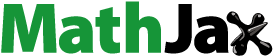
Abstract
Aim
Metal implant infections are a devastating problem due to the formation of biofilm which impairs the effectiveness of antibiotics and leads to surgical replacement as definitive treatment. Biofilm on metal implants can be reduced using heat generated by alternating magnetic fields (AMF). In this study, the relationship between implant surface biofilm reduction and surrounding tissue thermal damage during AMF exposure is investigated through numerical simulations.
Methods
Mathematical models of biofilm reduction with heat were created based on in vitro experiments. Simulations were performed to predict the spatial and temporal heating on the implant surface and surrounding tissue when exposed to AMF.
Results
The modeling results show that intermittent and slow heating can achieve biofilm reduction with a narrow zone of tissue damage around an implant of less than 3 mm. The results also emphasize that uniformity of implant heating is an extremely important factor impacting the effectiveness of biofilm reduction. For a knee implant, using a target temperature of 75 °C, an intermittent treatment strategy of 15 exposures (10 s to target temperature followed by cooldown) achieved a bacterial CFU reduction of 6-log10 across 25% of the implant surface with less than 3 mm of tissue damage. Alternatively, a single 60 s heating exposure to same temperature achieved a bacterial reduction of 6-log10 across 85% of the implant surface, but with 4 mm of tissue damage.
Conclusion
Overall, this study demonstrates that with uniform heating to temperatures above 70 °C, an implant surface can be largely reduced of biofilm, with only a few mm of surrounding tissue damage.
1. Introduction
Metal implants are used across a broad range of medical specialties including joint reconstruction, trauma, coronary stents, intra-uterine devices and dental implants [Citation1,Citation2]. Although these implants offer improvements in quality of life, they are also subject to failure. One of the most devastating complications following implantation is infection [Citation3–5], which often occurs within the first year of implant placement [Citation6]. The rates of infection range from 1–2% for prosthetic knee joints [Citation7], to upwards of 10–30% for orthopedic hardware associated with open fractures [Citation8]. In the United States, the gold standard for a prosthetic joint infection (PJI) is a two stage revision arthroplasty with prolonged antibiotic therapy [Citation9]. The treatment is highly invasive, expensive and difficult to undergo in patients with high comorbidity factors [Citation10], with an estimated failure rate of 25% two years post-procedure [Citation9]. The estimated cost of treatment for PJI in the US is $50,000–$60,000, adding up to a projected cost of $1.85 billion annually by the year 2030 [Citation11].
The difficulty in treating PJI is due to biofilm formation around the implant [Citation12]. Biofilm formation is a multistage process which starts with planktonic bacterial surface attachment, followed by intercellular adhesion and multilayered bacterial cell proliferation in an extracellular polysaccharide matrix. This process sets up an altered microenvironment for bacteria to thrive unhindered by the body’s natural immunologic protective response and antibiotic treatment [Citation3]. Consequently, the formation of antibiotic tolerant communities of bacteria leads to reduced long-term effectiveness of conventional treatment [Citation13,Citation14].
Studies have reported that heat can reduce biofilm on implant surfaces and can also increase its sensitivity to antibiotics [Citation8,Citation15–17]. Although promising, the application of heat to internal metal implants is a practical challenge. Recently it has been proposed that alternating magnetic fields (AMF) could be used as a method to heat-infected metal implants non-invasively to target biofilms [Citation10,Citation18–20]. When metal implants are exposed to AMF, eddy currents are induced and heat is generated through resistive losses [Citation21]. The flow of electrical currents can be restricted to the metal surface by exploiting a phenomenon known as the skin effect [Citation21]. The skin depth, the depth at which the current density is reduced by 1/e of the surface current, is a function of frequency and is less than 1 mm at 200 kHz in stainless steel. This enables a means to heat the outer surface of an implant where the target biofilm is located and avoids heating the bulk volume of the implant. As the ratio of tissue to metal electrical conductivity is approximately 1e-6, there is minimal direct tissue heating in comparison to a metal implant [Citation10,Citation18,Citation22]. Previously we demonstrated proof-of-concept biofilm reduction with AMF in vitro. A time-dependent response was observed in colony-forming unit (CFU) reduction with a fixed AMF exposure, presumably due to heating [Citation18]. A real-time remote wireless safety mechanism was also developed to detect boiling associated with acoustic emissions between a metal-tissue interface to thus serve as a safety indicator during AMF treatment [Citation23]. Recently, we also described the ability to achieve a strong sensitization of biofilm to antibiotics through brief intermittent AMF exposures delivered in periodic doses [Citation24]. Studies by other groups also showed effective reduction of biofilm with heat and its synergistic effect on antibiotics [Citation8,Citation16,Citation19,Citation25–27]. AMF was found to be a promising way to heat biofilm and to study the synergistic effect with antibiotics [Citation19,Citation26,Citation27].
Although these initial in vitro studies indicate the ability of AMF to reduce biofilm on metals, the temporal dynamics of heating in vivo are more complex since there are competing trends of bacterial reduction and surrounding tissue damage. Furthermore, the rate of bacterial inactivation may not be the same as the inactivation rate for surrounding tissue cells. Understanding these dynamics of heating is critical to identifying parameters for the safe and effective delivery of AMF. This study addresses this issue through two main sets of investigations. The first is an experimental characterization of the survival of common biofilm pathogens to heat to determine inactivation rates. The second is a numerical study that utilizes these inactivation rates, along with accepted thermal dosing rates for human tissue to explore the relationship between biofilm reduction and surrounding tissue damage. Different treatment parameters are investigated such as bacterial response to the different target temperatures, continuous versus intermittent heating, the effect of implant cool down and non-uniform power deposition on the implant. The results of this study provide a foundation for suitable treatment parameters using AMF to reduce biofilm.
2. Materials and methods
2.1. Determining heat inactivation rates for biofilm
2.1.1. Biofilm incubation on metal rings
Biofilm was grown on stainless steel rings (316 L, 3/4″ OD, 0.035″ wall thickness, 0.21″ height, cut from McMaster Carr, P/N 89785K857, USA) using the Gram-negative pathogen Pseudomonas aeruginosa (PAO1: ATCC strain, and MB699: provided by Sam Shelburne) or gram-positive pathogen Staphylococcus aureus (UAMS1, provided by M. Smeltzer, and TIDB1675, provided by Marissa Badham). PAO1 is a laboratory P. aeruginosa strain. MB699 is a multi-drug tolerant P. aeruginosa strain. UAMS1 is a clinical isolate of methicillin-susceptible S. aureus (MSSA). TIDB1675 is a methicillin-tolerant S. aureus (MRSA) derived from human orthopedic infections. For P. aeruginosa biofilm, an isolated colony was inoculated into 3 ml of cation-adjusted Mueller Hinton II (MHII) media (Becton-Dickinson by Thermo-Fisher Scientific) and incubated at 37 °C for 18 h at 220 RPM. A working solution was made by adding culture to sterile phosphate-buffered saline (PBS). The bacterial concentration was adjusted with MHII using a UV spectrophotometer (Genesys 20, Thermal Scientific) at 600 nm until the optical density (OD) read between 0.07 to 0.08, indicating a concentration of ∼108 CFU/mL. The working solution was then diluted to obtain a bacterial concentration of 5 × 105 CFU/mL. Biofilm was prepared on each metal ring by placing the ring in 5 ml of the bacterial solution in a 50 ml conical tube. The submerged ring was then incubated at 37 °C for 48 h at 110 RPM in a shaking incubator (Innova42, New Brunswick Scientific). The biofilms were transferred into a new tube containing sterile 5 ml MHII, and there was no rinse during biofilm growth phase. Biofilm prepared with S. aureus followed the same protocol using Tryptic Soy Broth (TSB, Becton-Dickinson by Thermo-Fisher Scientific) instead as the growth media. All strains of biofilms tested in this study were prepared using this protocol.
2.1.2. Biofilm heat mitigation
A temperature-controlled water bath (Model 1235, VWR Scientific) was used to conduct the heat treatment. About 50 ml tubes with 10 ml fresh media were placed in the water bath and pre-heated to a target temperature. For P. aeruginosa, MHII media was used, and TSB media was used for S. aureus. The 48-h biofilm was transferred into the pre-heated media using a sterile hook and immersed for a specified time. Then the heat-treated biofilm was immediately transferred to 5 ml sterile PBS, which had been placed on ice for 30 min, to quench the heating and rinse. After that, the biofilm was transferred to 4 ml of sterile PBS and sonicated in an ultrasonic water bath for 5 min. Bacterial density on the ring surface was quantified by plating 10 µL of the sonicated PBS on blood agar plates (TSA w/sheep blood, Thermo Fisher Scientific) using a standard serial dilution drip method. A plate with 100-μl sonicated PBS was also included. Three biological replicates were obtained for each experimental condition (specified temperature and time), and three technical replicates were utilized per experiment. A temperature range between 60 and 70 °C was chosen for study since temperatures below this had minimal effect on biofilm reduction, and higher temperatures were difficult to quantify due to the rapid reduction in biofilm. The limit of detection is 0.78 log10 (CFU/cm2) with the initial CFU density around 6.5 log10 (CFU/cm2), the dynamic range would be 6–7 log10. For each strain, the CFU reduction as a function of time was obtained for five distinct temperatures within this range (60, 62.5, 65, 67.5, 70 °C).
2.2. Arrhenius inactivation rate modelling
The heat inactivation data were fit to an Arrhenius model to characterize the rate of inactivation for the different biofilm strains. The basic Arrhenius model can be expressed as [Citation28]
(1)
(1)
(2)
(2)
where k is the rate constant, T is the absolute temperature in Kelvin, A is the pre-exponential factor, Ea is the activation energy for the reaction (in the same units as RT), R is the universal gas constant. Assuming the CFU reduction follows a log-linear reduction, where N is the log10 of CFU decrease log (CFU(t)/CFU(t = 0)) in a heat treatment of time t. The equation can be modified as,
(3)
(3)
where A and B are constants, B is defined as Ea/2.303R. This traditional log-linear model is the best-known primary survival model, and it is still widely used in sterility calculations in the food, pharmaceutical, and other industries [Citation29,Citation30]. A and B can be obtained through linear fitting by log(N/t) and 1/T. With A and B derived from this linear fit, a model for CFU reduction (N) as a function of temperature and time can be expressed as,
(4)
(4)
The threshold of 50 C was selected as no biofilm reduction was observed from water bath experiments.
2.3. Numerical simulations
Simulations were performed using the commercial finite-element simulation software COMSOL Multiphysics. (Comsol v5.5, Los Angeles, CA, USA). A quasi-static approximation of the Maxwell equation (ACDC module) was used to study the effect of magnetic field and the Penne’s bioheat-transfer model (Heat transfer module) was used for heat transfer analysis [Citation31]:
(5)
(5)
where ρ is the density of tissue or metal (kg/m3), C is the tissue or metal-specific heat capacity (J·kg−1·°C−1), T is the temperature (°C), t is time (s), k is the tissue or metal thermal conductivity (W·m−1·°C −1), Qm is the metabolic heat-generation rate (W·m−3), ωb is the perfusion rate (kg·m−3·s−1), and ρb, cb, and Tb correspond to the density, specific heat, and temperature of blood, respectively [Citation32–34]. The perfusion term was not included in regions where the metal implant was defined.
A user defined heat source was used to approximate uniform skin effect heating [Citation21]
(6)
(6)
(7)
(7)
where Js is the surface current density (A·m−2), r is the radial distance from the surface (m), δ is the skin depth (m), rho is the resistivity of the conductor (Ω·m), ω is the angular frequency (Hz), μ is the product of relative magnetic permeability of the conductor and the permeability of free space (H·m−1).
The thermal tissue damage generated in adjacent tissues during implant heating was estimated using the widely used thermal dose model, which is represented as cumulative equivalent minutes (CEM; at 43 °C) [Citation35]. This provides the time–temperature relationship in equivalent minutes, and it can be expressed as follows:
(8)
(8)
where R is the temperature dependence of the rate of cell death, with R = 0.5 for T > 43 °C, R = 0.25 for 43 °C ≥ T ≥ 39 °C, and R = 0.00 for T < 39 °C. Additionally, dt is the time interval (min), T is the average temperature (°C) during time interval dt, and to and tfinal are the initial and final heating periods (min), respectively [Citation32,Citation34].
shows the 2D axisymmetric model of a spherical metal implant surrounded by muscle that was used for these simulations. A spherical implant was chosen for these simulations since the goal was to describe the temporal dynamics of surface biofilm reduction and tissue thermal damage without being influenced by implant geometry. Therefore, these spherically symmetric simulations represent the best-case situation of these thermal dynamics. The diameter of the spherical implant was 8 cm to match the size of a typical orthopedic implant such as a prosthetic knee joint [Citation36,Citation37]. A set of simulations were also performed using a model of a prosthetic knee implant when the effect of geometry was being studied. The knee implant is an in-house developed CAD model based on existing clinical implants on the market. An initial temperature of 37 °C was used for the implant and muscle tissue and a large surrounding tissue layer was assumed to avoid the outer boundary effects. The physical tissue and implant properties used in the simulations are listed in . Muscle electrical and thermal properties were obtained from the IT’IS Foundation, Zurich, Switzerland [Citation38] and muscle blood perfusion and implant electrical and thermal properties are taken from the literature [Citation18,Citation39]. shows the temperature distribution after 10 s of skin effect heating, showing the radial drop off of temperatures into the implant and in tissue. Radial distances of 1, 2 and 3 mm from the implant surface were analyzed for thermal dose as shown.
Figure 1. Computational model used in the study: (a) 2D axisymmetric model of a spherical metal implant and surrounding tissue used in this study (R1 = 4 cm, R2 = 12 cm). (b) The spatial temperature distribution through a cross-section of the implant after 10s of uniform skin-effect (0.9681 mm at 200 kHz) heating shows the conduction of heat inward into the metal implant and outward into tissue. The reduction of bacteria on the surface, and the tissue thermal dose at 1, 2 and 3 mm were quantified for different exposure conditions.
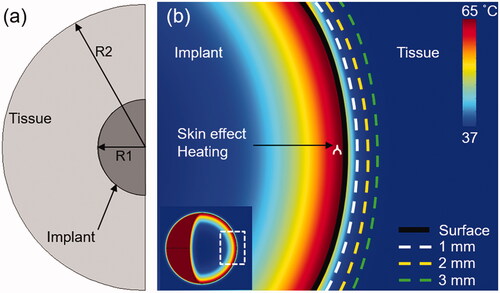
Table 1. Physical properties of materials used in simulations.
Table 2. Arrhenius coefficients of different bacterial strains.
3. Results
shows the reduction of biofilm (in log10 units) as a function of exposure time for different incubation temperatures in the water bath apparatus. A log-linear relationship was observed when the bacterial strains were exposed to temperatures between 60 and 70 °C. Temperatures below 60 °C were either ineffective or took impractically long times (>20 min) to achieve significant reductions. Temperatures above 70 °C were too difficult to quantify due to the rapid reduction in biofilm. Very different rates of biofilm reduction were observed for Gram positive and negative strains. As seen in ), a 6-log10 reduction in biofilm burden required approximately 20 s for P. aeruginosa (PAO1) at 65 °C, whereas for S. aureus (TIDB1657) it required almost 200 s. shows the Arrhenius plots for two different bacterial strains of S. aureus (TIDB1657, UAMS) and two different strains of P. aeruginosa (PAO1 and MB699). All strains exhibited a linear relationship between the rate of bacterial reduction and the inverse of the incubation temperature. Inactivation rates grouped by cell wall characteristics with Gram positive strains grouping together and the Gram negative strains grouping together, as seen in . Another interesting observation was that the rate of heat inactivation for a given strain (i.e., Gram positive or negative) was independent of whether it was drug sensitive or tolerant.
Figure 2. Reduction of biofilm with heat: log 10 (CFU) reduction of biofilm as a function of time for different incubation temperatures. (a) S. aureus (TIDB1675) strain (b) P. aeruginosa (PA01) strain (c) Arrhenius fitting of four different bacterial strains show consistency with gram positive and gram negative strains, but different rates of elimination across strains.
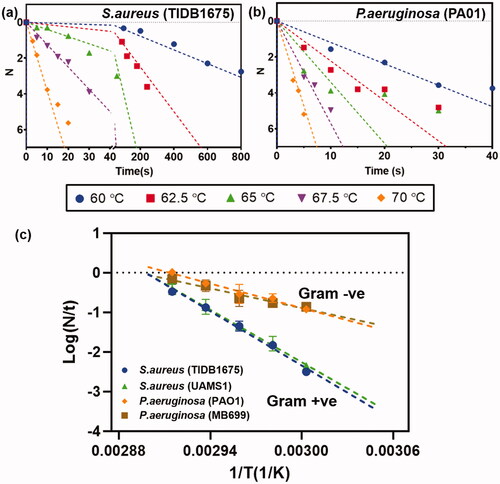
Figure 3. Effect of skin effect heating: Comparison between (a) volumetric (-) and (b) skin effect (--) heating of a metal implant with a target temperature of 65°C in 10s. (a,b) Spatial maps of the surface and internal temperature distribution show the faster cool down and lower surface temperatures for skin-effect heating of the implant. Conduction of heat into the implant for skin effect heating accounts for this difference in surface temperature. (c) The temperature profiles on the surface and in tissue reveal a significantly lower temperature achieved in tissue between skin effect and volumetric heating. (d) Radial tissue damage as a function of the time required to reach a target temperature. Faster heating leads to a greater reduction in tissue damage radius for skin effect heating, however, as the heating rate is reduced, the differences become less significant between the two cases.
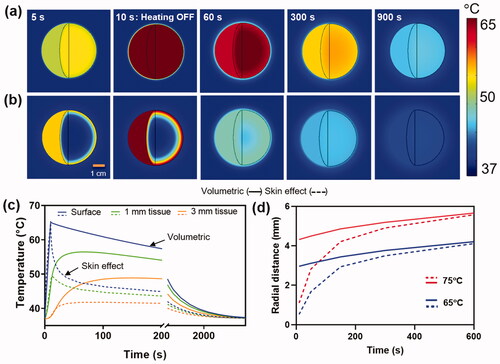
The differences between volumetric and skin effect heating of a metal implant are illustrated in . In these simulations, volumetric and skin effect heating was applied on a spherical metal implant of 8 cm diameter for 10 s, reaching a surface temperature of 65 °C in that time. ) shows the surface and internal temperatures of a metal sphere during the initial heating (5 s), at peak temperature (10 s) and cooldown (60, 300, 900 s). The faster cooldown of the implant surface with skin effect heating is apparent, principally due to the inward conduction of heat into the cooler regions of the implant. shows the temperature profiles over time at 0, 1 and 3 mm from the implant surface. The increased rate of surface cooling with skin effect heating is most apparent in the first 100 s and results in a >10 °C cooler average temperature in tissue compared with volumetric heating. This leads to a significantly lower accumulated thermal dose in adjacent soft tissues as shown in . In this example of 10 s heating to 65 °C, the radial extent of irreversible tissue damage (CEM43 > 240 min) after cooldown is 0.5 mm with skin-effect heating, whereas it is 2.9 mm in the case of volumetric heating. The impact of heating time and peak temperature on the radius of thermal damage for skin and volumetric heating is shown in . Most important is the fact that the difference between skin and volumetric heating disappears as the heating rate decreases. For fast heating (∼10 s), skin effect heating produces a damage radius ∼18% of volumetric heating. For medium rates of heating (∼60 s), there is still an advantage of skin effect heating, with the radius of thermal damage 58% of volumetric heating. However, for slow heating (∼5 min), the two cases produce essentially the same radius of tissue damage. This trend is observed for both target temperatures 65 °C and 75 °C.
depicts the reduction of S. aureus (TIDB1657) biofilm as a function of exposure duration when the implant surface is heated uniformly at a fixed temperature. S. aureus was chosen as a worst case scenario due to the increased heat tolerance compared to P aeruginosa. From , it is seen that at a temperature of 60 °C, 300 s are required to achieve ∼95% reduction (N = 1.25) of biofilm on the implant surface, at which point the radius of irreversible tissue damage (CEM43 > 240 min) is 3 mm. Similar trends for biofilm reduction and radial tissue damage are shown ( for a range of implant surface temperatures ranging from 60 to 80 °C, along with the times when the radius of irreversible tissue damage reaches 1, 2 and 3 mm. shows that surface temperatures above 70 °C can achieve > 6-log10 reduction of S. aureus (TIDB1657) biofilm in 12 s while restricting tissue damage to less than 1 mm. In contrast, at 65 °C it requires close to 150 s to achieve the same reduction of biofilm, and the radial tissue damage is 3 mm. For temperatures below 65 °C, it is not possible to achieve more than 1 log10 reduction in biofilm in a reasonable time and is therefore impractical to use for this strain of bacteria. This large variation in response to temperature is a result of the Arrhenius behavior of bacteria across this temperature range.
Figure 4. Instantaneous heating of implant: a) The reduction of S. aureus (TIDB1675) biofilm as a function of time for uniform implant heating at 60°C. Superimposed are the times when tissue thermal dose reaches a CEM43 value of 240 min (irreversible damage) at 1, 2 and 3 mm. From the graph it is seen that in order to achieve ∼95% reduction (N = 1.25) of biofilm on the implant surface tissue is irreversibly damaged to a radius of 3mm. b) Similar trends for biofilm reduction as a function of time are shown for a range of implant surface temperature ranging from 60–80°C, along with the times for tissue damage at 1,2 and 3 mm. The image shows that temperatures above 70°C can reduce biofilm (N > 6) in a few seconds while restricting tissue damage to less than a few 1 mm.
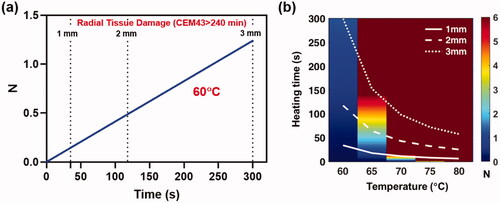
Previously, we have observed that intermittent heating and cooling of a metal surface with AMF and antibiotics can achieve biofilm reduction [Citation24]. explores the relationship between biofilm reduction and tissue thermal damage for intermittent heating. The intermittent heating approach, with repeated heating to a target temperature, followed by a cool-down period to baseline, is shown in . For these simulations, the rate of heating was varied such that the target temperature was reached in 10, 35 or 60 s, as seen in . The target temperature was varied between 60 and 80 °C for both P. aeruginosa (PAO1) and S. aureus (TIDB1657) biofilm. The results show that each exposure achieves a successive reduction of biofilm on the implant. Due to the increased sensitivity to heat, P. aeruginosa (PAO1) requires lower temperatures and fewer exposures to achieve full reduction of the biofilm (>6 log10), and this can be achieved with <1 mm of thermal damage at all temperatures. In the case of S. aureus (TIDB1657), more exposures are required, and temperatures of at least 65–70 °C are necessary depending on the rate of heating. With a 10-s heating exposure, at least eight exposures at 70 °C are required to reduce the biofilm, with thermal damage being less than 2 mm in radius. As the rate of heating was reduced, the number of exposures required to achieve biofilm reduction was also reduced, requiring only one exposure at 70 °C with a 60 s heating time. However, the longer exposures also tended to increase the radial extent of thermal damage, but only by a small amount. Finally, if target temperatures >75 °C are implemented, the full reduction could be achieved in a single 10 s exposure, for both P. aeruginosa (PAO1) and S. aureus (TIDB1657).
Figure 5. Effect of intermittent heating of implant: Intermittent heating of implant by ramping up to different target temperatures 60–80°C. (a) Intermittent heating and cooling of implant targeting 65°C at 10 s, 35 s and 60 s. (b–g) The number of exposures and thermal damage radii (1,2,3 mm) as a function of the target temperature for fast (10 s), medium (35 s) and slow (60 s) exposure heating times. As the heating time for each exposure is increased, a faster reduction of bacteria is observed, and fewer exposures are required to reach a given surface reduction. However, slower heating is also associated with an increase in the radius of tissue damage for a given number of exposures. The best balance between achieving maximum surface bacteria reduction and minimizing radial tissue damage occurs with the use of higher temperatures, and the fastest possible heating time.
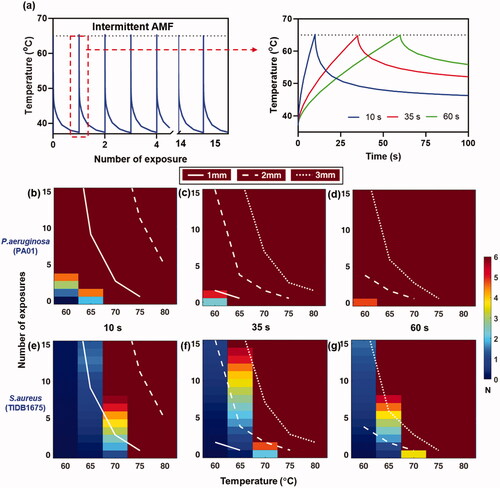
All the previous simulations assumed uniform surface heating of an implant, which in practice is impossible to achieve for a complex implant such as a knee. With a temperature distribution across the surface of the implant, one would expect corresponding distributions of biofilm reduction as well as radial tissue damage boundaries. The impact of heating uniformity on both the distribution of biofilm reduction and radial tissue damage is studied in . Three cases are considered: (1) heating with a uni-directional field along the z axis (as would be achieved with a solenoid or Helmholtz coil), (2) heating sequentially with uni-directional fields along the X, Y and Z axis (as could be achieved with sets of orthogonal Helmholtz coils) and (3) uniform heating. For uniform heating, a single exposure is only applied since it is the ideal case and the whole implant will be treated. Details on the uni-directional heating are given in Appendix A. shows a case where a spherical implant is heated to 75 °C through intermittent heating with nine exposures, each 10 s in duration, followed by cooldown, versus a single 60 s exposure, and compared these for the three different cases of heating uniformity. The details of the heating conditions are given in Supplementary Table 1. The histogram of bacterial reduction on the implant surface shows complete treatment (6 log10) for 25% of the surface area Z axis heating, 75% for sequential XYZ heating and 100% for uniform heating with intermittent exposure. The slower heating case showed an increase in treatment coverage to 30% for Z axis heating, 95% for sequential XYZ heating and 100% for uniform heating ()). The histogram of tissue damage distribution at a distance of 3 mm away from the implant surface shows that intermittent heating has minimal thermal damage CEM43 ≤ 60 min, while for slow heating the thermal dose was CEM43 ≥ 240 min. This example highlights how the slower 60 s heating can achieve virtually complete biofilm reduction on the implant, but with a penalty of more thermal tissue damage. The tissue damage beyond CEM 240 min was 3.2 mm from implant surface for slow heating. Slow heating also benefits from reduced treatment time. The overall treatment time required for slow heating is less than 0.5 h, whereas for intermittent heating it is 4.5 h which is a major difference for maximizing the treatment efficacy in a short time with minimal gain in safety.
Figure 6. Comparison between fast intermittent exposures (left column) and a single slow exposure (right column) on log10 reduction and tissue damage for a spherical implant. (a,b): Implant surface temperatures for the case where the magnetic field is applied in unidirectionally along the Z axis (left), sequentially along the X, Y, and Z axes (middle), and for uniform heating (right). Temperatures are shown for (a) intermittent (b) slow heating. (c,d): Biofilm reduction of S.aureus (TIDB1675) on the implant surface and a histogram of bacterial reduction (N) for (c)intermittent and (d) slow heating. (e,f): Tissue thermal dose distribution at 3 mm from implant surface and a histogram of thermal dose (CEM43) for (e) intermittent and (f) slow heating.
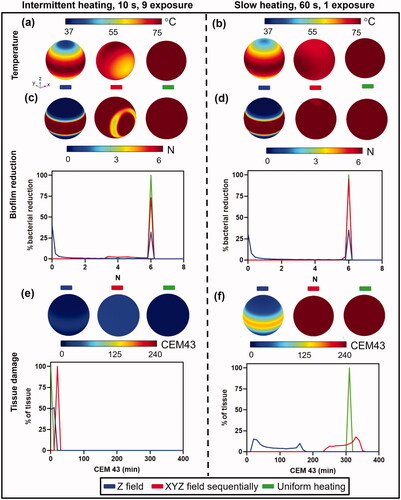
repeats the same types of heating exposures as described in , but using a 3D model of a human knee implant. The one difference in these simulations is a single field in the X-direction (left to right) was applied to simulate a partially uniform case. The implant model was comprised of a femoral (top) and tibial (bottom) component, and for these models it was assumed both were the same material (stainless steel). In practice, these implants can have different material compositions for each component, which adds another layer of complexity. evaluates the impact of heating the knee implants with intermittent or slow heating (parameters given in Supplementary Table 2). Due to the more complex geometry and less uniform temperature distribution compared to the sphere, the implant was aimed to heat to an average temperature of 75 °C but not exceeding a maximum of 85 °C . Both the surface biofilm reduction (S. aureus TIDB1657) and tissue thermal dose 3 mm from the implant are shown as surface distributions and histograms. shows the case where an implant was heated using a uni-axial Z-field, X-field with 15 exposures, each 10 s in duration and with a cooldown period of 1800 s in between and uniform heating. In contrast, shows the case of a single slow 60 s heating exposure with a uni-axial Z-field, X-field and uniform heating followed by cooldown back to baseline. The surface plots and histogram show that the range of temperatures across the implant were approximately 40–50 °C, and only a small fraction of the implant (<5%) was fully treated for a uni-axial Z-field. In the case of a uni-axial X-field, a much tighter temperature distribution was achieved, approximately 65–75 °C for the intermittent case, and 70–75 °C for the slow heating case. In this example, only 25% of the implant was treated with intermittent heating, but 85% was achieved using slow heating. The histogram of tissue damage distribution at a distance of 3 mm away from implant surface shows that intermittent heating has minimal thermal damage of CEM43 ≤ 100 min, while for slow heating the thermal dose increased to a CEM43 ≥ 240 min. As with the case of a spherical implant, these simulations show that intermittent heating in a knee implant is inefficient compared to slow heating for surface biofilm reduction, although it does produce less tissue damage at 3 mm. In addition, the treatment time differences are vast, with the slow heating treatment taking 60 s, while the intermittent approach requires 7.5 h of treatment time (due to the extended cooldown periods in between exposures).
Figure 7. Comparison between fast intermittent exposures (left column) and a single slow exposure (right column) on log10 reduction and tissue damage for a knee implant. (a,b): Implant surface temperatures for the case where the magnetic field is applied in unidirectionally along the Z axis (left), unidirectionally along the X axis (middle), and for uniform heating (right). Temperatures are shown for (a) intermittent (b) slow heating. (c,d): Biofilm reduction of S.aureus (TIDB1675) on the implant surface and a histogram of bacterial reduction (N) for (c) intermittent and (d) slow heating. (e,f): Tissue thermal dose distribution at 3 mm from implant surface and a histogram of thermal dose (CEM43) for (e) intermittent and (f) slow heating.
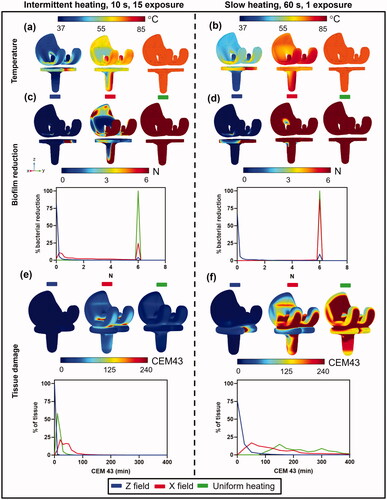
4. Discussion
In this study, the temporal dynamics of biofilm reduction and tissue thermal damage are investigated. Arrhenius models of biofilm reduction with heat were developed based on in vitro experiments on multiple bacterial strains, and these were incorporated into numerical predictions of surface heating of a metal implant using skin effect deposition, as would be achieved with AMF exposure. The study will provide a foundation for suitable treatment parameters using AMF to reduce biofilm.
The importance of skin-effect heating in reducing tissue damage is apparent in . The results demonstrate that rapid skin-effect heating achieves the least amount of tissue damage, however achieving this also requires the strongest magnetic field. The magnetic field is related to the amount of current that can be driven through an AMF device, and practical limits on the magnetic field of >10 mT will dictate the fastest rate of heating that can be achieved. The skin effect heating distribution shown in is similar to what would be achieved with a 200 kHz AMF exposure on an implant with a diameter of 8 cm. The skin effect also varies with frequency, and the effect of varying the frequency from 100 kHz to 1000 kHz on the heating time and radial tissue damage for a 65 °C target is shown in Appendix B. A 50% reduction in tissue damage can be achieved by increasing the frequency from 200 to 1000 kHz. However, at higher frequencies and faster heating, the ability to achieve uniform surface temperature distributions on a complex implant geometry can become a challenge. Our initial simulation and experimental results suggest that 200 kHz is a reasonable choice for AMF heating of implants similar in size and shape to a prosthetic knee joint [Citation40]. Frequencies between 200 and 500 kHz define a clinically relevant range since the alternating electric current does not induce significant tissue heating and action potential firing [Citation41].
The in vitro measurements of biofilm reduction with temperature and time reveal a number of important findings. First and foremost, the results show that biofilm is much less sensitive to heat than eukaryotic cells, making reduction more challenging to achieve without associated tissue damage. Secondly, a large variation was observed in the thermal sensitivity of Gram negative and Gram positive biofilm, with the latter being almost 10× more tolerant to heat. Finally, within a given strain, the thermal sensitivity of drug-sensitive or drug-tolerant biofilm was the same. This last observation has important implications on the potential power of AMF/heating in targeting drug tolerant implant infections. We point out that all the in vitro measurements were made in the absence of any antimicrobial agents. Recent results by our group and others have described the importance of antibiotics in further reducing biofilm in the presence of heating [Citation17,Citation24–27].
The implant heating simulations reveal some important relationships between heating time, surface temperature and spatial uniformity of heating that are critically important to applying this method successfully. For the case of uniform surface heating, the exponential relationship between time, tissue damage and bacterial inactivation leads to increasing tissue damage for slower heating. As an example from , heating the implant at 75 °C results in 6-log10 of S. aureus biofilm reduction in a few seconds, with less than 1 mm of tissue damage. However, reducing the temperature to 65 °C increases the time to achieve the same level of biofilm reduction to almost 3 min, and by this time, there is a 3 mm radius of tissue damage around the implant. The situation of intermittent heating in Figure5 also shows a similar trend. Reduction of a S. aureus biofilm at 65 °C requires 15 exposures, 35 s in duration, or 8 exposures, 60 s in duration. The tissue damage radius for these cases is approximately 3 mm around the implant. Taking into account that the cooldown between exposures can last 5 min in duration, this also results in very long treatment times of an hour or greater. Increasing the peak temperature of each intermittent exposure to 70 °C enables reduction to be achieved in one or two exposures for heating durations of 60 or 35 s respectively, which is more practical from the perspective of treatment. The thermal damage radius in these cases is also reduced to 2 mm, which is an advantage. Finally, for 75 °C, only a single 10 s exposure is sufficient to reduce the implant with 1 mm of tissue damage. The net conclusion from these simulations is that for uniform heating, the highest temperature (>70 °C) in the shortest time (10 s) results in the best balance between full biofilm reduction and safety to surrounding tissues. We point out these results are specific to S. aureus which is the more thermally tolerant strain. For Gram negative strains, reduction can be achieved at 60–65 °C with a few exposures, but the same relative trends are applicable.
One major issue that requires careful consideration for AMF exposure on metal implants is the spatial uniformity of heating. It may be challenging to design and build a coil that can generate uniform heating across the entire implant surface. Segmental heating of the implant [Citation10] and magnetic shielding [Citation42] may be strategies that can reduce the non-uniformity of heating, but perfectly uniform heating is likely unachievable. The final simulations of the study reveal the critical importance of spatial heating uniformity, and the importance of factoring this in when selecting an appropriate exposure time. and evaluate this for a simple spherical implant, and a more complex prosthetic knee. In both cases, very non-uniform heating, perfectly uniform heating and heating with a uniformity that might be achievable with a practical device were evaluated. For the uniform case, the results match what was described in the earlier figure. With 10 s of heating delivered in 15 exposures, or a single 60 s exposure, 100% of the implant surface is reduced of biofilm. However, the slower heating has the additional penalty of an increase in the thermal dose at 3 mm from the implant to irreversible levels. As earlier, with uniform heating, the strategy should be fast heating to high temperatures. In the non-uniform case, neither intermittent nor slow heating can reduce more than 25–30% of the implant surface, with almost an equivalent percentage of the surface area experiencing no reduction in biofilm.
For the moderately uniform heating case (middle column in and ), a distinct benefit of slow heating emerges, whereas the intermittent heating of 15 exposures, 10 s each only can reduce 25% of the implant surface of biofilm, slow heating of 60 s in a single exposure achieves reduction of close to 100% of the surface. However, this comes at a penalty of an increase of thermal damage at 3 mm to irreversible levels. Slow heating achieves a significant improvement in the ability to reduce virtually the whole surface of biofilm, whereas rapid heating is ineffective. This arises because the slower heating gives time for thermal conduction to distribute heat to areas with lower temperature, and results in a generally more uniform distribution on the implant surface.
We point out a number of limitations and shortcomings of this study. Because of the large number of variables that could be investigated, some parameters were kept constant. Perhaps the most important was AMF frequency, which was fixed at 200 kHz. Higher frequencies would have an improved skin-effect heating, but possibly greater non-uniformity, and should be evaluated to determine how the results trend with frequency. Another limitation of this study was the inability to factor in the effect of antibiotics on biofilm reduction, especially for intermittent exposures. We have observed previously, that in between exposures, there can be a strong synergistic response and further reduction of bacteria [Citation24], and this was not factored into the study. The study also assumes a model of biofilm that only exists on the surface of the implant. In reality there will be biofilm on tissue adjacent to the implant surface. Finally, another important limitation in this study is that the water bath produces heating from a different direction than AMF. Also, AMF involves electrical currents on the metal surface, which may have an additional impact on biofilm. The water-bath heating is chosen because of the simplicity and reproducibility of uniform heating. However, our in vitro results [Citation24] suggest that the effect of AMF-induced heating may be stronger.
5. Conclusion
This study determines the AMF and treatment parameters that are important for reducing biofilm while protecting surrounding tissues from thermal damage. Skin effect heating is important for minimizing tissue damage, especially for heating times less than 2 min. Biofilm inactivation with heat follows an Arrhenius relationship across the range studied, but differs greatly among strains, with a factor of ∼10 increase in time for equivalent reduction between S. aureus and P. aeruginosa. For uniform implant heating, the best balance of surface biofilm reduction and tissue safety is achieved with rapid heating (<10 s) to high temperature (>70 °C). However, as the surface heating distribution becomes non-uniform, slower heating is critically important to maintain the same level of biofilm reduction, albeit at a penalty of increased tissue damage. One practical heating scenario for effectively reducing S. aureus biofilm on a knee implant appears to be a target maximum temperature of 75 °C with a single 60-s exposure.
Supplemental Material
Download PDF (372.6 KB)Disclosure statement
Dr. David E. Greenberg and Dr. Rajiv Chopra are inventors of the technology related to AMF, holds patents related to this technology, and are the founders of Solenic Medical. Dr. David E. Greenberg serves as Chief Medical Officer and Dr. Rajiv Chopra serves as Chief Technology Officer at Solenic Medical. No potential conflict of interest was reported by the author(s).
Additional information
Funding
References
- Manivasagam G, Dhinasekaran D, Rajamanickam A. Biomedical implants: corrosion and its prevention - a review. Recent Pat Corros Sci 2010;2(1):713–54.
- U.S. Food & Drug Administration (FDA). Biological responses to metal implants. 2019. https://www.fda.gov/media/131150/download.
- Ribeiro M, Monteiro FJ, Ferraz MP. Infection of orthopedic implants with emphasis on bacterial adhesion process and techniques used in studying bacterial-material interactions. Biomatter. 2012;2(4):176–194.
- Tande AJ, Patel R. Prosthetic joint infection. Clin Microbiol Rev. 2014;27(2):302–345.
- Tayton ER, Frampton C, Hooper GJ, et al. The impact of patient and surgical factors on the rate of infection after primary total knee arthroplasty. Bone Jt J 2016;98-B(3):334–340.
- Pulido L, Ghanem E, Joshi A, et al. Periprosthetic joint infection: the incidence, timing, and predisposing factors. Clin Orthop Relat Res. 2008;466(7):1710–1715.
- Willey M, Karam M. Impact of infection on fracture fixation. Orthop Clin North Am. 2016;47(2):357–364.
- O'Toole A, Ricker EB, Nuxoll E. Thermal mitigation of Pseudomonas aeruginosa biofilms. Biofouling. 2015;31(8):665–675.
- Osmon DR, Berbari EF, Berendt AR, et al. Diagnosis and management of prosthetic joint infection: clinical practice guidelines by the Infectious Diseases Society of America. Clin Infect Dis. 2013;56(1):1–25.
- Pijls BG, Sanders IMJG, Kuijper EJ, et al. Segmental induction heating of orthopaedic metal implants. Bone Jt Res. 2018;7(11):609–619.
- Premkumar A, Kolin DA, Farley KX, et al. Projected economic burden of periprosthetic joint infection of the hip and knee in the United States. J Arthroplasty. 2021;36(5):1484–1489.e3.
- Zilberman M, Elsner JJ. Antibiotic-eluting medical devices for various applications. J Control Release. 2008;130(3):202–215.
- Schmolders J, Hischebeth GTR, Friedrich MJ, et al. Evidence of MRSE on a gentamicin and vancomycin impregnated polymethyl-methacrylate (PMMA) bone cement spacer after two-stage exchange arthroplasty due to periprosthetic joint infection of the knee. BMC Infect. Dis. 2014;14:144.
- Corona PS, Espinal L, Rodríguez-Pardo D, et al. Antibiotic susceptibility in gram-positive chronic joint arthroplasty infections: increased aminoglycoside resistance rate in patients with prior aminoglycoside-impregnated cement spacer use. J Arthroplasty. 2014;29(8):1617–1621.
- Richardson IP, Sturtevant R, Heung M, et al. Hemodialysis catheter heat transfer for biofilm prevention and treatment. Physiol Behav. 2017;176:139–148.
- Ricker EB, Aljaafari HAS, Bader TM, et al. Thermal shock susceptibility and regrowth of Pseudomonas aeruginosa biofilms. Int J Hyperthermia. 2018;34(2):168–176.
- Alumutairi L, Yu B, Filka M, et al. Mild magnetic nanoparticle hyperthermia enhances the susceptibility of Staphylococcus aureus biofilm to antibiotics. Int J Hyperth. 2020;37(1):66–75.
- Chopra R, Shaikh S, Chatzinoff Y, et al. Employing high-frequency alternating magnetic fields for the non-invasive treatment of prosthetic joint infections. Sci Rep. 2017;7:1–14.
- Pijls BG, Sanders IMJG, Kuijper EJ, et al. Non-contact electromagnetic induction heating for eradicating bacteria and yeasts on biomaterials and possible relevance to orthopaedic implant infections: in vitro findings. Bone Jt Res. 2017;6(5):323–330.
- Fang CH, Tsai PI, Huang SW, et al. Magnetic hyperthermia enhance the treatment efficacy of peri-implant osteomyelitis. BMC Infect Dis. 2017;17:1–12.
- Zinn S, SS L. Elements of induction heating: design, control, and applications. ASM Int. 1988;2:9–25.
- Tillotson CL, Rosenberg AE, Rosenthal DI. Controlled thermal injury of bone report of a percutaneous technique using radiofrequency electrode and generator. Invest Radiol. 1989;24(11):888–892.
- Cheng B, Chatzinoff Y, Szczepanski D, et al. Remote acoustic sensing as a safety mechanism during exposure of metal implants to alternating magnetic fields. PLoS One. 2018;13(5):e0197380–20.
- Wang Q, Vachon J, Prasad B, et al. Alternating magnetic fields and antibiotics reduce biofilm on metal in a synergistic fashion. Npj Biofilms Microbiomes. 2021;7(1):1–10.
- Ricker EB, Nuxoll E. Synergistic effects of heat and antibiotics on Pseudomonas aeruginosa biofilms. Biofouling. 2017;33(10):855–866.
- Pijls BG, Sanders IMJG, Kujiper EJ, et al. Induction heating for eradicating Staphylococcus epidermidis from biofilm. Bone Jt Res. 2020;9(4):192–199.
- Pijls BG, Sanders IMJG, Kuijper EJ, et al. Synergy between induction heating, antibiotics, and N-acetylcysteine eradicates Staphylococcus aureus from biofilm. Int J Hyperthermia. 2020;37(1):130–136.
- Laidler KJ. The development of the arrhenius equation. J. Chem. Educ. 1984;61(6):494–498.
- Peleg M, Normand MD, Corradini MG. The arrhenius equation revisited. Crit Rev Food Sci Nutr 2012;52(9):830–851.
- Lambert RJW. A model for the thermal inactivation of micro-organisms. J Appl Microbiol. 2003;95(3):500–507.
- Pennes H. Analysis of tissue and arterial blood temperatures in the resting human forearm. J Appl Physiol. 1948;1(2):93–122.
- Prasad B, Kim JK, Kim S. Role of simulations in the treatment planning of radiofrequency hyperthermia therapy in clinics. J Oncol. 2019;2019:9685476.
- Prasad B, Kim S, Cho W, et al. Quantitative estimation of the equivalent radiation dose escalation using radiofrequency hyperthermia in mouse xenograft models of human lung cancer. Sci Rep. 2019;9(1):3942.
- Prasad B, Kim S, Cho W, et al. Effect of tumor properties on energy absorption, temperature mapping, and thermal dose in 13.56-MHz radiofrequency hyperthermia. J Therm Biol. 2018;74:281–289.
- Sapareto SA, Dewey WC. Thermal dose determination in cancer therapy. Int J Radiat Oncol Biol Phys. 1984;10(6):787–800.
- Seedhom BB, Longton EB, Wright V, et al. Dimensions of the knee. Radiographic and autopsy study of sizes required by a knee prosthesis. Ann Rheum Dis. 1972;31(1):54–58.
- Tsikandylakis G, Mohaddes M, Cnudde P, et al. Head size in primary total hip arthroplasty. EFORT Open Rev. 2018;3(5):225–231.
- Hasgall PA, Di Gennaro F, Baumgartner C, et al. IT’IS Database for thermal and electromagnetic parameters of biological tissues. 2020. https://itis.swiss/database.
- Kok HP, Crezee J, Franken NAP, et al. Quantifying the combined effect of radiation therapy and hyperthermia in terms of equivalent dose distributions. Int J Radiat Oncol Biol Phys. 2014;88(3):739–745.
- Sadaphal V, Prasad B, Kay W, et al. Feasibility of heating metal implants with alternating magnetic fields (AMF) in scaled up models. Int J Hyperthermia. 2022;39(1):81–96.
- Rominiyi O, Vanderlinden A, Clenton SJ, et al. Tumour treating fields therapy for glioblastoma: current advances and future directions. Br J Cancer. 2021;124(4):697–709.
- Stauffer PR, Cetas TC, Fletcher AM, et al. Observations on the use of ferromagnetic implants for inducing hyperthermia. IEEE Trans Biomed Eng. 1984;31(1):76–90.