Abstract
Purpose
Dentin hypersensitivity (DH) is a prevalent condition, but long-term effective treatments are scarce. Differentiation of odontoblast-like cells is promising for inducing tertiary dentinogenesis and ensuring sustained therapeutic efficacy against DH. This study examined the effects and mechanism of action of mild heat stress (MHS) on the differentiation of odontoblast-like MDPC-23 cells.
Methods
We used a heating device to accurately control the temperature and duration, mimicking the thermal microenvironment of odontoblast-like cells. Using this device, the effects of MHS on cell viability and differentiation were examined. Cell viability was assessed using the MTT assay. The expression and nucleoplasmic ratio of the yes-associated protein (YAP) were examined by western blotting and immunofluorescence. The gene expression levels of heat shock proteins (HSPs) and dentin matrix protein-1 (DMP1) were measured using qPCR. Dentin sialophosphoprotein (DSPP) expression was evaluated using immunofluorescence and immunoblotting. Verteporfin was used to inhibit YAP activity.
Results
Mild heat stress (MHS) enhanced the odontoblast differentiation of MDPC-23 cells while maintaining cell viability. MHS also increased YAP activity, as well as the levels of HSP25 mRNA, HSP70 mRNA, HSP90α mRNA, DMP1 mRNA, and DSPP protein. However, after YAP inhibition, both cell viability and the levels of HSP90α mRNA, DMP1 mRNA, and DSPP protein were reduced.
Conclusion
YAP plays a crucial role in maintaining cell viability and promoting odontoblast differentiation of MDPC-23 cells under MHS. Consequently, MHS is a potential therapeutic strategy for DH, and boosting YAP activity could be beneficial for maintaining cell viability and promoting odontoblast differentiation.
Introduction
Dentin hypersensitivity (DH) is a common clinical condition characterized by transient acute pain in response to non-harmful stimuli on the exposed dentin, which is distinct from other dental defects or diseases. Despite the prevalence of DH, effective long-term treatments for this condition are lacking [Citation1,Citation2]. Desensitization therapy, which temporarily relieves pain by blocking the dentinal tubules or interrupting nerve impulses, is the most common noninvasive treatment for DH. However, the long-term efficacy of this therapy is questionable, as maintaining blockages in the dentinal tubules can be challenging [Citation3]. Therefore, there is a need for therapies that promote the formation of tertiary and intratubular dentin to provide long-term curative effects [Citation4]. Despite extensive research, conventional indirect pulp-capping materials and bioactive molecules have limited clinical applications [Citation3–5]. Indirect pulp capping requires additional space for restoration and fails to induce the formation of regular dentin, whereas the use of bioactive molecules presents challenges in preserving biological activity and ensuring effective drug delivery [Citation5]. The differentiation of odontoblast-like cells is crucial for the formation of tertiary and intratubular dentin [Citation6], which is key to the long-term effective treatment of DH [Citation7]. Therefore, exploring easily accessible therapies that promote odontoblast differentiation is crucial.
Heat stress, commonly encountered in the oral cavity through hot meals or high-energy dental instruments, is a common stimulus that affects teeth [Citation8]. Heat transfer in teeth is a time-dependent process; dentin and enamel reach a temperature similar to that of the external environment after being placed in a precisely designed heating device for 300 s [Citation9]. Severe heat stress occurs when the temperature inside the pulp cavity increases by over 5.5 °C, leading to irreversible damage to the dental pulp [Citation8,Citation10]. In contrast, mild heat stress (MHS, 38–42 °C) benefits cells and tissues [Citation11,Citation12]. MHS promotes the proliferation and osteogenic differentiation of dental follicle stem cells (DFSCs) [Citation13] and enhances the thermotolerance and differentiation of odontoblast-lineage KN-3 cells [Citation8]. Thermal therapy has garnered interest in tissue engineering and regenerative medicine [Citation12,Citation14,Citation15]. This therapy uses heat stress to regulate cellular events and promote tissue regeneration [Citation12]. Hence, MHS exhibits promise as a viable long-term treatment for DH. However, the impact of various MHS modalities on cell viability, differentiation of odontoblast-like cells, and the pertinent molecular mechanisms underlying the heat stress response remain ambiguous, thereby impeding its clinical applicability.
Yes-associated protein (YAP) is a key mediator in the Hippo signaling pathway and an important thermosensor [Citation16]. The nuclear translocation of YAP is promoted by MHS [Citation17]. YAP translates extracellular heat stress signals into intracellular responses, thereby regulating cell growth and survival [Citation16,Citation17]. YAP promotes the differentiation and mineralization of cementoblasts by upregulating dentin sialophosphoprotein (DSPP) and dentin matrix protein-1 (DMP1) [Citation18]. DSPP and DMP1 are considered markers of odontoblast differentiation [Citation19]. However, the effect of MHS on the viability and differentiation of odontoblast-like cells through YAP remains to be determined.
Heat shock proteins (HSPs) play significant roles in cellular responses to heat stress, including the maintenance of cell homeostasis and the promotion of differentiation [Citation20]. Based on their molecular weight, HSPs are classified into six groups: HSP100, HSP90, HSP70, HSP60, HSP40, and other small HSPs [Citation21]. KN-3 cells, which are odontoblast precursor cells, have been shown to enhance the adaptation and tolerance to heat stress by upregulating HSP90, HSP70, and HSP25 [Citation8,Citation22]. The HSP70-HSP90 chaperone cascade, a major conserved chaperone cascade involved in protein folding, plays a significant role in inhibiting apoptosis and maintaining protein homeostasis, enabling cells to withstand severe heat stress [Citation8,Citation23]. HSP25, a member of the small HSPs family, prevents apoptosis and acts as a switch between the differentiation and proliferation of odontoblasts [Citation24]. Interactions between HSPs and YAP have also been observed in cancer cells [Citation17,Citation25,Citation26]. However, the roles of HSPs and YAP in the heat shock response of odontoblast-like cells and their relationship in these processes remain unclear.
In this study, we investigated the role of MHS in the differentiation of odontoblast-like MDPC-23 cells. Furthermore, given the role of YAP in thermosensing, we assessed the interaction between YAP and HSPs in mediating the effects of MHS on the viability and odontoblast differentiation of MDPC-23 cells.
Materials and methods
Cell culture
MDPC-23 cells (immortalized mouse odontoblast-like cells) were generously provided by Prof. Franklin R. Tay of Augusta University. The cells were maintained in alpha modification of Eagles medium (α-MEM, Gibco, USA) supplemented with 10% fetal bovine serum (FBS, Gibco, USA) and 1% Penicillin-Streptomycin (Solarbio, China) at 37 °C in a humidified incubator with 5% CO2. The cells were passaged every 2 days.
Construction of the heat stress loading model
MDPC-23 cells were cultured on culture dishes up to 70–85% confluence. The culture dishes were then placed in block heaters (Coyote, China) in a cell incubator. Based on previous studies [Citation9,Citation17], a heating time of 15 min and a temperature of 41 °C were chosen for the short-term heat stress experiments. To maintain consistency, the block heaters were precisely calibrated to raise the temperature of the cell culture medium from 37 °C to the targeted 41 °C within 15 min. Subsequently, the temperature was maintained at 41 °C. Two groups were established: the dynamic-MHS group, where the cells were heated from 37 to 41 °C in 15 min, and the mixed-MHS group, where the cells were heated from 37 to 41 °C in 15 min, followed by an additional 15 min of incubation at 41 °C (). After the heating period, cells in both groups were allowed to cool naturally within the cell incubator until an ambient temperature of 37 °C was reached. Control samples were incubated at 37 °C on block heaters for either 15 or 30 min. The cells were exposed to heat treatment every 12 h for 2 days.
Figure 1. Mild heat stress (MHS) affects cell viability and YAP activity. (a) Schematic representation of the heating apparatus. MDPC-23 cells were heated using block heaters within a cell incubator, while a thermocouple monitored the temperature of the cell medium. (b) Real-time temperature recording in the cell culture medium via a thermocouple. Approximately 15 min were required to reach an equilibrium temperature, sustaining stability thereafter. (c) The cell area of MDPC-23 cells under MHS. (d) The viability of MDPC-23 cells under MHS. (e) The gene expression of HSP70 under MHS. (f) The gene expression of HSP90α under MHS. (g) Representative images of immunostained YAP under dynamic MHS. Scale bar, 10 μm. (h) Representative images of immunostained YAP under mixed MHS. Scale bar, 10 μm. (i) Quantification of the nucleo-cytoplasmic ratio in (g). (j) The relative protein levels of pYAP and YAP in MDPC-23 cells under dynamic MHS determined by Western blotting. (k) Quantification of the nucleo-cytoplasmic ratio in (h). (l) The relative protein levels of pYAP and YAP in MDPC-23 cells under mixed MHS determined by Western blotting. (Mean ± SD. ns, no statistically significant difference; *p < 0.05; **p < 0.01; ****p < 0.0001).
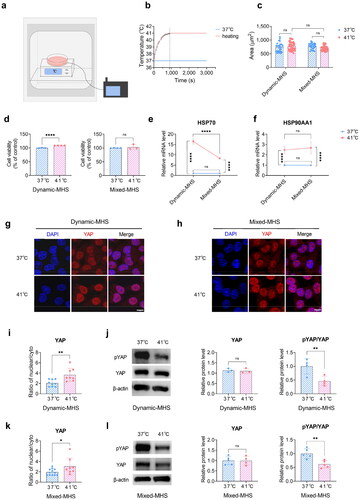
Methyl-thiazol-tetrazolium assay
Cell viability was assessed using the methyl-thiazol-tetrazolium (MTT) assay. MDPC-23 cells were seeded in culture dishes and subjected to thermal or drug treatments. Subsequently, 200 μL of MTT solution (5 mg/mL, Beyotime, China) was added to each culture dish. After 4 h of incubation, 1.5 ml of dimethyl sulfoxide (DMSO) was added to each dish to dissolve the formazan crystals. The absorbance of the resulting solution was measured at 490 nm using a microplate reader and standardized using the reference values.
Quantitative qPCR
Total RNA was extracted using RNA Extraction Kits (Accurate Biology, China) following the manufacturer’s instructions. cDNA was synthesized using the PrimeScript™ RT Master Mix (Takara, Japan). Real-time PCR was performed using SYBR Green (Accurate Biology, China) on a quantitative PCR machine (CFX96 Touch, Bio-Rad, USA). GAPDH was used as the reference gene. Data were analyzed using the 2−ΔΔCt method.
The following primer sequences were used:
mouse HSP25, forward 5′-ATCACTGGCAAGCACGAAGA-3′ and reverse 5′-GGCCTCGAAAGTAACCGGAA-3′;
mouse HSP70*, forward 5′-ACAAGTGCCAGGAGGTCATCTC-3′ and reverse 5′- TCTAATCCACCTCCTCGATGGTG-3′;
mouse HSP90AA1*, forward 5′-AATGCTTAGAACTATTTACTGAACTAGCAGAA-3′ and reverse 5′-GTCCTCGTGAATTCCAAGCTTT-3′;
mouse DMP1, forward 5′-TCAGGACAGTAGCCGATCCA-3′ and reverse 5′-CCGTCCTGTGAGAGCCATTT-3′;
mouse GAPDH, forward 5′-GGTGAAGGTCGGTGTGAACG-3′ and reverse 5′- CTCGCTCCTGGAAGATGGTG-3′;
Immunofluorescence staining
For immunofluorescence detection of YAP and DSPP, MDPC-23 cells were cultured in 35 mm confocal dishes (Beyotime, China) under heat stress. After heating, cells were fixed in 4% paraformaldehyde for 20 min, permeabilized with 0.5% Triton X-100 for 10 min, and blocked in 5% bovine serum albumin (BSA) for 30 min at room temperature. Samples were then incubated with rabbit anti-YAP primary antibody (14074S, 1:200; Cell Signaling Technology, USA) or mouse anti-DSPP primary antibody (sc-73632, 1:200; Santa Cruz, USA) in an antibody diluent at 4 °C overnight. Following primary antibody incubation, the cells were incubated with FITC-conjugated goat anti-mouse secondary antibody (BA1101, 1:200; Boster, China) or CY3-conjugated goat anti-rabbit secondary antibody (BA1032, 1:200; Boster, China) for 2 h at room temperature. Nuclei were counterstained with DAPI Staining Solution (Boster, China). Fluorescence images were captured using a FLUOVIEW FV3000 microscope (Olympus, Japan) and analyzed using the ImageJ software. The mean optical density was calculated using 2–4 representative regions of each sample.
Drug treatment
Verteporfin (VP, APExBIO, USA) was used to inhibit YAP. The cells were treated with VP dissolved in dimethyl sulfoxide (DMSO, Sigma, USA) or equal volume DMSO as the control for 12 h. The drug or control was diluted in the cell culture medium at the specified concentrations.
CCK-8 assay
The CCK-8 assay was used to assess the cytotoxicity of VP at different concentrations. The MDPC-23 cells were seeded in 96-well plates at a density of 4 × 103 cells/well. Once adhered, the cells were treated with various concentrations of VP or DMSO. At 0, 24, and 48 h post-treatment, the medium was replaced with 100 µL of culture medium containing 10 µL of CCK-8 solution (Beyotime, China) and incubated for 1.5 h. The absorbance at A450 was measured using a microplate spectrophotometer (BioTek, USA).
Western blotting
The cells were washed in cold PBS and lysed on ice using RIPA Lysis Buffer (ZHHC, China) supplemented with protease and phosphatase inhibitors (ZHHC, China). The lysates were centrifuged at 14,000 × g for 20 min at 4 °C, and the supernatant was collected for further analysis. Protein samples were denatured with 5 × loading buffer (Boster, China) for 5 min at 95 °C and subjected to 10% SDS-PAGE (Boster, China). The protein bands were transferred to polyvinylidene fluoride membranes (Millipore, USA), and blocked with Protein Free Rapid Blocking Buffer (Epizyme, China). Subsequently, the membranes were incubated with the following primary antibodies: rabbit anti-YAP antibody (14074S, 1:1000; Cell Signaling Technology, USA), rabbit anti-phospho-YAP1 antibody (bsm-52214R, 1:500; Bioss, China), mouse anti-DSPP antibody (sc-73632, 1:500; Santa Cruz, USA), or mouse anti-β-actin antibody (BM0627, 1:5000; Boster, China), followed by incubation with 1:5000 horseradish peroxidase-conjugated secondary antibodies (Boster, China) for 2 h at room temperature. Finally, the antibody-antigen complex was detected using enhanced chemiluminescence (Thermo, USA). β-actin was used as the control.
Statistical analysis
Data were statistically analyzed using GraphPad Prism 8.0. At least three independent experiments were performed, and representative images were displayed. The data were subjected to normality testing using the Shapiro–Wilk test. For multiple comparisons, two-way analysis of variance (ANOVA) or three-way ANOVA was performed to obtain p‐values. Post-hoc tests were performed when the ANOVA results indicated significant differences. Tukey’s multiple comparisons test was employed for post-hoc analyses in two-way ANOVA, whereas Sidak’s multiple comparisons test was utilized for post-hoc analyses in three-way ANOVA. Differences between the two groups were determined using the unpaired two-tailed Student’s t-test. A p-value <0.05 was considered significant. Data are presented as the mean ± SD.
Results
MHS did not alter the morphology of MDPC-23 cells
To investigate the influence of MHS on the viability and differentiation of MDPC-23 cells, we used a previously described [Citation29] in vitro heat stress loading model to simulate the changing thermal microenvironment of dental pulp cells (). The temperature rose from 37 °C to 41 °C in ∼900 s (). No significant alterations were observed in the cell morphology or area under MHS ().
MHS maintained the viability of MDPC-23 cells
To explore the influence of MHS on cell viability, we performed an MTT assay. The cell viability was higher in the dynamic-MHS group compared to that in the control group (). In contrast, the mixed-MHS group displayed no remarkable difference in viability compared to the control group (). This suggests that the viability of MDPC-23 cells increased remarkably under dynamic MHS but returned to normal levels under subsequent constant MHS.
MHS promoted the gene expression of HSP70 and HSP90α
As the heating time increased, the thermal microenvironment of MDPC-23 cells changed from a dynamic to a constant state. The gene expression of HSP70 increased in both the dynamic-MHS and mixed-MHS groups compared to the control group (). Moreover, the dynamic-MHS group exhibited a remarkably higher HSP70 expression than the mixed-MHS group (). The gene expression of HSP90α, the stress-inducible isoform of HSP90 encoded by HSP90AA1 [Citation17,Citation30], increased in both the dynamic-MHS and mixed-MHS groups, and the expression was not considerably different between the two groups (). In summary, MHS promotes the transcription of HSP70 and HSP90α, with dynamic MHS having a more pronounced effect on HSP70 transcription compared to mixed MHS.
MHS increased the nucleoplasmic ratio of YAP
YAP activity was assessed using immunofluorescence staining and immunoblotting. Immunostaining showed that the YAP protein initially diffused throughout the cytoplasm and nucleus of normally cultured MDPC-23 cells and translocated to the nucleus in both the dynamic-MHS and mixed-MHS groups (). The nucleoplasmic ratio of YAP was increased in both groups compared to that in the control (). Immunoblotting confirmed that both dynamic and mixed MHS promoted YAP dephosphorylation (). Therefore, MHS enhances YAP activity.
Verteporfin inhibited the expression and nucleoplasmic ratio of YAP
Cell viability after verteporfin (VP) treatment was assessed using the CCK8 assay, while immunofluorescence and immunoblotting were used to evaluate YAP expression and the nucleo-cytoplasmic ratio. At 24 h, MDPC-23 cells treated with increasing concentrations of VP (1, 3, 5, 7 μM) showed no obvious changes in viability. However, at 48 h, the viability of MDPC-23 cells decreased remarkably in the 3, 5, and 7 μM VP treatment groups (Figure S2). The immunofluorescence intensity and nucleoplasmic ratio of the YAP protein remarkably decreased in the 3 μM VP group under normal conditions and MHS (). Western blotting revealed that YAP was decreased in the 3 μM VP group under normal conditions and MHS (). Therefore, VP effectively inhibited the expression and nucleoplasmic ratio of YAP.
Figure 2. YAP is crucial for the maintenance of cell viability under mild heat stress (MHS). (a) Representative images of immunofluorescence-stained YAP in MDPC-23 cells pretreated with verteporfin under dynamic MHS. Scale bar, 10 μm. (b) Representative images of immunostained YAP in MDPC-23 cells pretreated with verteporfin under mixed MHS. Scale bar, 10 μm. (c) Quantification of the immunofluorescence staining and nucleo-cytoplasmic ratio in (a). (d) Quantification of the immunofluorescence staining and nucleo-cytoplasmic ratio in (b). (e) The relative protein levels of YAP in MDPC-23 cells pretreated with verteporfin under dynamic MHS. (f) The relative protein levels of YAP in MDPC-23 cells pretreated with verteporfin under mixed MHS. (g) The viability of MDPC-23 cells pretreated with verteporfin under MHS. (h) The gene expression of HSP90α in MDPC-23 cells treated with verteporfin under MHS. (Mean ± SD. ns, no statistically significant difference; *p < 0.05; **p < 0.01; ***p < 0.001; ****p < 0.0001).
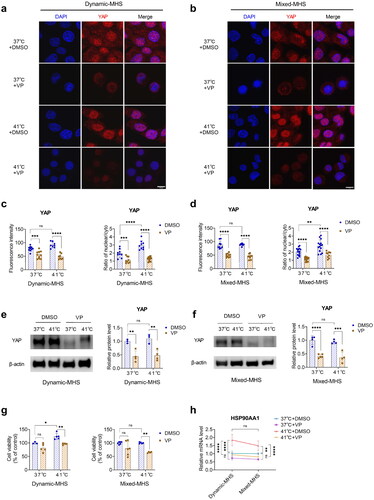
YAP inhibition diminished the viability of MDPC-23 cells
To investigate the influence of YAP on cell viability, the cell viability of MDPC-23 cells pretreated with 3 μM VP under MHS was determined by MTT. We found a remarkable decrease in cell viability in the 3 μM VP group under both dynamic and mixed MHS (), highlighting the crucial role of YAP in maintaining cell viability under MHS.
YAP inhibition diminished the transcription of HSP90α
We explored the influence of YAP on the transcription of heat shock proteins, in cells pretreated with VP under MHS using qPCR. The gene expression of HSP70 increased remarkably in the VP group under dynamic MHS, non-remarkably in the VP group under mixed MHS, and remained unchanged in the VP group under normal conditions (Figure S3). In contrast, the gene expression of HSP90α decreased in the VP group under both dynamic and mixed MHS, whereas it remained unchanged in the VP group under normal conditions (). Therefore, the HSP90α mRNA levels decreased, while HSP70 mRNA levels increased in YAP-inhibited cells under MHS.
MHS promoted the expression of DSPP, DMP1, and HSP25
To explore the influence of MHS on odontoblast differentiation, we conducted immunofluorescence and immunoblotting to assess DSPP protein expression and qPCR to measure DMP1 and HSP25 gene expression. DSPP was mainly localized in the cytoplasm of MDPC-23 cells and showed remarkably increased expression under MHS (). The mRNA expression levels of DMP1 and HSP25 were also remarkably increased under MHS (). Notably, the mRNA level of HSP25 was higher under dynamic MHS than under mixed MHS (). Therefore, MHS enhanced the levels of HSP25 mRNA, DMP1 mRNA, and DSPP protein.
Figure 3. Mild heat stress (MHS) promotes odontoblast differentiation of MDPC-23 cells. (a) Representative images of immunofluorescence-stained DSPP under dynamic MHS. Scale bar, 50 μm. (b) Representative images of immunofluorescence-stained DSPP under mixed MHS. Scale bar, 50 μm. (c) Quantification of the immunofluorescence staining in (a). (d) The relative protein levels of DSPP in MDPC-23 cells under dynamic MHS. (e) Quantification of the immunofluorescence staining in (b). (f) The relative protein levels of DSPP in MDPC-23 cells under mixed MHS. (g) The gene expression of DMP1 in MDPC-23 cells under MHS. (h) The gene expression of HSP25 in MDPC-23 cells under MHS. (Mean ± SD. ns, no statistically significant difference; *p < 0.05; ****p < 0.0001).
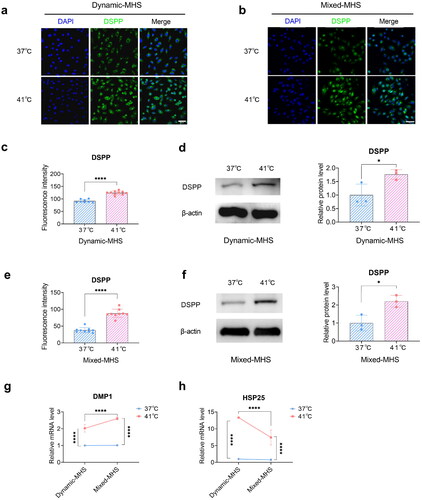
YAP inhibition diminished the expression of DSPP and DMP1
We applied MHS to cells pretreated with VP and detected the level of DSPP protein using immunofluorescence and immunoblotting and the level of DMP1 mRNA using qPCR. The levels of DSPP protein () and DMP1 mRNA () were decreased in YAP-inhibited cells under normal conditions and MHS.
Figure 4. YAP is crucial for the odontoblast differentiation of MDPC-23 cells under mild heat stress (MHS). (a) Representative images of immunofluorescence-stained DSPP in MDPC-23 cells pretreated with verteporfin under dynamic MHS. Scale bar, 30 μm. (b) Representative images of immunofluorescence-stained DSPP in MDPC-23 cells pretreated with verteporfin under mixed MHS. Scale bar, 30 μm. (c) Quantification of the fluorescence intensities in (a). (d) The relative protein levels of DSPP in MDPC-23 cells pretreated with verteporfin under dynamic MHS. (e) Quantification of the immunofluorescence staining in (b). (f) The relative protein levels of DSPP in MDPC-23 cells pretreated with verteporfin under mixed MHS. (g) The gene expression of DMP1 in MDPC-23 cells pretreated with verteporfin under MHS. (h) The gene expression of HSP25 in MDPC-23 cells pretreated with verteporfin under MHS. (Mean ± SD. ns, no statistically significant difference; *p < 0.05; **p < 0.01; ***p < 0.001; ****p < 0.0001).
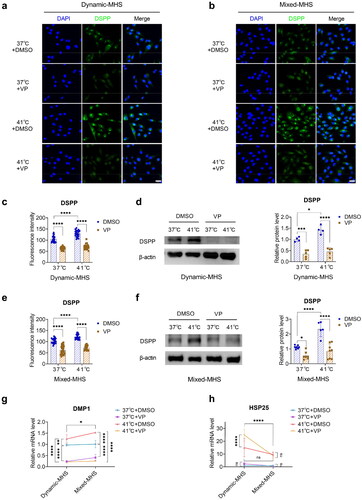
YAP inhibition increased the gene expression of HSP25 under dynamic MHS
MDPC-23 cells displayed a remarkable increase in HSP25 mRNA levels in the VP group under dynamic MHS, whereas no remarkable changes were observed in the VP group under normal conditions or mixed MHS ().
Discussion
In this study, we investigated the effects of MHS on the viability and differentiation of MDPC-23 cells, focusing on the role of YAP. We found that MHS maintains cell viability and promotes odontoblast differentiation through YAP. YAP inhibition led to decreased cell viability and odontoblast differentiation. Therefore, enhancing YAP activity may serve as a potential strategy to maintain cell viability and promote odontoblast differentiation under heat stress. Therefore, appropriate thermotherapy can be used to treat dentin hypersensitivity by inducing reparative dentinogenesis ().
Figure 5. Proposed model for the regulation of cell viability and odontoblast differentiation in MDPC-23 cells via YAP under mild heat stress (MHS). We propose that MHS enhances YAP activation, thereby maintaining cell viability and promoting odontoblast differentiation in MDPC-23 cells. Heat shock proteins collaborate to maintain cellular homeostasis, and interact with YAP.
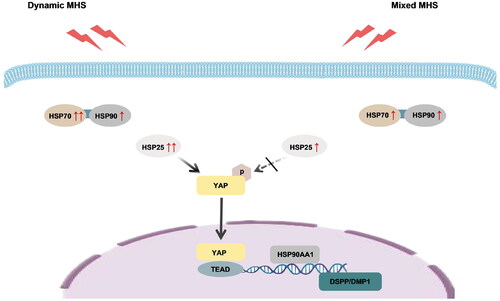
Previous studies have primarily examined cellular responses to constant MHS [Citation8,Citation17,Citation31], while the effects of dynamic and mixed MHS have not been investigated. In this study, an in vitro heat stress loading model was used to simulate the thermal microenvironment of odontoblast-like cells. The dynamic-MHS group represented a dynamic thermal microenvironment during the heat-up phase, whereas the mixed-MHS group represented a complex thermal microenvironment during the heat-up and constant-temperature phases. We found that cell viability increased under dynamic MHS but returned to normal under mixed MHS. Cell viability under MHS may be maintained through the cooperation of heat shock proteins (HSP70 and HSP90). HSP70 initially interacts with the unfolded proteins, whereas HSP90 participates in the subsequent folding process [Citation23]. The HSP70-HSP90 chaperone cascade plays an important role in protein synthesis and the correction of misfolded proteins under stress [Citation23,Citation32]. Although the gene expression of HSP70 increased under both dynamic and mixed MHS conditions, it was remarkably higher under dynamic MHS, indicating its crucial role in the early response to heat stress. HSP70 is also associated with odontoblast viability [Citation20,Citation33]. The enhanced cell viability observed under dynamic MHS may be attributed to the increased expression of HSP70. The mRNA levels of HSP90α, the stress-inducible isoform of HSP90 encoded by HSP90AA1 [Citation17,Citation30], increased under dynamic MHS and remained stable under constant MHS. This maintenance of HSP90α levels contributes to cell homeostasis and thermotolerance of MDPC-23 cells. In conclusion, our findings suggest that dynamic MHS leads to increased cell viability, possibly via enhanced expression of HSP70. Moreover, the transcription of HSP90 is crucial for cellular homeostasis and thermotolerance under heat stress.
The results of this study confirm the essential role of YAP in maintaining cell viability by regulating the transcription of HSP90α. Consistent with previous findings [Citation34–36], the gene expression of HSP90α was elevated under MHS to maintain cell homeostasis and enhance thermotolerance. Inhibition of YAP led to a decrease in HSP90α gene expression under MHS, resulting in diminished cell viability. This aligns with previous observations of the repression of HSP90α transcription in YAP/TAZ deleted or knockdown cells [Citation17]. Inhibition of HSP90α degrades client proteins crucial for protein homeostasis and cell survival [Citation37,Citation38]. Additionally, YAP inhibition also led to an increase in the mRNA levels of HSP70 under dynamic MHS. HSP90 functions downstream of HSP70 and is crucial in protein folding [Citation23]; a compensatory increase in HSP70 expression occurs in response to HSP90 downregulation [Citation39–42]. While YAP has little effect on the transcription of HSP70 under heat stress [Citation17], the compensatory transcription of HSP70 in the VP group may be attributed to the decrease in HSP90α. Consistent with its significant role in the early phase of heat stress responses, the compensatory transcription of HSP70 was more significant under dynamic MHS. However, this compensatory transcription of HSP70, in line with the results of a previous study [Citation40], failed to maintain cellular homeostasis and viability. Overall, our findings highlight the importance of YAP in maintaining cell viability by regulating the transcription of HSP90α. Further, our findings suggest the cooperation of HSP70 and HSP90 in maintaining cellular homeostasis, and the influence of dynamic and mixed MHS on cell viability.
Periodic MHS promotes osteoblastic differentiation of ectomesenchymal stem cells by activating YAP [Citation43]. In this study, we observed that MHS accelerated odontoblast differentiation of MDPC-23 cells. YAP played a significant role in this process by promoting the expression of DSPP and DMP1. DSPP is an important marker of odontogenesis, and its deficiency can lead to dentin diseases [Citation44]. DMP1 is crucial for dentin formation and acts as a regulator of odontoblast differentiation in dental pulp cells after tooth injury [Citation20]. The upregulated expression of DSPP and DMP1 under MHS may accelerate the differentiation of odontoblast-like cells and promote dentin formation. HSP25 acts as a switch between cell proliferation and differentiation in odontoblasts [Citation20]. The transcription of HSP25 precedes DSPP during the early differentiation of odontoblast-like cells under heat stress or during pulp healing [Citation8,Citation45]. In this study, we observed that the gene expression of HSP25 increased more remarkably under dynamic MHS than under constant MHS, indicating that the odontoblast differentiation of MDPC-23 cells was initiated under dynamic MHS. Previous studies reported that HSP25 regulates YAP activation [Citation25,Citation26]. The overexpression of HSP27, a human homolog of HSP25, significantly increases YAP dephosphorylation in various cancer cells [Citation26]. The inhibition of HSP27 suppresses YAP activation in oral carcinoma cells [Citation25]. Therefore, HSP25 may accelerate the odontoblast differentiation of MDPC-23 cells by activating YAP under dynamic MHS. This explains why YAP inhibition led to the increased transcription of HSP25 and decreased expression of DSPP and DMP1 under dynamic MHS. However, the transcription of HSP25 decreased under constant MHS, suggesting that HSP25 may be less important under constant MHS. YAP inhibition under constant MHS had little influence on the transcription of HSP25 but still decreased the expression of DSPP and DMP1, indicating that YAP activity may be regulated by other factors under constant MHS. Further studies are required to verify the interaction between HSP25 and YAP.
In summary, we demonstrated that YAP maintained cell viability and promoted odontoblast differentiation of MDPC-23 cells under MHS. The survival and differentiation of odontoblast-like cells under stress are essential for inducing pulp regeneration and ensuring normal dentin formation [Citation6]. Given the unsatisfactory success of the existing approaches in clinical therapy for treating DH [Citation7] the findings of our study, suggest the potential for the promotion of odontoblast differentiation through MHS and regulation of YAP activity, may be leveraged for developing new treatment approaches. We are now beginning to understand the relationship between YAP and HSP; however, the influence of HSP25 on YAP remains largely unknown. In conclusion, optimal thermotherapy may serve as an alternative treatment for DH, and enhancing YAP activity may promote the differentiation of odontoblast-like cells and accelerate the formation of reparative dentin.
Author contributions
Peiqi Liu and Zhen Li contributed to the conceptualization, methodology, validation, formal analysis, and data curation, drafted and critically revised the manuscript. Hui Zhang, Yijie Wang, Yuxin Liao, Yi Guo, Chenxu Wang, and Yuanwu Zou contributed to validation, formal analysis, and critically revised the manuscript. Rui Zou and Lin Niu contributed to conceptualization, methodology, resources, supervision, project administration and funding acquisition, drafted and critically revised the manuscript. All authors gave final approval and agreed to be accountable for all aspects of the work.
Supplemental Material
Download ()Acknowledgments
The authors wish to thank Prof. Franklin R. Tay of Augusta University for providing odontoblast-like MDPC-23 cells.
Disclosure statement
No potential conflict of interest was reported by the author(s).
Data availability statement
The authors confirm that the data supporting the findings of this study are available within the article and its Supplementary Materials.
Additional information
Funding
References
- Mahdian M, Behboodi S, Ogata Y, et al. Laser therapy for dentinal hypersensitivity. Cochrane Database Syst Rev. 2021;7(7):CD009434. doi: 10.1002/14651858.CD009434.pub2.
- Holland GR, Narhi MN, Addy M, et al. Guidelines for the design and conduct of clinical trials on dentine hypersensitivity. J Clin Periodontol. 1997;24(11):808–813. doi: 10.1111/j.1600-051x.1997.tb01194.x.
- Liu XX, Tenenbaum HC, Wilder RS, et al. Pathogenesis, diagnosis and management of dentin hypersensitivity: an evidence-based overview for dental practitioners. BMC Oral Health. 2020;20(1):220. doi: 10.1186/s12903-020-01199-z.
- Choung HW, Lee DS, Lee JH, et al. Tertiary dentin formation after indirect pulp capping using protein CPNE7. J Dent Res. 2016;95(8):906–912. doi: 10.1177/0022034516639919.
- Smith AJ, Duncan HF, Diogenes A, et al. Exploiting the bioactive properties of the dentin-pulp complex in regenerative endodontics. J Endod. 2016;42(1):47–56. doi: 10.1016/j.joen.2015.10.019.
- Kawashima N, Okiji T. Odontoblasts: specialized hard-tissue-forming cells in the dentin-pulp complex. Congenit Anom. 2016;56(4):144–153. doi: 10.1111/cga.12169.
- Morotomi T, Washio A, Kitamura C. Current and future options for dental pulp therapy. Jpn Dent Sci Rev. 2019;55(1):5–11. doi: 10.1016/j.jdsr.2018.09.001.
- Morotomi T, Kitamura C, Okinaga T, et al. Continuous fever-range heat stress induces thermotolerance in odontoblast-lineage cells. Arch Oral Biol. 2014;59(7):741–748. doi: 10.1016/j.archoralbio.2014.03.014.
- Niu L, Dong SJ, Kong TT, et al. Heat transfer behavior across the dentino-enamel junction in the human tooth. PLOS One. 2016;11(9):e0158233. doi: 10.1371/journal.pone.0158233.
- Lynch CD, Roberts JL, Al-Shehri A, et al. An ex-vivo model to determine dental pulp responses to heat and light-curing of dental restorative materials. J Dent. 2018;79:11–18. doi: 10.1016/j.jdent.2018.08.014.
- Andreeva NV, Zatsepina OG, Garbuz DG, et al. Recombinant HSP70 and mild heat shock stimulate growth of aged mesenchymal stem cells. Cell Stress Chaperones. 2016;21(4):727–733. doi: 10.1007/s12192-016-0691-7.
- Li L, Zhang X, Zhou J, et al. Non-invasive thermal therapy for tissue engineering and regenerative medicine. Small. 2022;18(36):e2107705. doi: 10.1002/smll.202107705.
- Rezai Rad M, Wise GE, Brooks H, et al. Activation of proliferation and differentiation of dental follicle stem cells (DFSCs) by heat stress. Cell Prolif. 2013;46(1):58–66. doi: 10.1111/cpr.12004.
- Schupper AJ, Chanenchuk T, Racanelli A, et al. Laser hyperthermia: past, present, and future. Neuro Oncol. 2022;24(Suppl 6):S42–S51. doi: 10.1093/neuonc/noac208.
- Yang Z, Gao D, Zhao J, et al. Thermal immuno-nanomedicine in cancer. Nat Rev Clin Oncol. 2023;20(2):116–134. doi: 10.1038/s41571-022-00717-y.
- Jiang X, Maruyama J, Iwasa H, et al. Heat shock induces the nuclear accumulation of YAP1 via SRC. Exp Cell Res. 2021;399(1):112439. doi: 10.1016/j.yexcr.2020.112439.
- Luo M, Meng Z, Moroishi T, et al. Heat stress activates YAP/TAZ to induce the heat shock transcriptome. Nat Cell Biol. 2020;22(12):1447–1459. doi: 10.1038/s41556-020-00602-9.
- Yang B, Sun H, Song F, et al. Yes-associated protein 1 promotes the differentiation and mineralization of cementoblast. J Cell Physiol. 2018;233(3):2213–2224. doi: 10.1002/jcp.26089.
- Fu J, Zhang X, Zheng H, et al. A WWP2-PTEN-KLF5 signaling axis regulates odontoblast differentiation and dentinogenesis in mice. J Biol Chem. 2022;298(8):102220. doi: 10.1016/j.jbc.2022.102220.
- Guo S, Yang H, Liu J, et al. Heat shock proteins in tooth development and injury repair. Int J Mol Sci. 2023;24(8):7455. doi: 10.3390/ijms24087455.
- Tukaj S, Sitko K. Heat shock protein 90 (Hsp90) and Hsp70 as potential therapeutic targets in autoimmune skin diseases. Biomolecules. 2022;12(8):1153. doi: 10.3390/biom12081153.
- Morotomi T, Kitamura C, Toyono T, et al. Effects of heat stress and starvation on clonal odontoblast-like cells. J Endod. 2011;37(7):955–961. doi: 10.1016/j.joen.2011.03.037.
- Morán Luengo T, Mayer MP, Rüdiger SGD. The Hsp70-Hsp90 chaperone cascade in protein folding. Trends Cell Biol. 2019;29(2):164–177. doi: 10.1016/j.tcb.2018.10.004.
- Nakasone N, Yoshie H, Ohshima H. An immunohistochemical study of the expression of heat-shock protein-25 and cell proliferation in the dental pulp and enamel organ during odontogenesis in rat molars. Arch Oral Biol. 2006;51(5):378–386. doi: 10.1016/j.archoralbio.2005.09.007.
- Ahmad US, Parkinson EK, Wan H. Desmoglein-3 induces YAP phosphorylation and inactivation during collective migration of oral carcinoma cells. Mol Oncol. 2022;16(8):1625–1649. doi: 10.1002/1878-0261.13177.
- Vahid S, Thaper D, Gibson KF, et al. Molecular chaperone Hsp27 regulates the Hippo tumor suppressor pathway in cancer. Sci Rep. 2016;6(1):31842. doi: 10.1038/srep31842.
- Adachi M, Liu Y, Fujii K, et al. Oxidative stress impairs the heat stress response and delays unfolded protein recovery. PLOS One. 2009;4(11):e7719. doi: 10.1371/journal.pone.0007719.
- Lin C, Zhang Y, Zhang K, et al. Fever promotes T lymphocyte trafficking via a thermal sensory pathway involving heat shock protein 90 and alpha4 integrins. Immunity. 2019;50(1):137–151.e6. doi: 10.1016/j.immuni.2018.11.013.
- Qiu J, Li Z, An K, et al. Thermo-chemical resistance to combination therapy of glioma depends on cellular energy level. ACS Appl Mater Interfaces. 2023;15(33):39053–39063. doi: 10.1021/acsami.3c05683.
- Zuehlke AD, Beebe K, Neckers L, et al. Regulation and function of the human HSP90AA1 gene. Gene. 2015;570(1):8–16. doi: 10.1016/j.gene.2015.06.018.
- Amano T, Muramatsu T, Amemiya K, et al. Responses of rat pulp cells to heat stress in vitro. J Dent Res. 2006;85(5):432–435. doi: 10.1177/154405910608500507.
- Unel NM, Baloglu MC, Altunoglu YC. Comprehensive investigation of cucumber heat shock proteins under abiotic stress conditions: a multi-omics survey. J Biotechnol. 2023;374:49–69. doi: 10.1016/j.jbiotec.2023.07.010.
- Kero D, Kalibovic Govorko D, Medvedec Mikic I, et al. Analysis of expression patterns of IGF-1, caspase-3 and HSP-70 in developing human tooth germs. Arch Oral Biol. 2015;60(10):1533–1544. doi: 10.1016/j.archoralbio.2015.07.004.
- Saadeldin IM, Swelum AA, Zakri AM, et al. Effects of acute hyperthermia on the thermotolerance of cow and sheep skin-derived fibroblasts. Animals. 2020;10(4):545. doi: 10.3390/ani10040545.
- Uehara Y, Temma K, Kobayashi Y, et al. Reduction of thermotolerance by heat shock protein 90 inhibitors in murine erythroleukemia cells. Biol Pharm Bull. 2018;41(9):1393–1400. doi: 10.1248/bpb.b18-00190.
- Vriend LEM, van den Tempel N, Oei AL, et al. Boosting the effects of hyperthermia-based anticancer treatments by HSP90 inhibition. Oncotarget. 2017;8(57):97490–97503. doi: 10.18632/oncotarget.22142.
- Garcia-Carbonero R, Carnero A, Paz-Ares L. Inhibition of HSP90 molecular chaperones: moving into the clinic. Lancet Oncol. 2013;14(9):e358–e369. doi: 10.1016/S1470-2045(13)70169-4.
- Zhou C, Zhang C, Zhu H, et al. Allosteric regulation of Hsp90alpha’s activity by small molecules targeting the middle domain of the chaperone. iScience. 2020;23(2):100857. doi: 10.1016/j.isci.2020.100857.
- Daturpalli S, Waudby CA, Meehan S, et al. Hsp90 inhibits alpha-synuclein aggregation by interacting with soluble oligomers. J Mol Biol. 2013;425(22):4614–4628. doi: 10.1016/j.jmb.2013.08.006.
- Duncan RF. Inhibition of Hsp90 function delays and impairs recovery from heat shock. FEBS J. 2005;272(20):5244–5256. doi: 10.1111/j.1742-4658.2005.04921.x.
- Lackie RE, Maciejewski A, Ostapchenko VG, et al. The Hsp70/Hsp90 chaperone machinery in neurodegenerative diseases. Front Neurosci. 2017;11:254. doi: 10.3389/fnins.2017.00254.
- Sittler A, Lurz R, Lueder G, et al. Geldanamycin activates a heat shock response and inhibits huntingtin aggregation in a cell culture model of Huntington’s disease. Hum Mol Genet. 2001;10(12):1307–1315. doi: 10.1093/hmg/10.12.1307.
- Shi W, Wang Z, Bian L, et al. Periodic heat stress licenses EMSC differentiation into osteoblasts via YAP signaling pathway activation. Stem Cells Int. 2022;2022:3715471. doi: 10.1155/2022/3715471.
- Chen S, Xie H, Zhao S, et al. The genes involved in dentinogenesis. Organogenesis. 2022;18(1):1–19. doi: 10.1080/15476278.2021.2022373.
- Nakatomi M, Ida-Yonemochi H, Ohshima H. Lymphoid enhancer-binding factor 1 expression precedes dentin sialophosphoprotein expression during rat odontoblast differentiation and regeneration. J Endod. 2013;39(5):612–618. doi: 10.1016/j.joen.2012.12.016.