Abstract
Objective: To verify that the TiO2 nanofilm dip-coated by sol-gel can reduce titanium alloy implants (TAI)’s heat production after microwave diathermy (MD).
Methods: The effect of 40 W and 60 W MD on the titanium alloy substrate coated with TiO2 nanofilm (Experimental Group) and the titanium alloy substrate without film (Control Group) were analyzed in vitro and in vivo. Changes in the skeletal muscle around the implant were evaluated in ex vivo by histology.
Results: After 20 min of MD, in vitro the temperature rise of the titanium substrate was less in the Experimental Group than in the Control Group (40 W: 1.4 °C vs. 2.6 °C, p < .01, 60 W: 2.5 °C vs. 3.7 °C, p < .01) and in vivo, the temperature rise of the muscle tissue adjacent to TAI was lower in the Experimental Group than in the Control Group (40 W: 3.29 °C vs. 4.8 °C, p < .01, 60 W: 4.16 °C vs. 6.52 °C, p < .01). Skeletal muscle thermal injury can be found in the Control Group but not in the Experimental Group.
Conclusion: Sol-gel dip-coated TiO2 nanofilm can reduce the heat production of TAIs under single 40~60 W and continuous 40 W MD and protect the muscle tissue adjacent to the implants against thermal injury caused by irradiation.
1. Introduction
Among all orthopedic diseases requiring rehabilitation treatment, patients with metal implants after internal fixation of limb fractures, joint replacement, and internal fixation of spinal fractures account for a large proportion, and many need high-frequency electrotherapy such as microwave diathermy (MD) to obtain the best rehabilitation effect [Citation1–3]. Microwaves from MD are selectively absorbed in tissues with high water content, such as muscle, and can enhance local blood circulation [Citation4], accelerate metabolism, and promote nerve reproduction [Citation5], thus improving soft tissue tension and pain [Citation6,Citation7], promoting fracture healing [Citation8], and so on. However, traditional physiotherapy does not recommend using MD in areas with metal implants and for patients with pacemakers since microwaves produce heat by affecting metals through various mechanisms, leading to thermal injury to the surrounding tissues [Citation9,Citation10]. The contraindication limits the use of MD after orthopedic implant surgery.
With the development of material science, titanium alloy has gradually replaced stainless steel and other metals in orthopedics. Titanium is a material with low electrical and thermal conductivity, and its heat production under microwave irradiation is significantly lower than that of other metals (such as stainless steel and cobalt-nickel alloy), which allows patients with titanium alloy implant (TAI) to undergo magnetic resonance imaging (MRI) examination. Moreover, we recently found that a single low-dose (20 W∼40 W) 2450 MHz microwave irradiation of the femurs of rabbits fixed with titanium alloy for 15 min increases the temperature of the deep muscles near TAI by 4.50 ± 0.21 °C compared to baseline, with a peak temperature below 42 °C [Citation11]. The follow-up study also found that the low dose (25 W) of 2450 MHz microwave irradiation for one month on the rabbit femur fixed by titanium alloy did not cause thermal injury to the peripheral nerve and muscle tissue [Citation12]. Therefore, we believe that low-dose 2450 MHz MD can be applied to TAIs under experimental animal conditions. However, it is also well known that MD at moderate to high doses can cause thermal injury to the tissue surrounding the implant [Citation13]. Thus, the question is whether there is another way further increase the dose of microwave irradiation while reducing the heat production of TAIs.
Some studies reported on surface modification and coating techniques that have been applied to improve the performance of modern orthopedic materials [Citation14,Citation15]. For example, Hua’s team from Shanghai University designed a TiO2 nanofilm, which was coated on a titanium alloy (Ti6Al4V) substrate by the sol-gel method [Citation16]. In order to verify the thermal resistance effect of the film, we investigated the temperature change of the titanium alloy substrate coated with TiO2 nanofilm after microwave irradiation with medium and high doses in vitro and in vivo, as well as the thermal injury to tissues caused by temperature rise in vivo.
2. Materials and methods
2.1. TAI coated with TiO2 nanofilm
The fabrication and characterization of TAIs dip-coated by the sol-gel method have been reported in a previous study [Citation16]. The coated samples had an average film thickness of 115 nm, an average Ra roughness value of 0.28 nm, a grain size of 47 nm, and an average electric resistivity of 330.02 mΩ compared to 1.35 mΩ for the uncoated samples.
2.2. In vitro experiment
The laboratory air conditioning temperature was set to 25 °C, and the temperature was measured and calibrated with a thermometer. A wooden workbench was placed in the center of the lab, and fixtures in the room that may affect air temperature were removed. An uncoated titanium alloy (Ti6Al4V) substrate (Control Group, n = 5) was placed into tight contact with a sensor of a galvanic thermometer (F-17086, FHC, USA), which was not subject to microwave interference, and a thermally conductive paste (X-23-7921-5, Shin-Etsu Chemical, JAPAN) was fixed. The power switch of the thermometer was turned on for 1 min. Then, when the temperature was stable, the baseline temperature was recorded. The titanium alloy substrate was placed in the center of the workbench with two plastic clamps. A wattmeter (Fantoom 460, Enraf, Netherlands) was used to calibrate the 2450 MHz MD instrument (PM-800, MINATO, JAPAN), and then the circular probe of the MD instrument was placed vertically 10 cm above the titanium alloy substrate, after which the microwave power was turned on. The parameter was adjusted to 40 W, and the irradiation time was set to 20 min (). Record the temperature per minute after starting irradiation. Next, the samples were irradiated with a 60 W microwave, and the temperature changes were recorded. In the Experimental Group (n = 5), the microwave irradiation object was replaced by the Ti6Al4V substrate coated with TiO2 nanofilm, and the other steps were the same.
2.3. Animal model construction
Forty cleaning-grade New Zealand white rabbits weighing 3. 0 ∼ 3.5 kg were selected. They were randomly divided into two groups (20 rabbits in each group: 10 for temperature measurement and histological observation after single 40 W and 60 W microwave irradiation, and another 10 for histological observation and apoptosis detection after continuous daily 40 W microwave irradiation): the Experimental Group and the Control Group. New Zealand rabbits were purchased from Shanghai Songjiang District Songlian Laboratory Animal Corporation (production license: SCXK (Shanghai) 2017-0008) and raised in the animal laboratory of our hospital. After one week of feeding and adaptation to the environment, animal experiments started, and animal models were constructed in the special operating room. All the animals were housed in an environment with a temperature of 22 ± 1 °C, a relative humidity of 50 ± 1%, and a light/dark cycle of 12/12 h. All animal studies (including the euthanasia procedure) were done in compliance with the regulations and guidelines of Shanghai Jiao Tong University institutional animal care and conducted according to the AAALAC and the IACUC guidelines.
An animal model of femoral shaft fracture internal fixation in rabbits was established following a previous protocol [Citation12]. Rabbits were anesthetized intravenously through the ear margin (pentobarbital sodium 30 mg/kg), and then the skin of the right lateral thigh was incised and bluntly separated layer by layer to expose the middle femur. The Control Group was implanted with a 380 mm × 42 mm titanium alloy plate without TiO2 nanofilm, while the Experimental Group received a titanium alloy plate covered with TiO2 nanofilm. A stainless-steel wire saw was used to cut the femur at the midpoint of the plate to a depth of approximately 3 mm, after which the wound was irrigated and sutured. A postoperative X-ray was used to determine the position and stability of the plate (). Penicillin (800,000 units/day) was administered continuously intramuscularly for three days postoperatively. All New Zealand rabbits were euthanized at the end of the microwave heating experiment by the overdose anesthesia method.
2.4. Microwave irradiation and temperature measurement
Four days after the operation, 40 W and 60 W microwave irradiation were performed on the two groups, and the temperature of the implants and the temperature of the deep muscle tissue adjacent to the implants were measured. The temperature of the air conditioner in the laboratory was adjusted to 25 °C, and the temperature was measured and calibrated with a thermometer. New Zealand rabbits were still anesthetized by ear vein. The original skin incision was cut to expose the implant, and the temperature sensor (F-17086, FHC, USA), which was not interfered by microwave, was closely contacted with the middle of the implant. The initial temperature was recorded when the temperature reading was stable. Next, a 2450 MHz microwave probe (PM-800, MINATO, JAPAN) was placed 10 cm above the wound (), and the microwave irradiation power was set at 40 W for 20 min, after which the irradiation started, and the time and the corresponding temperature readings were continuously recorded in minutes. After 20 min, the microwave power was automatically turned off. After 30 min, the temperature reading returned to the initial temperature, and then the temperature sensor was placed in the deep muscle tissue 5 mm above the middle surface of the implant and the 40 W microwave was irradiated again for 20 min; the temperature change per minute was recorded. The test procedures of 60 W microwave irradiation and 40 W microwave irradiation were the same.
2.5. Histopathology of skeletal muscle adjacent to the implant
Histopathology was observed by light microscopy and transmission electron microscopy. Skeletal muscle slides for gross histology were prepared as follows: after irradiation, muscle tissue above the titanium alloy (< 0.5 cm thick) was collected and fixed using 4% paraformaldehyde for 24 h rinsed, dehydrated, waxed, embedded, and sectioned. For HE staining, the sections were processed according to the steps of dewaxing, rehydration, staining nuclei, anti-blue, staining cytoplasm, dehydration, transparency, and sealing. After sealing the sections, the sections were observed and photographed using an optical microscope (BX53, OLYMPUS, JAPAN).
Micro-histological sections of skeletal muscle were produced as follows: after microwave irradiation, muscle tissue block (1 mm3) was obtained from the top of the implant, fixed with 2. 5% glutaraldehyde and phosphate buffer, rinsed, dehydrated with gradient alcohol, embedded with pure acetone and epoxy resin, solidified, sectioned and double stained with 3% uranyl acetate and lead citrate. Sections were observed under a transmission electron microscope (JEM-1200EX, JEOL, JAPAN).
2.6. Tunel staining for apoptosis
The Experimental and Control groups received microwave irradiation at 40 W (once a day for 20 min). Muscle tissue adjacent to the implant was taken at 14 days and 30 days, fixed in 4% paraformaldehyde for 24 h. Tissue sections were prepared according to the same procedure as above, followed by TUNEL staining according to the method of Majtnerová et al. [Citation17]. Finally, the muscle tissue specimens were observed, photographed and recorded using a light microscope with five different fields of view randomly selected. The percentage of positive cells per 100 nuclei in each sample was calculated as the apoptosis rate.
2.7. Statistical analysis
GraphPad Prism 8.0.2 (GraphPad Software, USA) and SPSS 19 (IBM, USA) were used for statistical analysis. Measurement data were expressed as mean ± SD, and multiple t-tests was used to analyze the temperature difference between the two groups at each time point. A chi-square test was used to compare the apoptosis rates of the two groups. A p value < .05 (both sides) was considered to be statistically significant.
3. Results
3.1. In vitro experiment
During 40 W microwave irradiation, the temperature of the Control Group and the Experimental Group increased rapidly in the first 10 min and 8 min, respectively; the temperature increase rate was higher in the Control Group vs. Experimental Group (0.21 °C/s vs. 0.10 °C/s). Both groups reached the endpoint temperature after 20 min irradiation. The comparison of the mean endpoint temperature between the two groups showed that the temperature in the Experimental Group increased by 1.4 °C while in the Control Group, it increased by 2.6 °C; the difference was statistically significant (p < .01) ().
Figure 4. Temperature changes of the Experimental Group and the Control Group after 2450 MHz microwave irradiation in vitro. Intra-group comparisons showed that the rate of temperature rise was faster when samples were irradiated with 60 W microwave compared to 40 W microwave, and the rate of temperature rise slowed down over time. The difference between the Experimental Group and the Control Group was statistically significant from the second minute after 40 W microwave irradiation (a) and from the third minute after 60 W microwave irradiation (b). *p < .05.
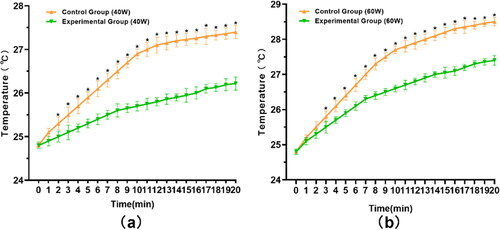
During 60 W microwave irradiation, the temperature of the Control Group and the Experimental Group increased rapidly in the first 8 min and 7 min, respectively; the temperature increase rate was higher in the Control Group than in the Experimental Group (0.31 °C/s vs. 0.21 °C/s). Both groups reached the endpoint temperature after 20 min irradiation. The comparison of the mean endpoint temperature between the two groups showed that the temperature in the Experimental Group increased by 2.5 °C, while the Control Group increased by 3.7 °C, and the difference was statistically significant (p < .01) ().
3.2. In vivo experiment
After 40 W and 60 W microwave irradiation, compared to baseline physiological temperature(35.9∼37.1 °C), the temperature of TAI and deep muscle tissue increased significantly in the Experimental Group and the Control Group (all p < .01).
Under 40 W microwave irradiation, the endpoint temperature of the implants in the Experimental Group was 40.72 ± 0.33 °C, with an average increase of 4.19 °C, while the endpoint temperature of the implants in the Control Group was 42.63 ± 0.42 °C, with an average increase of 6.13 °C (p < .01). The endpoint temperature of deep muscle in the Experimental Group was 39.99 ± 0.37 °C, with an average increase of 3.29 °C, and the value of CEM 43 °C was 0.08 min. while the endpoint temperature of deep muscle in the Control Group was 41.55 ± 0.41 °C, with an average increase of 4.8 °C (p < .01) (), and the value of CEM 43 °C was 0.25 min.
Figure 5. Temperature changes of TAI and deep muscle tissue under 40 W and 60 W microwave irradiation. (a) When the microwave power was 40 W, compared with the Control Group, the temperature of TAI in the Experimental Group began to show a statistical difference from the fourth minute (p < .05). The temperature of deep muscle was statistically different from the seventh minute (p < .05). (b) When exposed to a 60 W microwave. compared with the Control Group, the temperature of TAI in the Experimental Group began to show a significant difference from the fourth minute (p < .05). There was a significant difference in deep muscle temperature from the third minute (p < .05). *Experimental Group-TAI vs. Experimental Group-DMU, #Control Group-TAI vs. Control Group-DMU, &Experimental Group-DMU vs. Control Group-DMU, δExperimental Group-TAI vs. Control Group-TAI. TAI: titanium alloy implant, DMU: deep muscle.
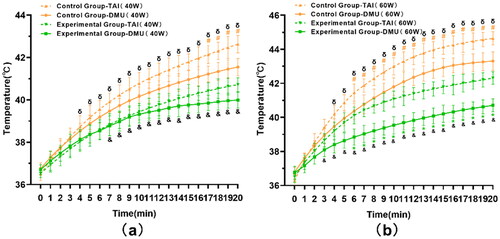
Under 60 W microwave irradiation, the endpoint temperature of implants in the Experimental Group was 42.31 ± 0.37 °C, with an average increase of 5.7 °C, while the endpoint temperature of implants in the Control Group was 44.65 ± 0.39 °C, with an average increase of 8.35 °C (p < .01). The endpoint temperature of deep muscle in the Experimental Group was 40.71 ± 0.41 °C, with an average increase of 4.16 °C, and the value of CEM 43 °C was 0.12 min, while the endpoint temperature of deep muscle in the Control Group was 43.31 ± 0.55 °C, with an average increase of 6.52 °C (p < .01), and the value of CEM 43 °C was 1.75 min. Overall, the endpoint temperature of TAI and deep muscle tissue in the Experimental Group was lower than that in the Control Group (p < .01) ().
3.3. Histopathology
3.3.1. Optical microscope
There was no injury to the adjacent skeletal muscle tissue of the implants in the Experimental Group and the Control Group when irradiated with a 40 W microwave.
Under 60 W microwave irradiation, there was no injury to the skeletal muscle tissue in the Experimental Group under the optical microscope; yet, congestion in the intercellular space of muscle cells, loose and disordered muscle fibers, and broken myofibrils were seen in the Control Group, which suggests that TiO2 nanofilm can protect the adjacent tissue of the implant from thermal damage when exposed to 60 W microwave ().
Figure 6. Histopathological changes of skeletal muscle adjacent to implants after 40 W and 60 W single microwave irradiation and continuous 40 W microwave irradiation (optical microscope). Black arrow: intermuscular bleeding, white arrow: myofibril disruption (a) and muscle fiber disorder (b).
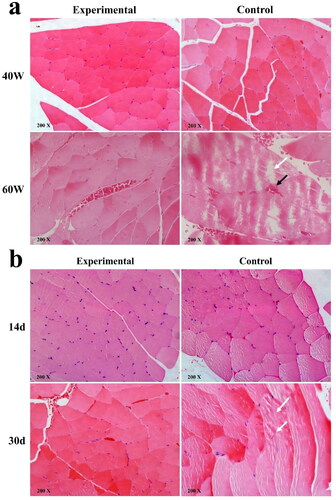
There were no significant histological changes in the Experimental Group at 14 days and 30 days when irradiated daily with 40 W microwaves, while the Control Group had slightly disturbed muscle fibers. This suggests that there may have been mild damage in the Control Group after continuous microwave irradiation ().
3.3.2. Transmission electron microscope
The skeletal muscle of the Control Group was damaged when exposed to 40 W and 60 W microwave. Mitochondria in the skeletal muscle increased significantly and were swollen when exposed to 40 W microwave irradiation. When exposed to 60 W microwave irradiation, the sarcomere of the skeletal muscle was disordered and partially broken. Yet, these changes were not seen in the Experimental Group (), which further confirmed the results seen above (optical microscope).
3.4. Apoptosis
The apoptosis of muscle tissue after continuous daily irradiation with 40 W microwave is shown in . The apoptosis rate of muscle cells in the Experimental Group at two weeks and four weeks microwave irradiation was significantly lower than that of the Control Group, indicating that the thermal damage to the muscle tissue adjacent to the implant was less in the Experimental Group. shows the results of the muscle TUNEL assay and shows the apoptotic cell count analysis.
4. Discussion
The metal implant is one of the contraindications of MD, which limits the application of MD in patients with orthopedic implants. However, a recent study has shown that low-dose microwave irradiation of titanium alloy internal fixation implants did not produce enough heat to damage the soft tissue around the implant [Citation13]. Ways to increase the dose of microwave irradiation through nanofilm technology are emerging.
TiO2 films have been used as thermal insulation materials due to their extremely low thermal conductivity [Citation18,Citation19]. In this study, in vitro and in vivo experiments were carried out to verify whether TiO2 nanofilm helps reduce the heat production of TAI exposed to MD. We found that when TAI coated with TiO2 film was irradiated by microwave at medium and high doses, the endpoint temperature of the implant was lower than that of the Control Group without film, and there was no thermal injury to the adjacent skeletal muscle tissue.
4.1. Heat production mechanism of microwave irradiated metals
A microwave is a high-frequency electromagnetic wave, including dielectric heating, Joule heating, and induction heating. The heating results depend on the characteristics of microwave and metal materials [Citation20,Citation21]. The electric field component of the microwave is responsible for dielectric heating, and the magnetic field component of the microwave is responsible for Joule heating and induction heating. The main mechanisms of microwave magnetic field heating include eddy current loss, hysteresis loss, magnetic resonance loss, and residual loss [Citation22]. Among them, eddy current loss and hysteresis loss are the main mechanisms.
Eddy current losses are Joule losses caused by eddy currents induced by an alternating magnetic field. As long as there is relative motion between the conductive material and the external magnetic field, eddy currents are generated [Citation23]. From the point of view of Joule heating due to an alternating magnetic field, the eddy current losses depend strongly on the material resistivity. In general, eddy current loss is the main mechanism for microwave heating of various conductor and semiconductor materials [Citation24,Citation25]. Hysteresis loss is caused by the irreversible magnetization process in an alternating magnetic field and is the energy consumed by a ferromagnet or the like in the process of repeated magnetization. Therefore, hysteresis loss occurs only in magnetic materials, such as iron, steel, nickel, and some other metals. When a magnetic metal is subjected to an alternating magnetic field, the magnetic dipole oscillates as the magnetic pole changes its polarity direction at each cycle [Citation26]. This rapid switching of the magnetic domains causes considerable friction and heating inside the material. The production of heat due to this oscillating mechanism is called hysteresis loss. Hysteresis losses contribute greatly to heating ferromagnetic materials in an alternating magnetic field. The commonly used TAI are non-magnetic or low-magnetic, so the eddy current loss is the main mechanism of heat production when irradiated by microwave.
4.2. Possible mechanisms for the thermal barrier effect of TiO2 nanofilm
In this study, the surface temperature of titanium alloy coated with TiO2 film was significantly lower than that of the Control Group under medium and high doses of microwave irradiation. This shows that the TiO2 nanofilm can act as a thermal barrier in the microwave field. We speculate that this is related to the electrical properties of TiO2 nanofilm. The microwave first encounters the film and undergoes reflection, transmission, and absorption. The energy conversion analysis is as follows (): (1) the TiO2 nanofilm will reflect the microwave, and this part of microwave energy will not be converted into heat energy on the surface of the film. (2) Because the thickness of the TiO2 nanofilm is 115 nm [Citation16] and the penetration depth of the microwave to the metal is generally at the micron level, the microwave can penetrate the nanofilm to reach the titanium alloy substrate, but the transmitted microwave energy will be reduced, so the eddy current heat production on the surface of the titanium alloy substrate will be relatively reduced (compared with the uncoated film). (3) The average resistance of titanium alloy coated with TiO2 film increased to 330.02 mΩ, which is 200 times higher than that of the uncoated titanium alloy [Citation16]. As a result, the microwave easily penetrates the film, and the microwave absorptivity of the film is significantly lower than that of the titanium alloy substrate, so the absorption heat of the film is lower than that of the titanium alloy substrate. (4) The physical barrier of the nanofilm will partially prevent heat transfer from the internal titanium alloy substrate to the surface of the film. Therefore, TiO2 nanofilm may act as ‘greenhouse glass’, thus avoiding the rapid increase of the surface temperature of the film after microwave irradiation.
4.3. Relationship between thermal dose and thermal injury
The thermal dose CEM43 °C is a widely used metric that converts all thermal exposures to ‘equivalent minutes’ at 43 °C, allowing for comparison of the thermal doses of different temperatures and duration [Citation27]. Although some methods that appear superior to CEM43 °C are beginning to emerge [Citation28,Citation29], CEM43 °C is still considered to be the most commonly used method for evaluating thermal dose. Although breakpoint and threshold for thermal injury to muscle tissue between different species are still being explored [Citation30], CEM43 °C appears to be an accurate predictor of thermal injury to tissues for thermal damage caused by tissue temperatures between 39 °C and 57 °C [Citation31]. Previous studies have used a CEM43 °C value located at 41–80 min as a threshold for mild muscle damage [Citation30], but in this study, histologically muscle damage was observed in a control group with a CEM43 °C of 1.75 min in deep muscle, which differs significantly from previous studies. We speculate that in the environment where the implant is present, the implant may reflect electromagnetic waves, causing the muscle tissue to receive secondary exposure, while the thermal conductivity of the implant is responsible for the muscle damage observed at lower CEM43 °C values. However, this is similar to the study by Nadobny et al. [Citation32] where the thermal injury was found to occur to muscle tissue in pigs at a CEM43 °C value of 1.1 min, and further research is required in the future.
4.4. Study limitations
No experiments were performed on a cellular level in vitro, including the biological safety of the films and the effect on cell adhesion. Secondly, the heat produced by microwave irradiation of metal implants is also related to many factors (such as implant shape, thickness, film thickness, roughness, microwave irradiation angle, irradiation distance, frequency, body tissue structure, etc.). This study was limited to the size of the animal femur and did not verify the influence of all parameters on temperature.
5. Conclusions
Our data showed that the TiO2 nanofilm coated by the sol-gel method could reduce the heat production of TAIs under single 40∼60 W and continuous 40 W microwave irradiation. Sol-gel dip-coated TiO2 film could protect the muscle tissue adjacent to the implants against thermal injury caused by irradiation, thus having potential application value for patients with orthopedic metal implants that undergo MD. Future studies should optimize the microstructure (such as anatase, rutile, and brookite) and inherent characteristics of TiO2 nanofilm to achieve a better thermal barrier effect. In addition, the biological safety of TiO2 nanofilm and the thermal barrier effect under different conditions need to be further studied.
Acknowledgments
We would like to express our gratitude to Shiqi Liu of Shanghai University for her help with in vitro study.
Disclosure statement
No potential conflict of interest was reported by the author(s).
Data availability statement
The data that support the findings of this study are available from the corresponding author Yiming Xu upon reasonable request.
Additional information
Funding
References
- Levy O, Schindler A, Chechik A, et al. A complication of internal fixation rods in sport. Br J Sports Med. 1993;27(1):36.
- Gardner RC. A complication of excessive compression and rigid internal fixation. J Trauma. 1972;12(6):534–536.
- SooHoo NF, Krenek L, Eagan MJ, et al. Complication rates following open reduction and internal fixation of ankle fractures. J Bone Joint Surg Am. 2009;91(5):1042–1049.
- Lehmann JF, Dundore DE, Esselman PC, et al. Microwave diathermy: effects on experimental muscle hematoma resolution. Arch Phys Med Rehabil. 1983;64(3):127–129.
- Fu T, Lineaweaver WC, Zhang F, et al. Role of shortwave and microwave diathermy in peripheral neuropathy. J Int Med Res. 2019;47(8):3569–3579.
- Durmus D, Ulus Y, Alayli G, et al. Does microwave diathermy have an effect on clinical parameters in chronic low back pain? A randomized-controlled trial. J Back Musculoskelet Rehabil. 2014;27(4):435–443.
- Andrade Ortega JA, Cerón Fernández E, García Llorent R, et al. Microwave diathermy for treating nonspecific chronic neck pain: a randomized controlled trial. Spine J. 2014;14(8):1712–1721.
- Maiettini D, De Angelis V, Graziosi L, et al. Sacrum colon-rectal cancer metastasis: microwave ablation for palliative pain treatment. Recenti Prog Med. 2016;107(12):673–676.
- Goats GC. Microwave diathermy. Br J Sports Med. 1990;24(4):212–218.
- Xu Y, Hua Z, Cai Y, et al. Study on TiO nanofilm that reduces the heat production of titanium alloy implant in microwave irradiation and does not affect fracture healing. Dis Markers. 2022;2022:4910731.
- Ye D, Xu Y, Fu T, et al. Low dose of continuous-wave microwave irradiation did not cause temperature increase in muscles tissue adjacent to titanium alloy implants–an animal study. BMC Musculoskelet Disord. 2013;14:364–364.
- Ye D, Xu Y, Zhang H, et al. Effects of low-dose microwave on healing of fractures with titanium alloy internal fixation: an experimental study in a rabbit model. PLoS One. 2013;8(9):e75756.
- Ye D, Xu Y, Wang G, et al. Thermal effects of 2450 MHz microwave exposure near a titanium alloy plate implanted in rabbit limbs. Bioelectromagnetics. 2015;36(4):309–318.
- Hua ZK, Sang RZ, Zhang JH. Micro and nano-scale surface texturing: an application in ceramic-on-ceramic artificial joint materials. Nanosci Nanotechnol Lett. 2012;4(9):879–882.
- Cao HL, Liu XY. Activating titanium oxide coatings for orthopedic implants. Surf Coat Tech. 2013;233:57–64.
- Hua Z, Tang F, Bai Y, et al. A titanium dioxide nano film encapsulation for metal orthopedic implants in diathermy rehabilitation therapy. Nanosci Nanotechnol Lett. 2015;7(3):268–271.
- Majtnerová P, Roušar T. An overview of apoptosis assays detecting DNA fragmentation. Mol Biol Rep. 2018;45(5):1469–1478.
- Bao Y, Guo R, Ma J. Hierarchical flower-like hollow SiO@TiO spheres with enhanced thermal insulation and ultraviolet resistance performances for building coating. ACS Appl Mater Interfaces. 2020;12(21):24250–24261.
- Liu T, Liu B, Wang J, et al. Smart window coating based on F-TiO2-KxWO3 nanocomposites with heat shielding, ultraviolet isolating, hydrophilic and photocatalytic performance. Sci Rep. 2016;6:27373.
- Metaxas AC, Meredith RJ. Industrial microwave heating. London, England: Institution of Engineering and Technology; 1988.
- Crane CA, Pantoya ML, Weeks BL. Spatial observation and quantification of microwave heating in materials. Rev Sci Instrum. 2013;84(8):084705.
- Sun J, Wang W, Yue Q. Review on microwave-matter interaction fundamentals and efficient microwave-associated heating strategies. Materials. 2016;9(4):231.
- Aguiar PM, Jacquinot JF, Sakellariou D. Experimental and numerical examination of eddy (Foucault) currents in rotating micro-coils: generation of heat and its impact on sample temperature. J Magn Reson. 2009;200(1):6–14.
- Roy R, Peelamedu R, Grimes C, et al. Major phase transformations and magnetic property changes caused by electromagnetic fields at microwave frequencies. J Mater Res. 2002;17(12):3008–3011.
- Cao Z, Wang Z, Yoshikawa N, et al. Microwave heating origination and rapid crystallization of PZT thin films in separated H field. J Phys D-Appl Phys. 2008;41(9):4.
- Sugumaran PJ, Yang Y, Wang Y, et al. Influence of the aspect ratio of iron oxide nanorods on hysteresis-loss-mediated magnetic hyperthermia. ACS Appl Bio Mater. 2021;4(6):4809–4820.
- Sapareto SA, Dewey WC. Thermal dose determination in cancer therapy. Int J Radiat Oncol Biol Phys. 1984;10(6):787–800.
- Pearce JA, Petryk AA, Hoopes PJ. Numerical model study of in vivo magnetic nanoparticle tumor heating. IEEE Trans Biomed Eng. 2017;64(12):2813–2823.
- Labavić D, Ladjimi MT, Courtade E, et al. Mammalian cell sensitivity to hyperthermia in various cell lines: a new universal and predictive description. Int J Hyperthermia. 2020;37(1):506–516.
- Yarmolenko PS, Moon EJ, Landon C, et al. Thresholds for thermal damage to normal tissues: an update. Int J Hyperthermia. 2011;27(4):320–343.
- Dewhirst MW, Viglianti BL, Lora-Michiels M, et al. Basic principles of thermal dosimetry and thermal thresholds for tissue damage from hyperthermia. Int J Hyperthermia. 2003;19(3):267–294.
- Nadobny J, Klopfleisch R, Brinker G, et al. Experimental investigation and histopathological identification of acute thermal damage in skeletal porcine muscle in relation to whole-body SAR, maximum temperature, and CEM43 °C due to RF irradiation in an MR body coil of birdcage type at 123 MHz. Int J Hyperthermia. 2015;31(4):409–420.