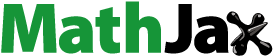
Abstract
Purpose
To investigate the design of an endoluminal deployable ultrasound applicator for delivering volumetric hyperthermia to deep tissue sites as a possible adjunct to radiation and chemotherapy.
Method
This study considers an ultrasound applicator consisting of two tubular transducers situated at the end of a catheter assembly, encased within a distensible conical shaped balloon-based reflector that redirects acoustic energy distally into the tissue. The applicator assembly can be inserted endoluminally or laparoscopically in a compact form and expanded after delivery to the target site. Comprehensive acoustic and biothermal simulations and parametric studies were employed in generalized 3D and patient-specific pancreatic head and body tumor models to characterize the acoustic performance and evaluate heating capabilities of the applicator by investigating the device at a range of operating frequencies, tissue acoustic and thermal properties, transducer configurations, power modulation, applicator positioning, and by analyzing the resultant 40, 41, and 43 °C isothermal volumes and penetration depth of the heating volume. Intensity distributions and volumetric temperature contours were calculated to define moderate hyperthermia boundaries.
Results
Parametric studies demonstrated the frequency selection to control volume and depth of therapeutic heating from 62 to 22 cm3 and 4 to 2.6 cm as frequency ranges from 1 MHz to 4.7 MHz, respectively. Width of the heating profile tracks closely with the aperture. Water cooling within the reflector balloon was effective in controlling temperature to 37 °C maximum within the luminal wall. Patient-specific studies indicated that applicators with extended OD in the range of 3.6–6.2 cm with 0.5–1 cm long and 1 cm OD transducers can heat volumes of 1.1–7 cm3, 3–26 cm3, and 3.3–37.4 cm3 of pancreatic body and head tumors above 43, 41, and 40 °C, respectively.
Conclusion
In silico studies demonstrated the feasibility of combining endoluminal ultrasound with an integrated expandable balloon reflector for delivering volumetric hyperthermia in regions adjacent to body lumens and cavities.
1. Introduction
Thermal therapy has been extensively investigated or used for treatment of cancers as an alternative to surgery. Compared to its counterparts such as radiofrequency (RF), laser, or microwave (MW), ultrasound delivers localized and spatially precise thermal therapy with a high degree of control over energy deposition and deeper penetration within the tissue. Mild hyperthermia (40–45 °C) is considered as an important modality for cancer treatment and as an adjunct therapy has shown enhancement of radiation and/or chemotherapy to increase response rates and overall survival [Citation1–4]. The various effects caused by hyperthermia on tumors can improve the delivery of systemically delivered drugs by increasing the tumor uptake of the bioavailable drug due to an increase in the blood flow [Citation5,Citation6], increase in the permeability of blood vessels and cell membranes [Citation7] with an increase in the size of endothelial pores [Citation8] which not only result in reduced interstitial pressure and hypoxia [Citation9,Citation10] but also ensures greater penetration and drug uptake [Citation11,Citation12] due to an increased permeability to drugs and increased liposomal extravasation [Citation13,Citation14]. Temperature sensitive liposomes are a significant advancement in targeted drug delivery mechanisms. These liposomes are loaded with anticancer drugs and are triggered to release by local application of heat within the hyperthermia regime (39–43 °C). Results of combining hyperthermia with temperature sensitive liposomes have been promising [Citation15–20]. Recent clinical trials have shown the efficacy of mild hyperthermia for drug delivery [Citation21–23]. While extracorporeal high-intensity focused ultrasound (HIFU) with mechanical or electronic scanning has been utilized to generate hyperthermia to deep target sites in combination with temperature sensitive liposomes, the depth, uniformity, and volume of hyperthermia achievable may be limited by the loss of gain and heterogeneity due to scanning speed. The reliance on commercial ablative device to deliver hyperthermia, lack of clinical ultrasound devices specific for inducing hyperthermia, and difficulty in monitoring tissue temperature are major hurdles in hyperthermia therapy in general, and notably for thermal-induced or triggered drug delivery.
Endoluminal ultrasound applicators offer a minimally invasive approach to deliver precision thermal therapy to regions adjacent to body lumen or cavities. While more invasive than extracorporeal HIFU, endoluminal ultrasound can provide better volumetric localization and can circumvent bone, gas, and bowels which impede the efficient delivery of ultrasound from extracorporeal HIFU that requires a clear acoustic window between the transducer and the target site [Citation24–30]. Various endoluminal or endorectal ultrasound applicator devices have been developed for ablation of liver [Citation31], pancreatic [Citation32,Citation33,Citation34], esophageal [Citation35,Citation36], or prostate [Citation37,Citation38] tumors. Endoluminal ultrasound applicators for thermal therapy of the pancreas through the GI wall under MR guidance have been investigated [Citation39,Citation40], however, they are limited in accessible areas and target depth (<2.5 cm) of delivered treatment due to constraints on the size of directional transducer that can be delivered through the esophagus. To facilitate the passage of the endoluminal devices through body lumens, the overall profile of the applicator is kept small which results in small effective radiating aperture, which, thus, may limit the heating volumes and reduce versatility to shape energy deposition patterns in accordance to the tumor volume or size. To account for this, cylindrical ultrasound transducers were combined with expandable balloon-based reflectors for generating focused or annular ablation patterns. Parabolic reflectors composed of inner water-filled and outer carbon dioxide filled balloons, surrounding ultrasound transducers, to reflect and redirect ultrasound energy and produce annular ablation pattern, were used for targeting pulmonary vein denervation [Citation40–43]. Adams et al. [Citation44] proposed a 1D cylindrical phased array integrated with an expandable balloon-based parabolic reflector offering tight focusing and electronic steering in depth. Hynynen [Citation45] used a conical 45° brass cone to reflect the ultrasound field from cylindrical transducers and redirect the acoustic energy in a forward propagation direction for radiation force measurements. Other investigations for endoluminal ablation have considered combining cylindrical ultrasound transducers within an expandable multi-compartment balloon with water, air, and perfluorocarbon liquid to reflect and redirect the acoustic energy forward through a larger effective aperture; thus, allowing for dynamic focusing in depth by mechanically varying the distal curvature of the compartment holding the perfluorocarbon fluid, as well as enhanced penetration [Citation46]. None of these studies have, however, investigated the generation and delivery of volumetric hyperthermia using an expandable deployable conical reflector.
The objective of this in silico study is, thus, to investigate the feasibility and performance metrics of an endoluminal ultrasound applicator configuration, which incorporates a low profile linear array of two tubular ultrasound transducers integrated within an expandable conical-shaped reflector balloon, for delivery of volumetric hyperthermia to deep organs such as pancreas. Three-dimensional acoustic and biothermal models were developed to calculate acoustic fields, power deposition patterns, and corresponding temperature distributions. An extensive set of parametric studies was performed by using temperature contours, iso-thermal volumes, and depth of temperature contours from the luminal wall, as performance metrics to assess applicator design schemes (transducer dimensions, balloon reflector geometry, frequency) and power delivery. Patient-specific models of pancreatic tumors and surrounding anatomy were developed based on segmentation of CT images, and comprehensive analysis were performed for a variety of potential target volumes and different applicator geometries to explore a range of clinical presentation. The simulation-based characterizations are used to devise delivery strategies and evaluate capability of the proposed applicator in achieving volumetric thermal coverage of pancreatic tumors while avoiding damage or thermal exposure to the surrounding healthy tissues.
2. Materials and methods
2.1. Endoluminal ultrasound hyperthermia applicator
Building upon the concepts shown feasible for redirecting acoustic energy from cylindrical transducers using parabolic [Citation40,Citation42,Citation44] and conical reflectors [Citation45,Citation46], the deployable ultrasound applicator investigated herein for hyperthermia delivery can similarly be inserted within a body lumen or laparoscopic port, and deployed adjacent to the target volume. Following the schema of , the cylindrical transducer source is surrounded by a multi-lumen balloon structure on the distal end of the device. The interior compartment hosting the tubular sources is filled with water and has a conical geometric profile along its transverse border when expanded. The outer compartment which couples with the transverse border of the interior balloon is filled with air forming a reflective surface. The angle of the reflector interface, in the conical geometry, with respect to the short axis of the catheter is which corresponds to a 44° reflector surface in the direction of beam propagation. After insertion and placement in close proximity to the target, the dual layer conical shaped balloon is inflated with water and thin air interface for reflecting and redirecting the ultrasound energy, generated by the tubular transducers, forward in an annular ring pattern through the coupling medium and luminal wall and propagate into the tissue.
Figure 1. Design schema for the deployable conical ultrasound hyperthermia applicator, consisting of two tubular ultrasound source transducers positioned at the distal end of the catheter, surrounded by a distensible two-compartment balloon, shown in (a) compact profile with the balloon in a collapsed state for device delivery, (b) expanded profile for thermal therapy after positioning at the target site, with the interior compartment filled with water and the outer thin compartment filled with air forming a reflective boundary that directs the ultrasound energy in the forward direction forming an annular beam pattern.
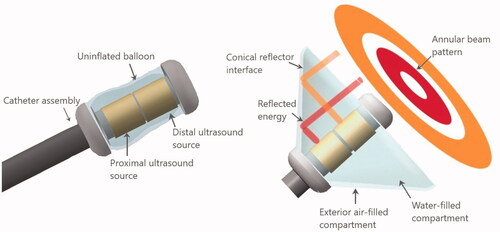
The input power of each cylindrical radiator can be adjusted independently to control the spatial power deposition into the tumor. Three applicator geometries were used in simulations, as listed in . All geometries consist of two tubular transducers centrally aligned along the distal tip of the applicator with a diameter of 10 mm and lengths of 10, 7, and 5 mm, respectively. The spacing between the two tubes is 1.5 mm. Two solid fixtures hold the tubes to the proximal and distal tip of the balloon. The height of the applicators, defined as the distance from the proximal to the distal end of the balloon, were 32, 23, and 19 mm. The dimensions of these applicators are shown in .
Table 1. Dimensions and configurations of the three applicator geometries modeled in this study.
2.2 Acoustic and biothermal modeling
A finite difference time-domain solver (FDTD) of the 3D linear acoustic pressure wave equation (LAPWE), Sim4life 5.2 (Zurich Med Tech AG, Zürich, Switzerland), was used to model acoustic wave generation by the applicator assembly and propagation through water and into the heterogenous tissue region. The formulation of the LAPWE used in Sim4Life is as follows:
(1)
(1)
where
is the density,
is the pressure,
is the sound speed,
is the time,
is the absorption coefficient, and
is the angular frequency. The FDTD solver accounts for reflection, refraction, diffraction, attenuation, and interference. The maximum element size of the voxels for FDTD acoustic simulation is set to λ/15 to ensure simulation accuracy, where λ is the wavelength.
To investigate temperature distributions achievable with the proposed applicator, a 3D bioheat transfer model of a multilayered generalized tissue model and patient specific models were employed. Temperature distributions resulting from the propagated acoustic intensity fields were simulated using the Pennes bioheat transfer equation [46]
(2)
(2)
where
(°C) is the tissue temperature,
(kg/m3) is the tissue density,
(J/kg/°C) is the specific heat capacity of the tissue,
(W/m/°C) is tissue thermal conductivity,
(kg/m3/s) is the blood perfusion rate,
(37 °C) is the capillary blood temperature,
(J/kg/°C) is the specific heat of blood,
(w/m3) is the acoustic power deposition in the tissue, which can be derived from the acoustic pressure field as
(3)
(3)
where
(Pa) is the complex pressure profile,
(W/m2) is the acoustic intensity and
(Np/m) is the acoustic absorption coefficient in tissue which was approximated as equal to the attenuation coefficient of the tissue assuming all the acoustic energy is absorbed locally. Intensity profiles can be calculated directly from the acoustic pressure in this equation, which is an approximation assuming plane wave propagation of the ultrasound field.
2.3 Generalized 3D model and parametric studies
Parametric studies were performed to investigate the capability of the deployable reflector applicator configuration to propagate acoustic energy through the luminal wall and into pancreatic parenchyma and tumor, and the feasibility of generating hyperthermia temperatures within pancreatic tumors. Acoustic intensity distributions generated by the assembly were simulated in a generalized 3D tissue model to determine the effects of frequency, attenuation, and perfusion on the heating volume and penetration depth from the luminal wall. The model consisted of layers of luminal wall, tumor, and pancreatic tissue. The dimensions of the model were 60 × 60 × 75 mm. The expanded applicator assembly was positioned adjacent to and forward projecting through the 2 mm thick luminal wall. The normal pancreatic tissue–tumor boundary was 5 mm from the luminal wall and the tumor is 30 mm thick. The model geometry of the applicator assembly and tissue compartments is shown in . The balloon–luminal wall interface was cooled with internal flow of water and modeled as a convective heat flux boundary condition as described below.
Figure 2. Cross-sectional slice of the 3D generalized tissue model and conical applicator as applied for parametric acoustic and thermal studies of hyperthermia delivery to pancreatic tumors.

Heterogeneous tissue properties and perfusion values of stomach, luminal wall, duodenum, pancreas, and tumor are shown in . Pancreatic tumors were modeled with the same properties as normal pancreatic parenchyma, but 1.25 times greater attenuation and 0.5 times perfusion to approximate effects of tumor specific desmoplastic stroma. The range of values of attenuation applied as a function of frequency, as reported in literature [Citation46,Citation48,Citation49], was 4.37 f1.2 Np/m–11.9 f°.78 Np/m, where f is the frequency in MHz. Perfusion values were varied in the range of 0.5–5 kg/m3/s, with a nominal value of 2.2 kg/m3/s. Previous studies have shown that perfusion level in tumor is between 48% and 60% of the normal tissue [Citation50], perfusion rate of 50% was, thus, used for the tumor. Three different attenuation levels (5.57 Np/m/MHz, 7.33 Np/m/MHz, and 9.09 Np/m/MHz spanning the range for healthy tissue and 1.25 times these values for pancreatic tumor), and three different values of the rate of blood perfusion levels (0.5, 2.2, 5 kg/m3/s and 0.25, 1.1, 2.5 kg/m3/s for normal pancreatic tissue and pancreatic tumor, respectively) were used to encompass the entire range of the tissue properties reported in the literature. Volumes of 3D contours of heating and the lateral extent of the heating contours were calculated for three temperature levels 43 °C, 41 °C, and 40 °C and termed as
and
respectively. Penetration depth to the start of therapeutic zone from the luminal wall (
) and the maximum extent of therapeutic zone (
) were also calculated. Temperature profiles were calculated with the Sim4Life thermal solver with Dirichlet boundary conditions set to 37 °C at the tissue extremities. Cooling water flow within the applicator provides acoustic coupling between the transducer and luminal wall and the thermal penetration can also be controlled by changing temperature of the cooling water flow. Convective heat loss due to the cooling flow is modeled by convective boundary condition at the luminal wall with heat flux given by the following equation:
(4)
(4)
where
is the outward normal vector, k is the thermal conductivity (W/m/°C),
is the cooling flow temperature, and
is the luminal wall temperature. The convective heat transfer coefficient
is set to 500 w/m2/°C and the initial value of
is set to 37 °C.
Table 2. Material properties used in acoustic and biothermal modeling [Citation33,Citation34,Citation47].
For parametric studies, the effects of changing transducer frequency, attenuation coefficient, perfusion rate and water-cooling temperature on the volume and dimensions of 40, 41, and 43 °C contours were determined. The maximum and the minimum penetration depth of the iso-temperature contours from the outer surface of the luminal wall in the central plane were also calculated. To facilitate direct comparison across a broad range of tissue parameters, the input surface intensities of each tubular transducer were optimized specific to each case to achieve uniform and broad temperature distribution in the tissue with a maximum value of 45 °C. A temperature based constrained optimization was performed to calculate the applied power levels which would maximize the hyperthermia treatment volume above 40 °C such that the maximum temperature in the treatment volume does not exceed 45 °C. A simplified objective function was used
(5)
(5)
The objective function was evaluated at
points in the heating volume and power values
on both the transducers were modulated to achieve temperature of 45 °C at the control points such that the maximum temperature does not exceed 45 °C and the minimum temperature stays above 40 °C inside the tumor.
2.4 Patient-specific anatomical models
To further evaluate the feasibility of the endoluminal ultrasound applicator for hyperthermia treatment of pancreatic tumors in a complex anatomical setting, patient specific models with anatomical structures were generated. The 3D models of patient anatomy were generated from CT images of patients with pancreatic cancer by segmenting the images in Mimics (Materialise, Leuven, Belgium) and then generating 3D surface meshes of the relevant anatomical structures including stomach, pancreas, tumor, duodenum, esophagus, luminal wall, major veins, and arteries surrounding and within the pancreas. The 3D anatomical structures were then imported as STL files into Sim4Life. Similar to the parametric studies, acoustic simulations to calculate acoustic pressure and intensity distributions were calculated using the 3D FDTD acoustic solver of Sim4Life based on linear acoustic pressure wave and adaptive rectilinear meshes with inhomogeneous perfectly matched layer (PML) boundary conditions. The dimensions of the voxels for acoustic simulations were set at λ/15. The transducers were excited with 3.4 MHz frequency and constant input power was selected for 45 °C rise to obtain temperature distributions at steady state. The attenuation coefficient of pancreas and pancreatic tumor were selected as the maximum values from . Localized cooling effects of major blood vessels were modeled using heat flux boundary conditions at the surface of the blood vessels, with an ambient blood temperature of 37 °C at the surface of the vessels and a heat transfer coefficient h set to 554 w/m2/°C [Citation51,Citation52]. Ducts were modeled with the properties of static fluid. Due to the uncertainty in the reported tissue properties and the likely variation in the tissue properties and perfusion rate during the heating, the applied perfusion rate and the attenuation was varied in the range of maximum and minimum values as shown in .
Four broadly diverse cases of pancreatic tumors were studied, with details shown in , to consider the feasibility of hyperthermia treatment with the endoluminal applicator. Model 1 includes an advanced case of pancreatic ductal adenocarcinoma with a large body tumor with ∼5.5 cm diameter and a volume of ∼32 cm3. Model 2 includes a smaller tumor in the body of the pancreas with 5 cm diameter and a volume of ∼18 cm3. Model 3 includes a smaller tumor in the body of the pancreas with ∼3 cm diameter and volume of ∼11 cm3 and model 4 comprises of a small tumor in the head of the pancreas and closed to duodenum with a diameter of ∼2 cm and volume of ∼3 cm3. The different applicator geometries described earlier were selected for each case based on the size and positioning of the tumor within the pancreas. Applicator geometry I was used for heating the large tumor of model I due to its maximum diameter of 5.5 cm, geometry II was used to heat the tumor in model II due to its relatively smaller width, whereas, geometry III was selected for delivering hyperthermia treatment to models III and IV due to their small sizes and the ease of positioning geometry III in the pylorus region to access the small head tumor.
Table 3. Anatomical characteristics of patient specific models with pancreatic tumors and corresponding deployable ultrasound applicator geometries from .
3. Results
3.1. Acoustic beam profiles
The propagation of acoustic energy produced from the linear array of two transducers and reflected through the applicator coupling surface to the heterogenous tissue medium with nominal attenuation and perfusion values is demonstrated in , comparing longitudinal intensity distributions produced by a conical applicator at 3.4 MHz with (a) an equal input surface intensity 2.44 w/cm2 on each of the tubular transducers, and compared to (b) a distal element surface intensity increased by a factor of 4 with respect to the proximal value, to compensate for the geometric loss differences due to the larger surface area of the outer annular ring. The acoustic intensity profile across the applicator surface can be varied by adjusting the relative levels of input power to the transducers and can broaden the intensity profile by delivering more power to the distal element. Slightly divergent annular patterns associated with each transducer segment can be seen, with axial energy deposition midline due to coherent field overlap.
Figure 3. Intensity distributions (W/cm2) projected from the deployable applicator into the generalized tissue model, (a) with equal input surface intensity (2.44 W/cm2) for both proximal and distal transducers, and (b) power weighting with input power at the distal element four times than the proximal transducer to compensate for the larger geometric intensity decrease inherent to the outer annular distribution. Z-axis represents depth or axial distance from the distal surface of the applicator. Applicator parameters were: 3.4 MHz, 46° conical balloon with 6 cm aperture, and two 10 mm long × 10 mm OD transducers.
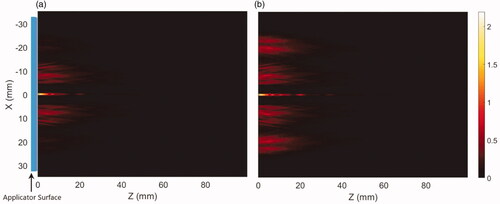
3.2. Generalized 3D model – parametric studies
The parametric studies in the generalized 3D model were performed with the applicator geometry I with dimensions as shown in . The characteristic temperature contours produced by the applicator in the generalized tissue model for 3.4 MHz and nominal attenuation and perfusion levels are shown in . The transverse planes show the axisymmetric annular pattern corresponding to the tubular transducers that mimic a concentric ring array. The 43 °C peaks are localized in ring shapes and extend up to 1 cm deep in the tumor, whereas the 40 °C and 41 °C contours further extend in the tumor. Since the acoustic intensity delivered by the cylindrical transducer is inversely proportional to the effective radiated surface area, the width of the temperature contours and, hence, the heating volume was observed to increase by increasing the input power of the distal element which acts as the outermost ring in a concentric ring array.
Figure 4. Cross-sectional hyperthermia temperature distributions in the generalized 3D tissue/tumor model for the ultrasound applicator geometry I (6 cm aperture, two 1 cm OD transducers) with (a) equal input powers at both tubular transducers and, (b) modulated input power to broaden the heat distribution (distal element powered 3.4 times higher than proximal), at 3.4 MHz and nominal perfusion and attenuation values. The red, yellow and cyan contours are 43, 41 and 40 °C, respectively. The blue line in the axial and the circle on the transverse planes represent the outer surface projections of the applicator.
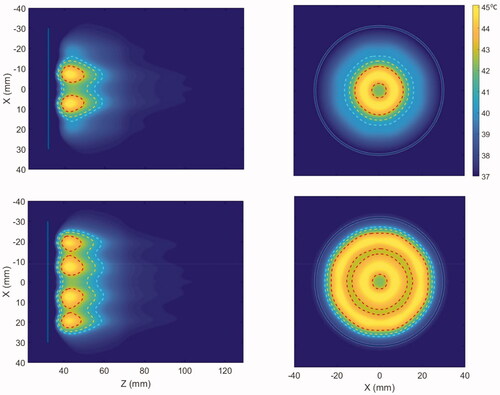
The penetration depth and heating patterns for various frequencies are shown in . Large volumetric coverage of heating and deeper and broader penetration is achieved with lower frequencies due to low attenuation and absorption at such frequencies. The effect of operating frequency on hyperthermia heating volume and the penetration depth of the heating contours from the luminal wall is further elaborated in . The heating volume of 40, 41, and 43 °C temperature contours ranged from 33 to 60 cm3, 24 to 48 cm3, and 7 to 18 cm3, whereas the depth from the luminal wall varied from 24 to 37 mm, 20 to 34 mm, and 9 to 25 mm as frequency decreased. The input surface intensity on both the transducers were modulated to achieve uniform volumetric coverage in the tumor domain such that peak temperature in each annular ring does not exceed 45 °C. The required input power at both the transducers is reduced with increasing frequency, as the ultrasound energy at higher frequencies exhibit higher absorption and power deposition in the tissue. The input surface intensities varied from 1.1 to 2 W/cm2 and 3.6 to 7.5 W/cm2 for both the proximal and distal elements in the frequency range of 1–4.7 MHz, where the lowest values of the input power correspond to the highest frequency as shown in . At 3.4 MHz, 40 °C temperature contour had a heating volume of 41 cm3 and a penetration depth of 31 mm from the luminal wall and was chosen as the frequency of operation in the rest of simulations.
Figure 5. Effect of the operating frequency on hyperthermia temperature distribution in the generalized tissue model for (a) 1 MHz, (b) 1.5 MHz, (c) 2.45 MHz, (d) 3.4 MHz, and (e) 4.7 MHz, with applicator geometry I (6 cm aperture, two 1 cm OD × 1 cm long transducers). The red and cyan contours are 43 and 40 °C, respectively. Nominal values of attenuation and perfusion level were used. The cooling flow water temperature was 20 °C.
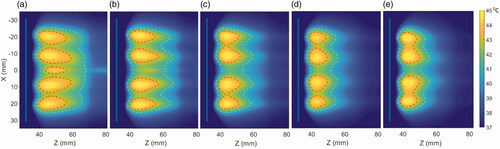
Figure 6. Effect of the operating frequency on iso-thermal heating volumes and the average penetration depth of the heating contours from the luminal wall in the central axial plane. The lines indicate the effect on changing heating volume for 43 °C (red), 41 °C (black), and 40 °C (blue) iso-temperature volumes, whereas the values of the minimum and maximum extent of heating contours for 43 °C (yellow), 41 °C (green), and 40 °C (purple) are overlaid and shown with the right y-axis.
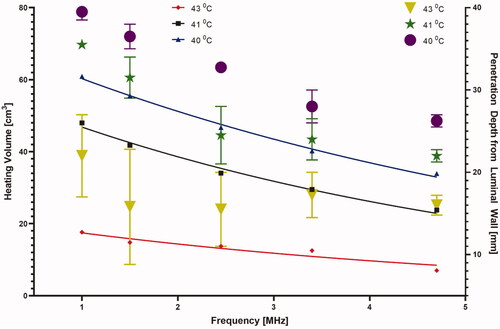
Figure 7. The required input surface intensity at both proximal and distal transducer segments as a function of (a) frequency and (b) attenuation and perfusion, for optimal settings of 45 °C maximum and uniform hyperthermia, p1, p2, and p3 correspond to perfusion values of 0.5, 2.5 and 5 kg/m3/s respectively.
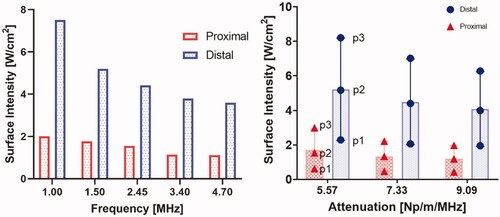
The effect of different tissue attenuation values and perfusion levels on the heating performance of the applicator operating at a nominal frequency of 3.4 MHz is summarized in , showing the change in the heating volume of 43 °C, 41 °C, and 40 °C contours. The heating volumes for these configurations ranged from 33 to 63 cm3 at 40 °C, 23.3 to 49.3 cm3 at 41 °C, and 7.6 to 27 cm3 at 43 °C. The highest heating volume was observed at the lowest attenuation and perfusion levels. There was a considerable variation in the required input power at both the proximal and distal elements for these values of attenuation and perfusion. As expected, and shown in , the required input surface intensity was lower for higher attenuation and lower perfusion. Overall, the required input surface intensity ranged from 0.43 to 2.59 W/cm2 and 1.95 to 8.2 W/cm2 for different attenuation and perfusion values at both the proximal and distal tubes, respectively. The penetration depth of the therapeutic zone from luminal wall for various configurations is shown in . The greatest penetration depth from the luminal wall is observed for the lowest values of attenuation and perfusion rate as expected. Penetration depth in the central axial plane varied from 21.5 to 39 mm, 13 to 36 mm, and 10 to 29 mm for 40 °C, 41 °C, and 43 °C contours, respectively. Lower perfusion level and lower attenuation results in maximum depth of the heating contours in the tissue, albeit with an increased applied power requirement (). Since the heating contours are not uniform along the depth, maximum and minimum values of the contours are calculated. It can be seen in that high perfusion results in higher deviation from the base value signifying more heterogeneity. The depth of the heating contours is largely uniform at lower perfusion values and is less uniform along the depth when the value of perfusion rate increases.
Figure 8. Effect of pancreatic tissue attenuation coefficient and perfusion rate on hyperthermic heating volume as determined by 3D contours > 43 °C (red), > 41 °C (black) and > 40 °C (blue) at 3.4 MHz frequency. Perfusion levels are 0.5, 2.5 and 5 kg/m3/s in the tissue and 50% of these values in the tumor, T1, T2, and T3 corresponds to temperatures at 40 °C, 41 °C, and 43 °C, respectively.
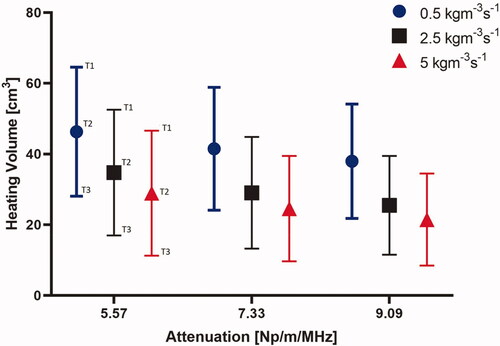
Figure 9. Effect of pancreatic tissue attenuation coefficient and perfusion rate on the maximum penetration depth measured from the outer surface of the luminal wall for geometry I (6 cm aperture, two 1 cm OD × 1 cm long transducers) for: (a) 2D contours in the central plane for T > 43 °C, T > 41 °C, and T > 40 °C and for attenuation values of 9.09 Np/m/MHz (left), 7.33 Np/m/MHz (middle), and 5.58 Np/m/MHz (right), and (b) 2D contours in the central plane > 40 °C (left), > 41 °C (middle), and >43 °C (right). Perfusion levels are 0.5, 2.5 and 5 kg/m3/s in the tissue and 50% of these values in the tumor.
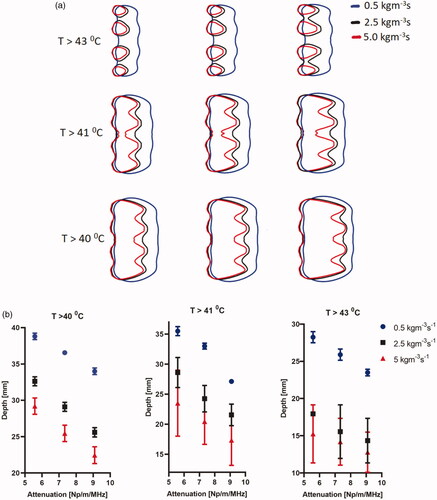
3.3 Patient-specific models
More complex acoustic and thermal simulations were performed in four patient specific models using one of the three applicator geometries, selected depending on the size and position of the tumor within the pancreas () to afford positioning and thermal coverage.
As shown in , model 1 describes a large tumor with dimensions 39 × 55 × 35 mm3 and volume 32.64 cm3 positioned in body of the pancreas adjacent to stomach lumen. The applicator geometry I (two tubes 1 cm OD × 1 cm long, 3.4 MHz, 6 cm OD) was selected to heat this tumor due to its large size. shows 3D model of the patient anatomy used in the simulations and the resulting temperature distributions. The temperature of the tumor was elevated above 40 °C at steady state. It was observed that 7–26 cm3 and 16–37 cm3 of the tumor can be heated at the highest and lowest perfusion rates, with 18 and 31 cm3 of tumor heated at the nominal perfusion level, above 41 °C and 40 °C, respectively (see ). The extremities of maximum and minimum penetration depth of the 40 °C heating contour ranged from 1.25 to 5 mm and 35 to 36.5 mm from the luminal wall, whereas its width varied from 49 to 53 mm with varying perfusion rate.
Figure 10. (a) 3D patient specific model and biothermal simulation for endoluminal hyperthermia treatment of a large pancreatic body tumor and surrounding anatomy (model 1): (a) deployed ultrasound applicator (A) (geometry I: two 1 cm OD × 1 cm long transducers, 3.4 MHz, 6 cm balloon aperture) is positioned in the stomach (S) adjacent to lumen wall, and energy directed toward the pancreatic tumor, (b) applicator positioning in the stomach and steady-state temperature distribution overlayed on the planning CT image, (c) detailed temperature distributions with labeled 40 and 43 °C contours across a central axial plane, with both tumor (T) and pancreas (P) delineated; (d) temperature distribution across a transverse plane at 10 mm depth from the applicator surface, with an overlay of the projected tumor boundary; D: duodenum; SMA: superior mesenteric artery; SMV: superior mesenteric vein; SV: splenic vein.
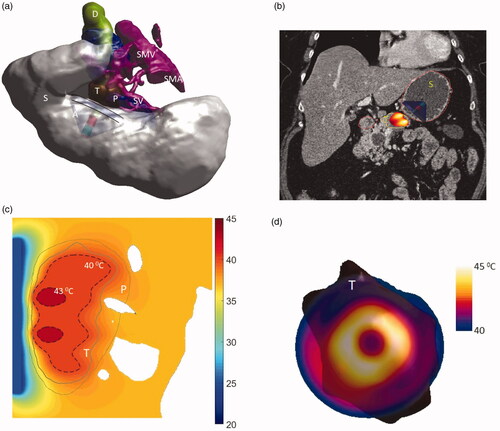
Table 4. Parameters and corresponding thermal dosimetry described for endoluminal ultrasound hyperthermia simulations of four patient-specific patient anatomies and three applicator geometries.
Model 2 describes a small tumor in the body of the pancreas and in close proximity to the stomach lumen, and applicator geometry II was selected for heating this tumor. The applicator had two tubes each 7 mm long and radii of 5 mm and had an overall balloon diameter of 4.6 cm when inflated. The applicator was positioned close to the lumen and operated at 3.4 MHz. This model was simulated with two different attenuation and three perfusion values to encompass the entire range of tissue properties shown in . The resultant temperature distributions for this case can be seen in and . Temperature of the entire tumor was elevated above 40 °C at steady state. It was observed that with high attenuation, 26–100% and 57–100% tumor can be heated at highest and lowest perfusion rates, whereas, with low attenuation, the entire tumor can be heated above 41 °C and 40 °C, respectively. At nominal perfusion rate, 93% and 100% of the tumor can be heated above 41 °C with low and high attenuation, respectively. The maximum and the minimum penetration depth of the 40 °C contour from the luminal wall varied from 3 to 3.5 mm and 22 to 38 mm for high attenuation, whereas, at low attenuation, the maximum and the minimum penetration depth were in the range of 1–5 mm and 32.5–44 mm, respectively.
Figure 11. Simulated temperature distributions of endoluminal ultrasound hyperthermia directed at a pancreatic tumor (case II medium body) using applicator geometry II (two 1 cm OD × 0.7 cm long transducers, 3.4 MHz, 4.6 cm balloon aperture): (a) temperature distribution with highlighted 40 and 43 °C contours across a central axial plane, with both tumor (T) and pancreas (P) delineated; (b) Temperature distribution across a central axial plane and a transverse plane at a depth of 11 mm from aperture surface overlaid with model anatomy; (c) 43 °C iso-temperature volume inside the target; and (d) 40 °C iso-temperature volume inside the target. P: pancreas, S: stomach, T: tumor.
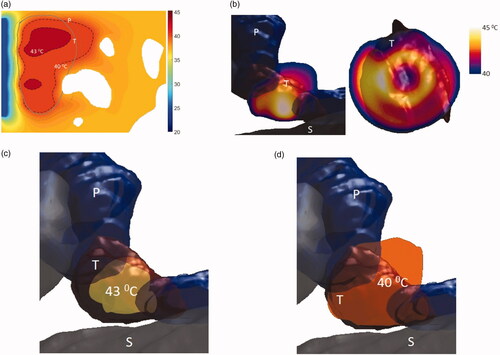
Figure 12. Simulated temperature distributions of endoluminal ultrasound hyperthermia directed at a pancreatic tumor (case III small body) using applicator geometry III (two 1 cm OD × 0.5 cm long transducers, 3.4 MHz, 3.6 cm balloon aperture): (a) temperature distribution with highlighted 40 and 43 °C contours across a central axial plane, with both tumor (T) and pancreas (P) delineated, (b) Temperature distribution across a central axial plane and a transverse plane at a depth of 6 mm from aperture surface overlaid with model anatomy, (c) 43 °C iso-temperature volume inside the target, and (d) 40 °C iso-temperature volume inside the target. P: pancreas, S: stomach, T: tumor.
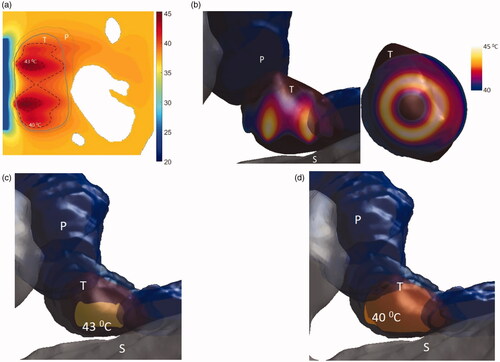
Model 3 describes a smaller tumor in the body of the pancreas, and applicator geometry III was selected for heating this tumor due to its relatively small size. The applicator has two tubes each 5 mm long and radii of 5 mm and an overall balloon aperture diameter of 3.6 cm. The applicator was positioned close to the lumen such that the tumor was 4 mm away from the distal surface of the applicator. This model was also simulated with maximum and minimum attenuation values and three perfusion values from . It was seen that with high attenuation, the volumes of the 41 °C and 40 °C contours ranged from 3.3 to 7.4 cm3 and 6.3 to10.3 cm3, whereas, at low attenuation, the heated volumes were 5.3–9 and 9.8–12.3, respectively. At nominal perfusion rate, the temperature of 84–100% of the tumor was elevated above 40 °C with minimum and maximum attenuation values. At the nominal perfusion rate, the minimum and maximum penetration depth from the luminal wall was 1 mm and 17 mm for high attenuation and 1 mm and 31.5 mm for low attenuation values, respectively. The width of the 40 °C heating contour was 34 mm and 39 mm at nominal perfusion and maximum and minimum attenuation values, encompassing the entire tumor volume. The stomach lumen was not heated and the 43 °C contour was at least 2 mm away from the outer surface of the stomach ().
Model 4 describes a small tumor in the head of the pancreas, with applicator geometry III selected due to the smaller size of the tumor, its proximity with duodenum and location of the tumor which is close to the pylorus, thus, suitable for smaller applicator to easily fit in. It was seen that the entire volume of tumor could be heated above 41 °C at steady state with 45% of tumor heated above 43 °C ( and ). The extremities of the depth of the 40 °C contours from the luminal wall are 21 and 1 mm, respectively. Due to the small dimensions of the tumor, two approaches were used to calculate heat distribution within the tumor. First, both the proximal and distal elements were excited equally and a broader than tumor heating distribution was observed. For this case, the heating was localized within the tumor by turning off the distal element. Whereas the depths of the temperature contours were same for both cases, the breadth varied significantly as can be seen in and .
Figure 13. 3D patient-specific model and biothermal simulation for endoluminal hyperthermia treatment of a small pancreatic head tumor and surrounding anatomy (model IV): (a) deployed ultrasound applicator (A) (geometry III: two 1 cm OD × 0.5 cm long transducers, 3.4 MHz, 3.6 cm balloon aperture) is positioned in the pylorus stomach (S) adjacent to lumen wall and close to the pancreas head, and energy directed toward the pancreatic tumor, (b) temperature distribution across a transverse plane at a depth of 6 mm from aperture surface overlaid with model anatomy, when both the transducers are active (left) and when only the proximal transducer is active (right), (c) detailed temperature distributions with labeled 40 °C and 43 °C contours across a central axial plane, with both tumor (T) and pancreas (P) delineated, when both the transducers are active (left) and when only the proximal transducer is active (right). D: duodenum; SMA: superior mesenteric artery; SMV: superior mesenteric vein; SV: splenic vein.
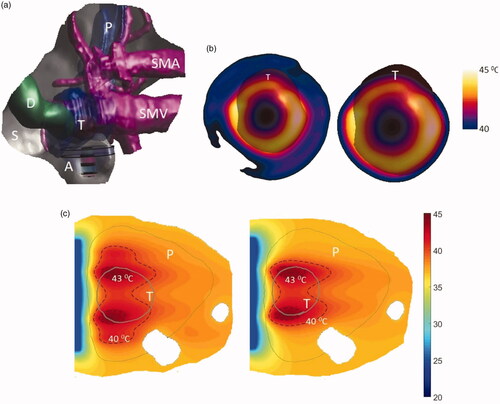
4. Discussion
In this study, a simulation framework was implemented to investigate the general performance and feasibility metrics of a deployable endoluminal ultrasound applicator configuration that can be delivered endoluminally or laparoscopically in a compact form, and once in position can be distended near the target for delivery of hyperthermia. The applicator consists of two transducer elements enclosed with-in a fixed shape balloon and reflector surface that expands once deployed to redirect the ultrasound energy forward, and, thus, provides a large effective aperture for broader hyperthermia coverage. The reflector surface of the balloon has a conical geometry, where the angle between the interface and the long axis of the catheter is 44°, thus, all reflected acoustic energy is collimated along the long catheter axis with minimal focusing of the energy in the tissue. The deployed applicator mimics a lower order annular ring array similarly used for extracorporeal HIFU [Citation53,Citation54] or open intraoperative hyperthermia [Citation55], and produces forward projected concentric annular zones of energy deposition, with each defined by either the distal or proximal transducer. Over a range of parameters and patient specific anatomy, these deployable forward firing ultrasound applicators have potential to deliver volumetric and penetrating hyperthermia patterns to tumor regions greater than 30 cm3 from within the stomach. Through selection of applicator and balloon dimensions and operating frequency, heating volumes up to 63 cm3 and 3.6 cm penetration depth, and up to 5.5 cm width of the therapeutic heating zone, can be achieved while limiting heating to the intervening GI wall. Delivery of local deep hyperthermia through this minimally invasive approach could provide the means of an effective adjunct for augmenting drug delivery, chemotherapy, and perhaps radiation to deep organs such as pancreas.
Due to variation in the size, depth, and location of pancreatic tumors, simulations with varying applicator geometries incorporating different patient anatomy models illustrated the feasibility of delivering hyperthermia across different tumor sizes and positions in the pancreas. As reported in the literature for endoscopic ultrasound imaging [Citation56–58] and confirmed in this study, the tumors in the body and tail of the pancreas can be accessed from the body of the stomach, whereas manipulating the stomach wall and positioning the applicator toward the pylorus would provide access to regions within the head of the pancreas. By selection of device balloon diameter and careful consideration of the applicator dimensions and frequency, depth of treatment from the luminal wall as well as dimensions of the therapeutic zone encompassing an effective margin around the tumor can be estimated a priori. A 3.4 MHz, 4.5–6 cm extended diameter balloon can be deployed and positioned within in the body of the stomach or proximal stomach to deliver hyperthermia to the pancreatic body and tail lesions at a maximum depth of 2–4 cm from the stomach wall, heat tumor regions up to 6 cm in diameter and avoid propagating energy and heating of bowel and sensitive structures distally. A smaller balloon with extended diameter of 3–3.6 cm could be more suitable to target head and neck lesions from distal stomach close to the pylorus. In cases with deeper or more extensive tumor infiltration within the pancreatic head and body, lower frequencies close to 1 MHz devices could be effective up to 4–6 cm depth; although possible limitations for low frequency implementation may be inadequate power delivery due to absolute limits on acoustical power on the distal element, especially for low regions of absorption and high blood flow.
To more completely assess applicator performance in silico, the uncertainty of specific tissue properties that dominate the achievable temperature distributions can be incorporated. The blood perfusion acts as a heat sink and can dynamically change during therapy and can considerably impact temperature heterogeneity and depth of heating; similarly, attenuation values strongly determine the penetration and magnitude of the acoustic power deposition and subsequent heating pattern. In consideration of the studies herein, the nominal values of blood perfusion and acoustic attenuation were based off average measurements obtained from the literature and inference. Given the uncertainties in these tissue properties and variations that are possible, simulations bracketed the anticipated range of perfusion () and attenuation (
) values. At the lower end of the perfusion rate, the volume of tumor heated above 40 °C and the maximum depth from the lumen wall for all the models were in the range of
and
respectively, whereas, at the highest perfusion rate, the heated tumor volume and the penetration depth were
and
respectively. For decreased attenuation at the lowest values, increases in heated volume and penetration depth are expected, as simulation results using model 2 suggested a
increase in the heated tumor volume and a further 10 mm extension of the 41 °C contour from the luminal wall. These results are in good agreement with the parametric studies and suggest that the applicator can deliver volumetric hyperthermia to large portions of tumor under dynamic or unknown tissue properties, with expected reduction in penetration due to increased perfusion and attenuation as commonly encountered in ultrasound hyperthermia. Though the tumors shown are heated in entirety above 40–41 °C which is an indication of its potential efficacy toward augmenting drug delivery, only portions of tumors are heated above 43 °C. This can be overcome by further optimizing and modulating the input power of the distal element thus increasing the temperature in the peripheries of the heated volume, or if tolerated increasing the maximum temperature. Another possible limitation is that the depth of heating volume is just short of 4 cm, which is adequate and more suitable for targets in pancreas, however, the depth and volume of therapeutic zone can be enhanced by lowering the frequency for hyperthermia delivery in other deep organs. It is important to note that these findings are obtained using simulations based upon approximations and estimations of tissue properties as common practice, and thus provide useful trends and general performance measures, but not precise treatment planning and verification.
Verification of placement of the applicator is important to ensure that energy is delivered precisely into and encompassing the target tumor. Image-guidance techniques such as ultrasound or magnetic resonance imaging (MRI) can be utilized for accurate positioning and placement of the endoluminal applicator. Although invasive, temperature sensors embedded within a flexible catheter could be positioned within the treatment region and placed at various distances from the applicator to monitor the temperature profile. Ultrasound image-guidance has previously been used for placement of catheter-based ultrasound probes. US imaging can be combined with EM tracking to co-register applicator position with the anatomy and to accurately position the applicator [Citation59,Citation60]. An ultrasound imaging array could also be embedded at the distal end of the applicator for target planning and image guidance, or similarly the deployable device can be integrated to an endoscopic ultrasound system [Citation33]. The applicator assembly could also be compatible with MRI for target localization and therapy monitoring. Advances in MR temperature monitoring can provide real-time means to monitor temperature, feedback control, and accommodate respiratory motion [Citation61–65]. MRTI is integrated in extracorporeal [Citation66,Citation67], transurethral [Citation37,Citation38,Citation68], interstitial [Citation61,Citation69–73,Citation74], and EM commercial systems [Citation75,Citation76] for treating sites such as prostate, pancreas, cervix, bone, and other deep tumors. The development of MR compatible endoluminal devices and integrating with MR thermometry has been shown as a promising technique for delivering accurate thermal therapy to deep organs under MR guidance [Citation32,Citation35,Citation77].
The delivery of volumetric and localized hyperthermia to deep targets such as the pancreas is difficult. Deep regional hyperthermia systems based upon extracorporeal electromagnetic or RF capacitive applicators generate large volumes of heating to the abdomen which are not isolated to a specific target zone [Citation78,Citation79]. MRI and US guided extracorporeal focused ultrasound systems have capabilities for improved spatial localization of hyperthermia, as demonstrated in clinical and pre-clinical studies [Citation16–18,Citation22,Citation23,Citation80–83]; however, difficulties defining adequate acoustic windows and reduced target volume to depth ratios limit the overall access to the pancreas and treatable target dimensions. In this setting, the deployable ultrasound applicator could provide enhanced volumetric heating of the pancreas with spatial control of power deposition from within the stomach. By positioning in close proximity to the target, performance is less affected by the presence of strong aberrating structures or obstructions and can selectively direct the heating volume to a specified target zone within the pancreas while avoiding damage to other nearby tissues. Moreover, in consideration of target zones larger than the applicator aperture, the device can be repositioned to abut therapeutic heating zones in a sequential manner to cover a large surface area and volume, which is a common practice in clinical hyperthermia. Further investigations should be undertaken to investigate additional potential applications for the proposed applicator design. The transducer geometry can be modified to tailor the need of the application under treatment. The diameter and length of the tubular transducers, the diameter of conical reflector, the spacing between the tubes and the reflector, and the frequency of operation can be adjusted for not only localized heating of the pancreatic tumors from within the stomach, but other sites such as intraluminal treatment of bladder cancer, and laparoscopic treatment of deep organs such as liver and kidney. The shape and size of the annular heating pattern can be spatially controlled by enhancing the applicator assembly to a 2D cylindrical array, with more rings and subdivisions along the angular extent, each with separate power control to offer better localization and selectivity of the heating patterns.
As device development and clinical investigations progress, it may be possible to deliver hyperthermia with these deployable applicators as an adjunct to a chemotherapy regimen such as conventional FOLFIRNOX [Citation20,Citation84] or immunotherapy [Citation1,Citation2,Citation85,Citation86]. As supported by many key studies, the localized heat can impact the blood flow and permeability of desmoplastic tumors [Citation5–7,Citation10,Citation87], thereby increasing drug uptake [Citation88] and activity [Citation11,Citation89], or directly targeting release of thermally sensitive liposomes [Citation16–18,Citation20–22,Citation80,Citation81] or nanoparticles [Citation13,Citation14]. Strong experimental evidence has also shown that mild hyperthermia has the potential to increase antigen uptake, enhances the expression of heat shock proteins and T cell stimulatory capacity of dendritic cells thereby improving the anti-tumor immune response [Citation90,Citation91]. The potential for using these deployable endoluminal ultrasound applicators for delivering more targeted volumetric hyperthermia with greater thermal doses compared to what is achievable with conventional extracorporeal deep hyperthermia systems, can provide motivation to implement this minimally invasive approach. As envisioned, single procedures in sync with the administration of targeted or thermally released drug may provide a significant therapeutic enhancement, as well as weekly or monthly procedures as sequential to augment chemotherapy regimens.
5. Conclusion
Parametric and patient-specific bioacoustic simulations have demonstrated the feasibility of generating volumetric hyperthermia distributions from endoluminal ultrasound applicators with an integrated deployable reflector balloon. In consideration of targeting pancreatic tumors from within the stomach, this study has shown that temperatures between 40 °C and 45 °C can be delivered out to 4 cm into the pancreas sustaining up to 60 cm3 of target tissue. Three specific applicator transducer configurations, ranging from 3.6 cm to 6.2 cm OD expanded apertures, were employed for hyperthermia treatment of body and head tumor models. 3D acoustic and thermal simulations across four models studied herein demonstrated the feasibility of heating 49–100% and 84–100% of tumors with varying sizes above 41 °C and 40 °C, respectively. Preliminary insight on performance characteristics for the transducer geometry, appropriate frequency, applicator positioning, and treatment parameters were obtained from thermal simulations for treatment of pancreatic head and body tumors. Further investigation and additional development are warranted for the device design and optimize delivery techniques for a range of applications.
Disclosure statement
No potential conflict of interest was reported by the author(s).
Additional information
Funding
References
- Datta NR, Grobholz R, Puric E, et al. Enhanced tumour regression in a patient of liposarcoma treated with radiotherapy and hyperthermia: hint for dynamic immunomodulation by hyperthermia. Int J Hyperthermia. 2015;31(5):574–577.
- Hurwitz MD. Hyperthermia and immunotherapy: clinical opportunities. Int J Hyperthermia. 2019;36(Suppl 1):4–9.
- Wust P, Hildebrandt B, Sreenivasa G, et al. Hyperthermia in combined treatment of cancer. Lancet Oncol. 2002;3(8):487–497.
- Van Der Zee J. Heating the patient: a promising approach? Ann Oncol. 2002;13(8):1173–1184.
- Shakil A, Osborn JL, Song CW. Changes in oxygenation status and blood flow in a rat tumor model by mild temperature hyperthermia. Int J Radiat Oncol Biol Phys. 1999;43(4):859–865.
- Zhu D, Lu W, Weng Y, et al. Monitoring thermal-induced changes in tumor blood flow and microvessels with laser speckle contrast imaging. Appl Opt. 2007;46(10):1911–1917.
- Bischof JC, Padanilam J, Holmes WH, et al. Dynamics of cell membrane permeability changes at supraphysiological temperatures. Biophys J. 1995;68(6):2608–2614.
- Chen B, Zhou M, Xu LX. Study of vascular endothelial cell morphology during hyperthermia. J Therm Biol. 2005;30(2):111–117.
- Sen A, Capitano ML, Spernyak JA, et al. Mild elevation of body temperature reduces tumor interstitial fluid pressure and hypoxia and enhances efficacy of radiotherapy in murine tumor models. Cancer Res. 2011;71(11):3872–3880.
- Vaupel P, Horsman MR. Tumour perfusion and associated physiology: characterization and significance for hyperthermia. Int J Hyperthermia. 2010;26(3):209–210.
- Los G, van Vugt MJ, Pinedo HM. Response of peritoneal solid tumours after intraperitoneal chemohyperthermia treatment with cisplatin or carboplatin. Br J Cancer. 1994;69(2):235–241.
- Elias D, Bonnay M, Puizillou JM, et al. Heated intra-operative intraperitoneal oxaliplatin after complete resection of peritoneal carcinomatosis: pharmacokinetics and tissue distribution. Ann Oncol. 2002;13(2):267–272.
- Kong G, Braun RD, Dewhirst MW. Hyperthermia enables tumor-specific nanoparticle delivery: effect of particle size. Cancer Res. 2000;60(16):4440–4445.
- Kong G, Braun RD, Dewhirst MW. Characterization of the effect of hyperthermia on nanoparticle extravasation from tumor vasculature. Cancer Res. 2001;61(7):3027–3032.
- Needham D, Anyarambhatla G, Kong G, et al. A new temperature-sensitive liposome for use with mild hyperthermia: characterization and testing in a human tumor xenograft model. Cancer Res. 2000;60(5):1197–1201.
- Ranjan A, Jacobs GC, Woods DL, et al. Image-guided drug delivery with magnetic resonance guided high intensity focused ultrasound and temperature sensitive liposomes in a rabbit Vx2 tumor model. J Control Release. 2012;158(3):487–494.
- Staruch RM, Ganguly M, Tannock IF, et al. Enhanced drug delivery in rabbit VX2 tumours using thermosensitive liposomes and MRI-controlled focused ultrasound hyperthermia. Int J Hyperthermia. 2012;28(8):776–787.
- Staruch RM, Hynynen K, Chopra R. Hyperthermia-mediated doxorubicin release from thermosensitive liposomes using MR-HIFU: therapeutic effect in rabbit Vx2 tumours. Int J Hyperthermia. 2015;31(2):118–133.
- Paulides MM, Dobsicek Trefna H, Curto S, et al. Recent technological advancements in radiofrequency- and microwave-mediated hyperthermia for enhancing drug delivery. Adv Drug Deliv Rev. 2020;163–164:3–18.
- He M, Sun J, Zhao D, et al. Modified-FOLFIRINOX combined with deep regional hyperthermia in pancreatic cancer: a retrospective study in Chinese patients. Int J Hyperthermia. 2019;36(1):394–402.
- Zagar TM, Vujaskovic Z, Formenti S, et al. Two phase I dose-escalation/pharmacokinetics studies of low temperature liposomal doxorubicin (LTLD) and mild local hyperthermia in heavily pretreated patients with local regionally recurrent breast cancer. Int J Hyperthermia. 2014;30(5):285–294.
- Gray MD, Lyon PC, Mannaris C, et al. Focused ultrasound hyperthermia for targeted drug release from thermosensitive liposomes: results from a phase I trial. Radiology. 2019;291(1):232–238.
- De Maar JS, Suelmann BBM, Braat MNGJA, et al. Phase I feasibility study of Magnetic Resonance guided High Intensity Focused Ultrasound-induced hyperthermia, Lyso-Thermosensitive Liposomal Doxorubicin and cyclophosphamide in de novo stage IV breast cancer patients: study protocol of the i-GO study. BMJ Open. 2020;10(11):e040162.
- Aubry J-F, Pernot M, Marquet F, et al. Transcostal high-intensity-focused ultrasound: ex vivo adaptive focusing feasibility study. Phys Med Biol. 2008;53:2937–2951.
- Marquet F, Aubry JF, Pernot M, et al. Optimal transcostal high-intensity focused ultrasound with combined real-time 3D movement tracking and correction. Phys Med Biol. 2011;56(22):7061–7080.
- Cochard E, Aubry JF, Tanter M, et al. Adaptive projection method applied to three-dimensional ultrasonic focusing and steering through the ribs. J Acoust Soc Am. 2011;130(2):716–723.
- Muhammad Z, Robert JD. 3D synthetic aperture imaging with a therapeutic spherical random phased array for transcostal applications. Phys Med Biol. 2020; 66(3):035024.
- Zubair M, Harput S, Dickinson R. 3D ultrasound image guidance and therapy through the rib cage with a therapeutic random phased array. In 2018 IEEE International Ultrasonics Symposium, 2018, p. 1–9.
- Zubair M, Dickinson RJ. Simulation of a modified multielement random phased array for image guidance and therapy. In 2019 IEEE 16th International Symposium on Biomedical Imaging (ISBI 2019); 2019.
- Zubair M, Dickinson R. Calculating the effect of ribs on the focus quality of a therapeutic spherical random phased array. Sensors. 2021;21(4):1211.
- Pioche M, Lafon C, Constanciel E, et al. High-intensity focused ultrasound liver destruction through the gastric wall under endoscopic ultrasound control: first experience in living pigs. Endoscopy. 2012;44(Suppl 2 UCTN):E376–E377.
- Adams MS, Salgaonkar VA, Plata-Camargo J, et al. Endoluminal ultrasound applicators for MR-guided thermal ablation of pancreatic tumors: preliminary design and evaluation in a porcine pancreas model. Med Phys. 2016;43(7):4184–4184.
- Li T, Khokhlova T, Maloney E, et al. Endoscopic high-intensity focused US: technical aspects and studies in an in vivo porcine model (with video). Gastrointest Endosc. 2015;81(5):1243–1250.
- Adams MS, Scott SJ, Salgaonkar VA, et al. Thermal therapy of pancreatic tumours using endoluminal ultrasound: parametric and patient-specific modelling. Int J Hyperthermia. 2016;32(2):97–111.
- Melodelima D, Salomir R, Chapelon JY, et al. Intraluminal high intensity ultrasound treatment in the esophagus under fast MR temperature mapping: in vivo studies. Magn Reson Med. 2005;54(4):975–982.
- Melodelima D, Prat F, Fritsch J, et al. Treatment of esophageal tumors using high intensity intraluminal ultrasound: first clinical results. J Transl Med. 2008;6(1):28.
- Chopra R, Burtnyk M, Haider MA, et al. Method for MRI-guided conformal thermal therapy of prostate with planar transurethral ultrasound heating applicators. Phys Med Biol. 2005;50(21):4957–4975.
- Diederich CJ, Stafford RJ, Nau WH, et al. Transurethral ultrasound applicators with directional heating patterns for prostate thermal therapy: in vivo evaluation using magnetic resonance thermometry. Med Phys. 2004;31(2):405–413.
- Adams MS, Scott SJ, Salgaonkar VA, et al. Development of an endoluminal high-intensity ultrasound applicator for image-guided thermal therapy of pancreatic tumors. Proc SPIE Int Soc Opt Eng. 2015;9326:93260F.
- Meininger GR. Initial experience with a novel focused ultrasound ablation system for ring ablation outside the pulmonary vein. J Interv Cardiac Electrophysiol. 2003;8(2):141–148.
- Nakagawa H, Antz M, Wong T, et al. Initial experience using a forward directed, high-intensity focused ultrasound balloon catheter for pulmonary vein antrum isolation in patients with atrial fibrillation. J Cardiovasc Electrophysiol. 2007;18(2):136–144.
- Sinelnikov YD, Fjield T, Sapozhnikov OA. The mechanism of lesion formation by focused ultrasound ablation catheter for treatment of atrial fibrillation. Acoust Phys. 2009;55(4–5):647–656.
- Sinelnikov YD, Sutin AM, Vedernikov AY, et al. Time reversal acoustic focusing with a catheter balloon. Ultrasound Med Biol. 2010;36(1):86–94.
- Adams MS, Diederich CJ. Deployable cylindrical phased-array applicator mimicking a concentric-ring configuration for minimally-invasive delivery of therapeutic ultrasound. Phys Med Biol. 2019;64(12):125001.
- Hynynen K. Acoustic power calibrations of cylindrical intracavitary ultrasound hyperthermia applicators. Med Phys. 1993;20(1):129–134.
- Hasgall PA, Baumgartner C, Neufeld E, et al. IT’IS Database for thermal and electromagnetic parameters of biological tissues. Version 4.0.
- Adams MS, Salgaonkar VA, Scott SJ, et al. Integration of deployable fluid lenses and reflectors with endoluminal therapeutic ultrasound applicators: preliminary investigations of enhanced penetration depth and focal gain. Med Phys. 2017;44(10):5339–5356.
- Pennes HH. Analysis of tissue and arterial blood temperatures in the resting human forearm. J Appl Physiol. 1948;1(2):93–122.
- Cameron J. Physical properties of tissue. A comprehensive reference book, edited by Francis A. Duck. Med Phys. 1991;18(4):834–834.
- Komar G, Kauhanen S, Liukko K, et al. Decreased blood flow with increased metabolic activity: a novel sign of pancreatic tumor aggressiveness. Clin Cancer Res. 2009;15(17):5511–5517.
- Haemmerich D, Wright AW, Mahvi DM, et al. Hepatic bipolar radiofrequency ablation creates coagulation zones close to blood vessels: a finite element study. Med Biol Eng Comput. 2003;41(3):317–323.
- Dos Santos I, Haemmerich D, da Silva Pinheiro C, et al. Effect of variable heat transfer coefficient on tissue temperature next to a large vessel during radiofrequency tumor ablation. Biomed Eng. 2008;7(1):21.
- Cain CA, Umemura S. Concentric-ring and sector-vortex phased-array applicators for ultrasound hyperthermia. IEEE Trans Microwave Theory Technol. 1986;34(5):542–551.
- Fjield T, Fan X, Hynynen K. A parametric study of the concentric‐ring transducer design for MRI guided ultrasound surgery. J Acoust Soc Am. 1996;100(2):1220–1230.
- Ryan TP, Hartov A, Colacchio TA, et al. Analysis and testing of a concentric ring applicator for ultrasound hyperthermia with clinical results. Int J Hyperthermia. 1991;7(4):587–603.
- Rösch T, Lightdale CJ, Botet JF, et al. Localization of pancreatic endocrine tumors by endoscopic ultrasonography. New Eng J Med. 1992;326(26):1721–1726.
- Zamboni GA, Ambrosetti MC, M Pezzullo M, et al. Optimum imaging of chronic pancreatitis. Abdominal Radiol. 2020;45(5):1410–1419.
- Yousaf MN, Chaudhary FS, Ehsan A, et al. Endoscopic ultrasound (EUS) and the management of pancreatic cancer. BMJ Open Gastroenterol. 2020;7(1):e000408.
- Burdette EC, Filip F, Diederich CJ, et al. Conformal needle-based ultrasound ablation using EM-tracked conebeam CT image guidance. Proc Spie. 2011; SPIE 790107.
- Diederich CJ, Wootton J, Prakash P, et al. Catheter-based ultrasound hyperthermia with HDR brachytherapy for treatment of locally advanced cancer of the prostate and cervix. Proc SPIE Int Soc Opt Eng. 2011;7901:79010O.
- N’Djin WA, Burtnyk M, Lipsman N, et al. Active MR-temperature feedback control of dynamic interstitial ultrasound therapy in brain: in vivo experiments and modeling in native and coagulated tissues. Med Phys. 2014;41(9):093301.
- Burtnyk M, Chopra R, Bronskill MJ. Quantitative analysis of 3-D conformal MRI-guided transurethral ultrasound therapy of the prostate: theoretical simulations. Int J Hyperthermia. 2009;25(2):116–131.
- Rieke V, Butts Pauly K. MR thermometry. J Magn Reson Imaging. 2008;27(2):376–390.
- Grissom WA, Rieke V, Holbrook AB, et al. Hybrid referenceless and multibaseline subtraction MR thermometry for monitoring thermal therapies in moving organs. Med Phys. 2010;37(9):5014–5026.
- Roujol S, Ries M, Quesson B, et al. Real-time MR-thermometry and dosimetry for interventional guidance on abdominal organs. Magn Reson Med. 2010;63(4):1080–1087.
- Partanen A, Tillander M, Yarmolenko PS, et al. Reduction of peak acoustic pressure and shaping of heated region by use of multifoci sonications in MR-guided high-intensity focused ultrasound mediated mild hyperthermia. Med Phys. 2012;40(1):013301.
- Partanen A, Yarmolenko PS, Viitala A, et al. Mild hyperthermia with magnetic resonance-guided high-intensity focused ultrasound for applications in drug delivery. Int J Hyperthermia. 2012;28(4):320–336.
- Chopra R, Baker N, Choy V, et al. MRI-compatible transurethral ultrasound system for the treatment of localized prostate cancer using rotational control. Med Phys. 2008;35(4):1346–1357.
- Goharrizi AY, N'djin WA, Kwong R, et al. Development of a new control strategy for 3D MRI-controlled interstitial ultrasound cancer therapy. Med Phys. 2013;40(3):033301.
- Kangasniemi M, Diederich CJ, Price RE, et al. Multiplanar MR temperature-sensitive imaging of cerebral thermal treatment using interstitial ultrasound applicators in a canine model. J Magn Reson Imaging. 2002;16(5):522–531.
- Delabrousse E, Salomir R, Birer A, et al. Automatic temperature control for MR-guided interstitial ultrasound ablation in liver using a percutaneous applicator: ex vivo and in vivo initial studies. Magn Reson Med. 2010;63(3):667–679.
- Nau WH, Diederich CJ, Ross AB, et al. MRI-guided interstitial ultrasound thermal therapy of the prostate: a feasibility study in the canine model. Med Phys. 2005;32(3):733–743.
- Lafon C, Melodelima D, Salomir R, et al. Interstitial devices for minimally invasive thermal ablation by high-intensity ultrasound. Int J Hyperthermia. 2007;23(2):153–163.
- Scott SJ, Prakash P, Salgaonkar V, et al. Approaches for modelling interstitial ultrasound ablation of tumours within or adjacent to bone: theoretical and experimental evaluations. Int J Hyperthermia. 2013;29(7):629–642.
- Issels RD, Lindner LH, Verweij J, et al. Neo-adjuvant chemotherapy alone or with regional hyperthermia for localised high-risk soft-tissue sarcoma: a randomised phase 3 multicentre study. Lancet Oncol. 2010;11(6):561–570.
- Merten R, Ott O, Haderlein M, et al. Long‐term experience of chemoradiotherapy combined with deep regional hyperthermia for organ preservation in high‐risk bladder cancer (Ta, Tis, T1, T2). Oncologist. 2019;24(12): e1341–e1350.
- Melodelima D, Salomir R, Mougenot C, et al. 64-element intraluminal ultrasound cylindrical phased array for transesophageal thermal ablation under fast MR temperature mapping: an ex vivo study. Med Phys. 2006;33(8):2926–2934.
- Diederich CJ, Hynynen K. Ultrasound technology for hyperthermia. Ultrasound Med Biol. 1999;25(6):871–887.
- Babincová M, Leszczynska D, Sourivong P, et al. Blood-specific whole-body electromagnetic hyperthermia. Med Hypotheses. 2000;55(6):459–460.
- Lyon PC, Gray MD, Mannaris C, et al. Safety and feasibility of ultrasound-triggered targeted drug delivery of doxorubicin from thermosensitive liposomes in liver tumours (TARDOX): a single-centre, open-label, phase 1 trial. Lancet Oncol. 2018;19(8):1027–1039.
- Lyon PC, Mannaris C, Gray M, et al. Large-volume hyperthermia for safe and cost-effective targeted drug delivery using a clinical ultrasound-guided focused ultrasound device. Ultrasound Med Biol. 2021;47(4):982–997.
- Cheng B, Bing C, Staruch RM, et al. The effect of injected dose on localized tumor accumulation and cardiac uptake of doxorubicin in a Vx2 rabbit tumor model using MR-HIFU mild hyperthermia and thermosensitive liposomes. Int J Hyperthermia. 2020;37(1):1052–1059.
- Wu S-K, Santos MA, Marcus SL, et al. MR-guided focused ultrasound facilitates sonodynamic therapy with 5-aminolevulinic acid in a rat Glioma Model. Sci Rep. 2019;9(1):10465.
- Bai Z, Shi Y, Wang J, et al. Intratumoral radiofrequency hyperthermia-enhanced direct chemotherapy of pancreatic cancer. Oncotarget. 2017;8(2):3591–3599.
- Maloney E, Khokhlova T, Pillarisetty VG, et al. Focused ultrasound for immuno-adjuvant treatment of pancreatic cancer: an emerging clinical paradigm in the era of personalized oncotherapy. Int Rev Immunol. 2017;36(6):338–351.
- Cohen G, Chandran P, Lorsung RM, et al. The impact of focused ultrasound in two tumor models: temporal alterations in the natural history on tumor microenvironment and immune cell response. Cancers. 2020;12(2):350.
- Kirui DK, Koay EJ, Guo X, et al. Tumor vascular permeabilization using localized mild hyperthermia to improve macromolecule transport. Nanomedicine. 2014;10(7):1487–1496.
- Harrington KJ, Mohammadtaghi S, Uster PS, et al. Effective targeting of solid tumors in patients with locally advanced cancers by radiolabeled pegylated liposomes. Clin Cancer Res. 2001;7(2):243.
- Ta T, Porter TM. Thermosensitive liposomes for localized delivery and triggered release of chemotherapy. J Control Release. 2013;169(1):112–125.
- Li Z, Deng J, Sun J, et al. Hyperthermia targeting the tumor microenvironment facilitates immune checkpoint inhibitors. Front Immunol. 2020;11:595207.
- Oei AL, Korangath P, Mulka K, et al. Enhancing the abscopal effect of radiation and immune checkpoint inhibitor therapies with magnetic nanoparticle hyperthermia in a model of metastatic breast cancer. Int J Hyperthermia. 2019;36(Suppl 1):47–63.