisol and Epi/NE activates glucocorticoid and/or β2-adrenergic receptors by increasing the release of cAMP, which increases NFKBIA gene expression and, in turn, reduces NF-κB activation, thus leading to attenuated synthesis of the proinflammatory cytokines IL-6, IL-1β, and TNF-α [Citation28,Citation29,Citation58]. In the current study, immersion of the whole body in cold water (14 °C) for 10 min increased cortisol, Epi, and NE release, which most likely delayed the response of IL-6 and TNF-α cytokines, possibly via attenuation of NF-κB activation. These results are consistent with those of a study that reported that exposure to circulating 15 °C water for 15 min after an intense bout of resistance exercise (6-fold increase in lactate concentration) resulted in a suppressed and delayed acute (within 60 min) IL-6 and TNF-α cytokine response compared with passive recovery under a thermoneutral condition [Citation5].
In our study, the return of cortisol, Epi, and NE concentrations to preimmersion levels and/or to the natural diurnal cycle kinetics did not parallel the post-CWI cytokine kinetic responses. In addition to cortisol, Epi, and NE, body (cell) temperature itself can regulate NF-κB activity [Citation28,Citation58]. For example, in human peripheral blood mononuclear cells studied in vitro, NF-κB activation at 33 °C was delayed by 1.5 h after stimulation with lipopolysaccharide, which in turn delayed proinflammatory cytokine production compared with that observed at 37 °C [Citation28]. Intriguingly, our findings provide the first evidence that, although Tsk showed a significant trend during recovery to the preimmersion level, Tre and Tmu continued to decrease by about 0.5 °C and 3.0 °C, respectively, within 60 min after CWI. An afterdrop in Tre and Tmu may have occurred because of conductive and convective (via the blood) transfer of cold from the periphery to the core [Citation59–63].
This continued decrease in deep body temperatures after CWI was accompanied by elevated (vs. preimmersion value) MHP and VE, perceptions of increased subjective thermal stress and shivering, and muscle EMG-based activity. Considering that we measured post-CWI body temperatures for only 60 min, we can only speculate that when stress hormone levels, body temperature, and metabolic activity began to return to the baseline (or to the natural circadian cycle kinetics), a gradual increase in NF-κB activation [Citation58] through the activation of the canonical pathway of the NF-κB signaling pathway [Citation64] may have increased synthesis and release of IL-6 cytokine within 6–12 h after CWI. Although mostly regarded as a proinflammatory cytokine, IL-6 also has many regenerative and anti-inflammatory activities [Citation65]. Endogenous IL-6 has been shown to play a crucial anti-inflammatory role in both local and systemic acute inflammatory responses by controlling the level of proinflammatory cytokines [Citation66]. Moreover, IL-6 plays important roles as a myokine in metabolism, browning of white fat, bone formation, hypertrophy, and tumor growth [Citation67].
White blood cells are the first line of defense against many pathogens and tissue damage and have been shown to react promptly (within minutes) to psychologically and physically stressful events. These cells are an important part of the innate immune system [Citation68]. Cytokines are produced by neutrophils, monocytes, and lymphocytes. These cells are the main source of cytokines upon initial contact with potentially pathogenic organisms, and cytokines are thought to play a critical role in immunomodulation [Citation68]. Acute short-term stress induces an initial increase, followed by a decrease, in blood lymphocyte and monocyte counts, as well as an increase in blood neutrophil count [Citation7,Citation69]. Soon after the beginning of stress, the glucocorticoid and catecholamine stress hormones induce the body’s leukocytes to exit the spleen, lung, marginated pool, and other organs, and to enter the blood vessels and lymphatics [Citation69]. In contrast to long-term (60–170 min) CWI [Citation24,Citation26,Citation27], short-term (10 min) CWI in the current study did not alter the leukocyte count or monocyte percentage. However, in agreement with other reports, short-term CWI increased the neutrophil percentage and decreased the lymphocyte percentage. These differences between short- and long-term CWI suggest a dose-dependent effect on the white blood cell count. Considering that the monocyte count was unchanged and that the lymphocyte and neutrophil counts recovered completely within 6–12 h after CWI, it is likely that the decrease in lymphocyte percentage and increase in neutrophil percentage reflects a redistribution of immune cells from the blood to other organs rather than the destruction of these cells [Citation70]. Interestingly, the recovery of lymphocyte and neutrophil percentages paralleled to some extent the changes in TNF-α cytokine synthesis and release, which may indicate a reduction in the number of available sources of cytokines.
One common assumption is that even a brief exposure to cold can lead to cold-related illnesses. To date, the effect of cold exposure on the predisposition to illness remains poorly understood, and better understanding of the immune responses during cold exposure and its residual effects may explain an organism’s susceptibility to infection. A previous study supports the notion that induction of proinflammatory cytokines may correlate with an increased risk of upper respiratory infection [Citation71]. Another study reported that increased cortisol secretion may also increase the susceptibility to upper respiratory infection [Citation72]. The findings of our present study suggest that even though CWI caused changes in stress and immune markers, there was no predisposition to symptoms of the common cold in the participants within 48 h after CWI.
This study has some limitations. The number of participants is small, and the results are limited to only young (aged 20–30 years) healthy men. The anatomical, physiological, and functional differences between men and women [Citation73–76] and children [Citation77], and the weakened thermoregulatory response to temperature extremes in older people [Citation78,Citation79] suggest that the results of the present study may not be directly applicable to women, children, or older people. Another possible limitation is that we used static (i.e. without human movement) CWI. As in most published research, our set water temperature (14 °C) did not include the full range of temperatures routinely encountered in open water by workers or athletes during the winter months [Citation40]. In addition, there may be differences in the responses to static CWI and cold-water swimming because exercise and cold independently impose stress on the human body, and, therefore, multiple stressors applied together may have a combined effect on immunomodulation. Moreover, an accidental fall into cold/icy open water would induce strong survival (panic)-related stress [Citation2], which may further modify the neuroendocrine and immune responses. Notably, no analysis of NF-κB activation was performed in this study, which weakens the direct-evidence-based interpretation of the data presented.
In conclusion, we have shown that immersion of the whole body in cold water (14 °C) for 10 min led to changes in thermoregulation, stress hormone levels, immune cell profile, and cytokine levels. We found a delayed change in IL-6 concentration after CWI and that the blood TNF-α level decreased but the IL-1β cytokine level was not affected within 48 h after CWI. Short-term CWI resulted in a delayed increase in neutrophil percentage and decrease in lymphocyte percentage. Whether and to what extent these changes affect the infectious processes in healthy people is unclear and should be examined in future studies.
Disclosure statement
No potential conflict of interest was reported by the author(s).
References
- Bleakley C, Davison G. What is the biochemical and physiological rationale for using cold-water immersion in sports recovery? A systematic review. Br J Sports Med. 2009;44:179–187.
- Tipton MJ, Collier N, Massey H, et al. Cold water immersion: kill or cure? Experimental Physiology. 2017;102:1335–1355.
- Peake JM, Roberts LA, Figueiredo VC, et al. The effects of cold water immersion and active recovery on inflammation and cell stress responses in human skeletal muscle after resistance exercise. The Journal of Physiology. 2017;595:695–711.
- Procter E, Brugger H, Burtscher M. Accidental hypothermia in recreational activities in the mountains: a narrative review. Scand J Med Sci Sports. 2018;28:2464–2472.
- Earp JE, Hatfield DL, Sherman A, et al. Cold-water immersion blunts and delays increases in circulating testosterone and cytokines post-resistance exercise. Eur J Appl Physiol. 2019;119:1901–1907.
- Tipton MJ. The initial responses to cold-water immersion in man. Clin Sci (Lond). 1989;77:581–588.
- Brazaitis M, Eimantas N, Daniuseviciute L, et al. Time course of physiological and psychological responses in humans during a 20-day severe-cold–acclimation programme. PLOS One. 2014;9:e94698.
- Craig A. Interoception: the sense of the physiological condition of the body. Curr Opin Neurobiol. 2003;13:500–505.
- Nakamura K, Morrison SF. Preoptic mechanism for cold-defensive responses to skin cooling. J Physiol. 2008;586:2611–2620.
- Vay L, Gu C, McNaughton PA. The thermo-TRP ion channel family: properties and therapeutic implications. Br J Pharmacol. 2012;165:787–801.
- Tipton MJ, Stubbs DA, Elliott DH. Human initial responses to immersion in cold water at three temperatures and after hyperventilation. Journal of Applied Physiology. 1991;70:317–322.
- Hensel H, Zotterman Y. The effect of menthol on the thermoreceptors. Acta Physiol Scand. 1951;24:27–34.
- Johnson DG, Hayward JS, Jacobs TP, et al. Plasma norepinephrine responses of man in cold water. Journal of Applied Physiology. 1977;43:216–220.
- Houben H, Thien T, Wijnands G, et al. Effects of cold exposure on blood pressure, heart rate and forearm blood flow in normotensives during selective and non-selective beta-adrenoceptor blockade. Br J Clin Pharmacol. 1982;14:867–870.
- Sapolsky RM, Romero LM, Munck AU. How do glucocorticoids influence stress responses? Integrating permissive, suppressive, stimulatory, and preparative actions. Endocr Rev. 2000;21:55–89.
- Elenkov IJ, Chrousos GP. Stress hormones, proinflammatory and antiinflammatory cytokines, and autoimmunity. Ann N Y Acad Sci. 2002;966:290–303.
- Miller GE, Cohen S, Ritchey AK. Chronic psychological stress and the regulation of pro-inflammatory cytokines: a glucocorticoid-resistance model. Health Psychol. 2002;21:531–541.
- Tian R, Hou G, Li D, et al. A possible change process of inflammatory cytokines in the prolonged chronic stress and its ultimate implications for health. ScientificWorldJournal. 2014;2014:780616.
- Cross A, Asher L, Seguin M, et al. The importance of a lipopolysaccharide-initiated, cytokine-mediated host defense mechanism in mice against extraintestinally invasive Escherichia coli. J Clin Invest. 1995;96:676–686.
- Leon LR, White AA, Kluger MJ. Role of IL-6 and TNF in thermoregulation and survival during sepsis in mice. Am J Physiol. 1998;275:R269–277.
- Doherty GM, Lange JR, Langstein HN, et al. Evidence for IFN-gamma as a mediator of the lethality of endotoxin and tumor necrosis factor-alpha. The Journal of Immunology. 1992;149:1666–1670.
- Munoz C, Carlet J, Fitting C, et al. Dysregulation of in vitro cytokine production by monocytes during sepsis. J Clin Invest. 1991;88:1747–1754.
- Tracey KJ, Lowry SF. The role of cytokine mediators in septic shock. Adv Surg. 1990;23:21–56.
- Janský L, Šrámek P, Šavlíková J, et al. Change in sympathetic activity, cardiovascular functions and plasma hormone concentrations due to cold water immersion in men. Europ J Appl Physiol. 1996;74:148–152.
- Solianik R, Skurvydas A, Vitkauskienė A, et al. Gender-specific cold responses induce a similar body-cooling rate but different neuroendocrine and immune responses. Cryobiology. 2014;69:26–33.
- Brazaitis M, Eimantas N, Daniuseviciute L, et al. Two Strategies for Response to 14 °C Cold-Water Immersion: is there a difference in the response of motor, cognitive, immune and stress markers? PLOS One. 2014;9:e109020.
- Eimonte M, Eimantas N, Daniuseviciute L, et al. Recovering body temperature from acute cold stress is associated with delayed proinflammatory cytokine production in vivo. Cytokine. 2021;:155510.
- Kimura A, Sakurada S, Ohkuni H, et al. Moderate hypothermia delays proinflammatory cytokine production of human peripheral blood mononuclear cells. Crit Care Med. 2002;30:1499–1502.
- Platzer C, Döcke W-D, Volk H-D, et al. Catecholamines trigger IL-10 release in acute systemic stress reaction by direct stimulation of its promoter/enhancer activity in monocytic cells. Journal of Neuroimmunology. 2000;105:31–38.
- Dhabhar FS, McEwen BS. Enhancing versus suppressive effects of stress hormones on skin immune function. PNAS. 1999;96:1059–1064.
- Dhabhar FS, Miller AH, McEwen BS, et al. Stress-induced changes in blood leukocyte distribution. Role of adrenal steroid hormones. J Immunol. 1996;157:1638–1644.
- Mills PJ, Ziegler MG, Rehman J, et al. Catecholamines, catecholamine receptors, cell adhesion molecules, and acute stressor-related changes in cellular immunity. Adv Pharmacol. 1998;42:587–590.
- Dhabhar FS. Effects of stress on immune function: the good, the bad, and the beautiful. Immunol Res. 2014;58:193–210.
- Brenner IK, Castellani JW, Gabaree C, et al. Immune changes in humans during cold exposure: effects of prior heating and exercise. J Appl Physiol (1985). 1999;87:699–710.
- Rhind SG, Castellani JW, Brenner IK, et al. Intracellular monocyte and serum cytokine expression is modulated by exhausting exercise and cold exposure. Am J Physiol Regul Integr Comp Physiol. 2001;281:R66–75.
- Lombardi G, Ricci C, Banfi G. Effect of winter swimming on haematological parameters. Biochem Med (Zagreb. ). 2011;21:71–78.
- Kim K, Suzuki K, Peake J, et al. Physiological and leukocyte subset responses to exercise and cold exposure in cold-acclimatized skaters. Biol Sport. 2014;31:39–48.
- Gagnon DD, Gagnon SS, Rintamäki H, et al. The effects of cold exposure on leukocytes, hormones and cytokines during acute exercise in humans. PLoS One. 2014;9:e110774.
- Siqueira AF, Vieira A, Bottaro M, et al. Multiple cold-water immersions attenuate muscle damage but not alter systemic inflammation and muscle function recovery: a parallel randomized controlled trial. Sci Rep. 2018;8:10961.
- LaVoy ECP, McFarlin BK, Simpson RJ. Immune Responses to Exercising in a Cold Environment. Wilderness & Environmental Medicine. 2011;22:343–351.
- Watson NF, Badr MS, Belenky G, et al. Recommended amount of sleep for a healthy adult: a joint consensus statement of the american academy of Sleep Medicine and Sleep Research Society. Sleep. 2015;38:843–844.
- van Ooijen AMJ, van Marken Lichtenbelt WD, van Steenhoven AA, et al. Seasonal changes in metabolic and temperature responses to cold air in humans. Physiol gy Behav. 2004;82:545–553.
- Heerfordt IM, Philipsen PA, Larsen BØ, et al. Long-term Trend in Sunscreen Use among Beachgoers in Denmark. Acta Derm Venereol. 2017;97:1202–1205.
- Tikuisis P, Meunier P, Jubenville C. Human body surface area: measurement and prediction using three dimensional body scans. Eur J Appl Physiol. 2001;85:264–271.
- McArdle WD, Magel JR, Gergley TJ, et al. Thermal adjustment to cold-water exposure in resting men and women. J Appl Physiol Respir Environ Exerc Physiol. 1984;56:1565–1571.
- Burton AC. Human CalorimetryII. The average temperature of the tissues of the body: three figures. J Nutr. 1935;9:261–280.
- Péronnet F, Massicotte D. Table of nonprotein respiratory quotient: an update. Canadian Journal of Sport Sciences = Journal Canadien Des Sciences du Sport. 1991;16:23–29.
- Ooijen AMJ, van Lichtenbelt WD, van M, Steenhoven AA. v, et al. Cold-induced heat production preceding shivering. Br J Nutr. 2005;93:387–391.
- Wyckelsma VL, Venckunas T, Houweling PJ, et al. Loss of α-actinin-3 during human evolution provides superior cold resilience and muscle heat generation. Am J Hum Genet. 2021;108:446–457.
- Cernych M, Baranauskiene N, Eimantas N, et al. Physiological and Psychological Responses during Exercise and Recovery in a Cold Environment Is Gender-Related Rather Than Fabric-Related. Front Psychol. 2017;8:1664–1078.
- Smyth JM, Ockenfels MC, Gorin AA, et al. Individual differences in the diurnal cycle of cortisol. Psychoneuroendocrinology. 1997;22:89–105.
- Wittmers LE, Savage MV. Cold Water Immersion. Med Aspects Harsh Environ. 2001;1:531–552.
- Barsky SM, Long D, Stinton B. Dry suit diving: a guide to diving dry. Oxnard (CA): Hammerhead Press; 2006.
- Tipton M, Bradford C. Moving in extreme environments: open water swimming in cold and warm water. Extreme Physiol Med. 2014;3:12.
- Kauppinen K, Pajari-Backas M, Volin P, et al. Some endocrine responses to sauna, shower and ice water immersion. Arctic Med Res. 1989;48:131–139.
- Abraham E, Matthay MA, Dinarello CA, et al. Consensus conference definitions for sepsis, septic shock, acute lung injury, and acute respiratory distress syndrome: time for a reevaluation. Critical Care Medicine. 2000;28:232–235.
- Padgett DA, Glaser R. How stress influences the immune response. Trends in Immunology. 2003;24:444–448.
- Fairchild KD, Singh IS, Patel S, et al. Hypothermia prolongs activation of NF-κΒ and augments generation of inflammatory cytokines. American Journal of Physiology-Cell Physiology. 2004;287:C422–C431.
- Clements JM, Casa DJ, Knight JC, et al. Ice-Water immersion and cold-water immersion provide similar cooling rates in runners with exercise-induced hyperthermia. J Athl Train. 2002;37:146–150.
- Proulx CI, Ducharme MB, Kenny GP. Effect of water temperature on cooling efficiency during hyperthermia in humans. J Appl Physiol. 2003;94:1317–1323. (1985).
- Peiffer JJ, Abbiss CR, Watson G, et al. Effect of cold-water immersion duration on body temperature and muscle function. J Sports Sci. 2009;27:987–993.
- Mittleman KD, Mekjavić IB. Effect of occluded venous return on core temperature during cold water immersion. J Appl Physiol (1985). 1988;65:2709–2713.
- Romet TT. Mechanism of afterdrop after cold water immersion. J Appl Physiol (1985). 1988;65:1535–1538.
- Park YR, Sultan MT, Park HJ, et al. NF-κB signaling is key in the wound healing processes of silk fibroin. Acta Biomater. 2018;67:183–195.
- Scheller J, Chalaris A, Schmidt-Arras D, et al. The pro- and anti-inflammatory properties of the cytokine interleukin-6. Biochim Biophys Acta. 2011;1813:878–888.
- Xing Z, Gauldie J, Cox G, et al. IL-6 is an antiinflammatory cytokine required for controlling local or systemic acute inflammatory responses. J Clin Invest. 1998;101:311–320.
- Severinsen MCK, Pedersen BK. Muscle-organ crosstalk: the emerging roles of Myokines. Endocr Rev. 2020;41:594–609.
- Prame Kumar K, Nicholls AJ, Wong CHY. Partners in crime: neutrophils and monocytes/macrophages in inflammation and disease. Cell Tissue Res. 2018;371:551–565.
- Dhabhar FS, Malarkey WB, Neri E, et al. Stress-induced redistribution of immune cells—from barracks to boulevards to battlefields: a tale of three hormones – Curt Richter Award Winner. Psychoneuroendocrinology. 2012;37:1345–1368.
- Cox JH, Ford WL. The migration of lymphocytes across specialized vascular endothelium: IV. prednisolone acts at several points on the recirculation pathways of lymphocytes. Cellular Immunology. 1982;66:407–422.
- Wine TM, Alper CM. Cytokine responses in the common cold and otitis media. Curr Allergy Asthma Rep. 2012;12:574–581.
- Janicki-Deverts D, Cohen S, Turner RB, et al. Basal salivary cortisol secretion and susceptibility to upper respiratory infection. Brain Behav Immun. 2016;53:255–261.
- Stephenson LA, Kolka MA. Thermoregulation in women. Exercise and Sport Sciences Reviews. 1993;21:231–262.
- Janssen I, Heymsfield SB, Wang ZM, et al. Skeletal muscle mass and distribution in 468 men and women aged 18-88 yr. J Appl Physiol. 2000;89:81–88. (1985).
- van Marken Lichtenbelt WD, Vanhommerig JW, Smulders NM, et al. Cold-activated brown adipose tissue in healthy men. N Engl J Med. 2009;360:1500–1508.
- Solianik R, Skurvydas A, Mickevičienė D, et al. Intermittent whole-body cold immersion induces similar thermal stress but different motor and cognitive responses between males and females. Cryobiology. 2014;69:323–332.
- Skurvydas A, Brazaitis M. Plyometric training does not affect central and peripheral muscle fatigue differently in prepubertal girls and boys. Pediatric Exercise Science. 2010;22:547–556.
- Kenney WL, Munce TA. Invited review: aging and human temperature regulation. J Appl Physiol (1985). 2003;95:2598–2603.
- Brazaitis M, Paulauskas H, Eimantas N, et al. Heat transfer and loss by whole-body hyperthermia during severe lower-body heating are impaired in healthy older men. Experimental Gerontology. 2017;2017:12–18.