ABSTRACT
The development of the cyanobiphenyls for display applications was one of the most important technological advances of the 20th century. Here, we present a personal account of how advances in biphenyl science (synthesis and application) influenced the development of discotic liquid crystals. We focus on those based on triphenylene, the most widely studied discotic core. The search for nematic discotics proved to be challenging with relatively few early examples being reported. In examples of symmetrically hexasubstituted triphenylenes, a ring of peripheral aryl ester or alkynylaryl substituents provides effective steric blocking to columnar assembly – the face-to-face molecular arrangement that is observed in the majority of triphenylene discotics. The synthetic challenges associated with early triphenylene synthesis have now been largely overcome. Efficient oxidative trimerisation of dialkoxybenzenes gave direct access to materials with high purity and at scale. The intermediates can be manipulated through deprotection and elaboration, a pathway that allowed synthesis of lyotropic (Nc) systems among others. The combination of modern cross-coupling chemistry with oxidative processes has proved versatile, leading to synthesis of unsymmetrical monomeric and dimeric systems. Dimeric systems linked by rigid bridges have been identified as a separate class of materials that show strong tendency for nematic mesophase formation.
Graphical abstract
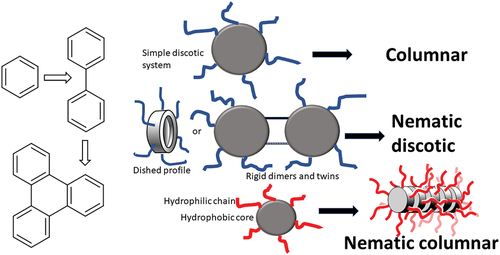
Introduction
The flat-screen display industry was founded upon the ease with which thermotropic nematic phases can be aligned using surface effects and the ease with which that alignment can be switched using electromagnetic fields. In the development of these applications the invention of the cyanobiphenyls was the game-changing step. Cyanobiphenyls became the basis of most displays as well as the main ‘workhorse’ used by liquid crystal scientists world-wide. It was this success that led to the belief that, for essentially all liquid crystal devices, electromagnetically switchable phases would be the key to success, and the focus naturally lay with the low viscosity nematics.
As a result, when discotic liquid crystals were discovered, there was immediate interest in finding mesogens that could be switched, and particularly on materials that would give discotic nematic (ND) phases. Partly, these hopes proved false (discotic nematics proved difficult to obtain, difficult to align and difficult to switch!), but it was the ND phases which led to the first commercially important application. The first generation of discotic liquid crystals were all columnar [Citation1] and subsequent development of the field has been dominated by studies of the columnar phase [Citation2,Citation3]. Indeed, some early studies also investigated potential switching of ferroelectric/chiral columnar systems [Citation4]. While biphenyls can be considered the workhorse of calamitic liquid crystal development, the same can be said for triphenylenes within the development of discotic liquid crystals [Citation5]. Unlike calamitic systems, where nematic systems are not uncommon and where it is not unusual to find nematic/smectic phase transitions, ND phases are relatively rare and ND/columnar phase transitions are particularly scarce [Citation6]. This is because the molecular features which give rise to columnar and to ND phases are different ().
Figure 1. (Colour online) (a) mesogens giving rise to the ND phase (b) schematic representation of a discogen core with a ‘dished’ surface. (cross-section right) (c) schematic of the columnar stacking of flat aryl cores. (d) schematic of the columnar stacking of ‘dished’ aryl cores. (e) schematic of the ND phase of flat aryl cores. (f) schematic of the ND phase of ‘dished’ aryl cores. In (c)-(f) the varying degrees of accessible conformational space (c>f>e) for the (red) side-chains is indicated by the pale red shading (see text).
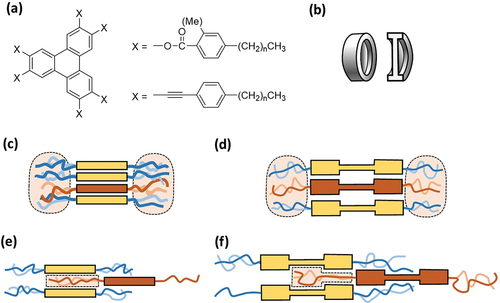
The tendency of discotic liquid crystals to give columnar phases is often vaguely attributed to ‘pi-stacking interactions’ with the unspoken implication that ‘something’ always causes aromatic rings to stack on top of each other giving columns. However, this is not the case. In the absence of significant dipole/dipole interactions, the stacking of aromatic rings is dictated by the competing effects of the van der Waals interaction (favouring co-planar/columnar stacking) and quadrupole/quadrupole interaction (favouring an orthogonal arrangement) [Citation7]. Hence, for benzene and many of its derivatives, the quadrupole term dominates leading to a preferred orthogonal arrangement of nearest neighbour molecules; often a ‘herringbone’ packing in the crystalline state. Only for polynuclear aromatics, where there is increased delocalisation, easier polarisation, and stronger van der Waals interactions, is columnar stacking favoured. For discotic mesogens, the significant ‘extra factor’ which favours columnar stacking is chain/core micro-phase separation. The large enthalpy associated with the Cr/Col transition relates to the melting of the side-chains [Citation8]; it relates to the entropic term associated with the transformation from one to many conformational states. This is maximised when the conformational space available to the side-chains is also maximised. This is the case when discogens are stacked one-on-top of each other (as in the columnar phase, ) but not when the side-chains are (partly) sandwiched between rigid aromatic cores (as in the ND phase, ). The first triphenylene ND systems to be discovered were those where the core was surrounded by O2CC6H4R [Citation9–12] or C≡CC6H4R [Citation13,Citation14] substituents (). These substituents create a core that has a ‘dished surface; one that is thicker at its rim than it is in its centre (). In the case of the O2CC6H4R systems, this arises because, in the most stable conformation, the benzene ring is twisted out of the plane of the triphenylene. In the C≡CC6H4R systems, the most stable conformation has all the aryl rings coplanar, but the barrier to rotation is so low [Citation15] that there is a significant population of non-coplanar states. This destabilises the columnar phase. Packing of ‘dished’ rings is generally difficult and packing in columns (in particular) creates free space [Citation16] (Compare . However, in the nematic phase, it provides additional conformational space for the side-chains (compare ).
It was the ND phases of the ester derivatives that were to lead to the first significant (and, so far, only significant) commercial application of discotics. Although they have switching times that are far too slow for them to be useful as display materials, their optical properties provided the ideal solution to the problem of improving the angle of view of liquid crystal display [Citation17,Citation18]. In the early years of liquid crystal displays this was a persistent problem. A clear image with true colours was only seen when the observer was positioned directly in front of the screen. This is a result of the birefringence of the oriented liquid crystal film. In the ‘on’ state of a twisted nematic display, although the director is perpendicular to the electrodes in the centre of the cell, it remains pinned in a planar orientation next to the surface. This ‘hybrid’ orientation of the director gives a birefringence profile that can be cancelled by a matching discotic film with the opposite birefringence profile. Fortunately, thin films of ND discotics provide exactly that with planar alignment of the director at the solid interface and a perpendicular or tilted alignment at the air interface (). Furthermore, in the case of the ‘reactive mesogen esters’ (), polymerisation locks this birefringence profile into a stable film that is transparent and cheap to make; a polymer film that solves the ‘angle of view’ problem.
Figure 2. (Colour online) (a) example of a mesogen used in the manufacture of optical compensating film. (b) schematic of a nematic film with a hybrid orientation of the director.
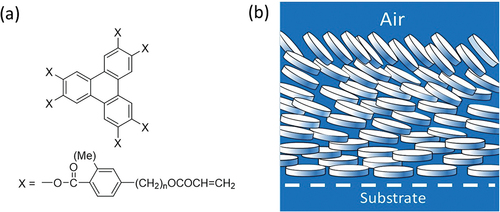
The success of the nematic phases of the cyanobiphenyls also stimulated interest in the stabilisation of nematic phases of lyotropic liquid crystals and, once again, discotic mesogens provided an answer. It was shown as far back as 1967 [Citation19] that solutions of orientationally ordered disc-shaped (ND) or rod-shaped (NC) micelles [Citation20–22] could give rise to nematic behaviour, but the first lyotropic nematic phases found were only stable over very restricted ranges of temperature and concentration [Citation23,Citation24]. In part, this is a result of the fact that (because hydrophobic alkyl chains are so flexible) a conventional amphiphile can give rise to rod-shaped, spherical or disc-shaped aggregates (). However, amphiphiles with a rigid hydrophobic part can only form one type of aggregate. For example, a discoid amphiphile consisting of a rigid hydrophobic core which is surrounded by hydrophilic groups can only give rod-shaped aggregates and NC nematic phases. The first example of such a discoid amphiphile was 2,3,6,7,10,11-hexa-(1′,4′,7′-trioxaoctyI)-triphenylene () normally known by the abbreviation TP6EO2M [Citation25]. In water, this amphiphile forms an NC phase over a wide range of concentrations and temperatures [Citation26]. Although designating these rod-like aggregates as ‘micelles’ is perhaps useful in making the conceptual link to the NC phases formed by conventional lyotropic systems, perhaps the term ‘aggregate’ is preferable. The aggregation of TP6EO2M is pseudo-isodesmic, and there is no critical micelle concentration [Citation26–30]. Since the discovery of TP6EO2M, a range of similar systems with wide nematic ranges have been reported. These include mesogens in which perylenebisimine [Citation31], dibenzophenazine [Citation32], phthalocyanine [Citation33] and tricycloquinazoline [Citation34] hydrophobic cores are surrounded by an annulus of hydrophilic EOn chains. Like thermotropic nematic systems, the lyotropic NC systems can be oriented in strong magnetic fields, but unlike the thermotropic nematics, they prove frustratingly difficult to orient using surface effects [Citation35]. What was not realised at the time TP6EO2M was developed was that it is a non-ionic analogue of a ‘chromonic’ liquid crystal and chromonic liquid crystals systems [Citation36] had been known (if not properly understood) for many years [Citation37,Citation38].
Figure 3. (Colour online) (a) the molecular formula of TP6EO2M (b) the contrast between the types of aggregate that can be formed in water by an amphiphile with a flexible hydrophobic alkyl chain and one (like TP6EO2M) that has a rigid hydrophobic aryl core. For the first three aggregates a few molecules have been removed from part of the surface to reveal the hydrophobic core.
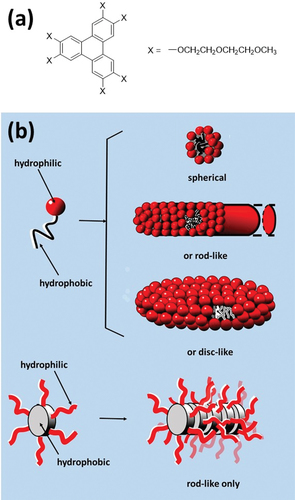
The link between biphenyls and triphenylenes extends beyond their respective status as benchmark cores for calamitic and discotic liquid crystals because biphenyls play an important role in the synthesis and synthetic development of triphenylenes. For the most part, the molecules discussed so far are based on symmetrically hexa-substituted triphenylenes. Structures of this type became readily available when efficient cyclotrimerization of dialkoxybenzenes was achieved [Citation39]. Oxidative trimerisation of dimethoxybenzene (veratrole) followed by exhaustive demethylation provides the starting material for synthesis of hexa-esters, among many other possibilities ().
Scheme 1. Straightforward synthesis of hexaalkoxytriphenylenes (HAT), the presumed reaction sequence, and products from full and partial (single) hydrolysis.
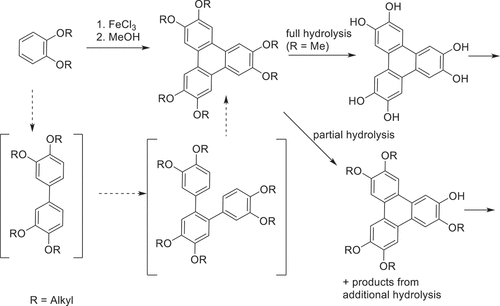
Unsymmetrically substituted triphenylenes remained difficult to access for some time. Some success has been achieved via reaction of a mixture of substituted benzenes [Citation39] or partial dealkylation/hydrolysis strategies [Citation40–42], followed by separation of the various reaction products. However, the first breakthrough in this area stemmed from consideration of the mechanistic sequence that converts (substituted) benzenes into triphenylenes. As depicted in , the reaction is presumed to follow the sequence benzene-biphenyl-terphenyl-triphenylene, intermediates with frameworks that had become highly familiar within the LC field. We reasoned that a pre-synthesised suitable biphenyl intermediate could subsequently lead to controlled preparation of a single unsymmetrical triphenylene upon reaction with a complementary benzene partner, so long as the reaction conditions favoured triphenylene formation over the known oxidative dimerisation of biphenyl intermediates [Citation43]. The protocol proved to be successful and convenient [Citation44]. Symmetrical and unsymmetrical tetraalkoxybiphenyls, prepared by traditional Ullman-type coupling of halobenzenes, were reacted with dialkoxybenzenes again using FeCl3 as oxidant (). Combinations of long-chain alkoxy (hexyl) and methoxy substituents were chosen to aid separation/isolation, but also to allow further manipulation via selective deprotection of the methyl ethers. The resulting dihydroxytriphenylene derivatives were originally converted to main-chain polymers [Citation45], but the intermediates have since been more widely employed in investigations of differentially substituted unsymmetrical triphenylenes to probe substituent effects and their influence on mesophase behaviour.
Scheme 2. Synthesis of unsymmetrically substituted hexaalkoxytriphenylenes by reaction of a pre-formed tetraalkoxybiphenyl with dialkoxybenzene.
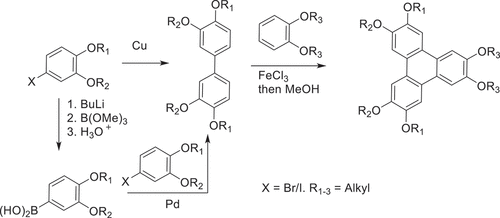
At around the same time, the application of transition-metal catalysed cross-coupling chemistry in biaryl synthesis, and synthesis in general, was gaining significant momentum. Hird and co-workers [Citation46] used Suzuki-Miyaura cross-coupling strategies to efficiently access biphenyl intermediates to deliver unsymmetrical triphenylenes, and the combination of cross-coupling with oxidative approaches has proved highly effective. A significant advantage of the cross-coupling approach is that the electronic character of the reacting components does not need to be matched and electron-rich systems (required for oxidative coupling) are not needed. Triphenylenes can be synthesised with free β-positions (e.g. 4) through construction of o-terphenyl intermediates, and they can be post-functionalised by simple electrophilic aromatic substitution [Citation47]. Bromination, for example, conveniently gives intermediates suitable for nucleophilic aromatic substitution [Citation47] or subsequent cross-coupling [Citation48–51]. shows one example where this sequence was employed to produce an unsymmetrical triphenylene 6 that exhibits the rare helical columnar mesophase at room temperature [Citation47].
Scheme 3. An example of synthesis of a lightly substituted triphenylene via an o-terphenyl, and subsequent functionalisation to give a mesogenic hexasubstituted material [Citation47].
![Scheme 3. An example of synthesis of a lightly substituted triphenylene via an o-terphenyl, and subsequent functionalisation to give a mesogenic hexasubstituted material [Citation47].](/cms/asset/30110d65-1653-4666-b7f0-d34f8cb2dad6/tlct_a_2259856_sch0003_b.gif)
The range of triphenylene materials available for design has therefore been significantly expanded and has particularly opened the way for investigation of new structural motifs to probe factors controlling columnar stability and to encourage nematic mesophase formation. A wide range of differentially (hexa)substituted triphenylenes have now been investigated, sequentially replacing alkoxy substituents on the parent hexaalkoxytriphenylenes. The studies reveal some general trends. Mesophase formation is often supported so long as conjugating substituents are introduced (essentially extending the core) [Citation50]. The reduced symmetry of the systems also typically results in a suppression of crystallisation leading to glassy columnar phases. shows representative examples that demonstrate how relatively minor changes can impact mesophase formation and stability.
Figure 4. Examples of structural variation in unsymmetrically substituted triphenylenes (Hx = n-hexyl) [Citation47–50].
![Figure 4. Examples of structural variation in unsymmetrically substituted triphenylenes (Hx = n-hexyl) [Citation47–50].](/cms/asset/94bea919-ab47-4c7d-afd0-4f10a96887f7/tlct_a_2259856_f0004_b.gif)
The contrasting mesophase behaviour of the triphenylene hexaethers (columnar) and the hexaarylesters (nematic) has been highlighted earlier. Studies investigating mixed ether-ester linkages in the triphenylene series have been initiated recently. Replacing a single ether of the parent hexaalkoxytriphenylene with aryl ester substituents so far leads only to columnar mesophases. In fact, in the initial study, we find that the mesophase range is remarkably insensitive to the size and character of the aryl ester employed, implying that the single aromatic unit can be easily accommodated in the chain region between columns [Citation52]. This investigation has now been extended to include selected examples of isomeric diesters, reported here for the first time, and again columnar mesophases only are observed ().
Figure 5. Triphenylenes with mixed ether and ester substituents showing only columnar mesophases (Hx = n-hexyl).
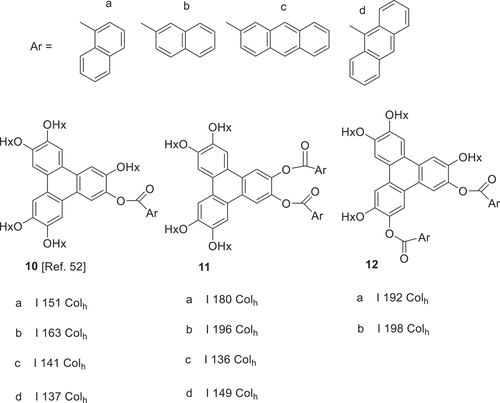
While columnar packing/assembly is effectively constrained in examples of triphenylene hexaaryl esters, an alternative approach to generating nematic mesophases in triphenylene discotics has emerged whereby columnar packing is disfavoured by the formation of dimeric systems, typically linked by rigid bridges. Some examples are shown in Here the behaviour contrasts with dimeric systems linked by flexible spacer chains of appropriate length, where columnar mesophase formation is preserved [Citation53–55]. The first examples of this motif were the diacetylene-bridged triphenylenes reported by Kumar and Varshney [Citation56]. Twinned systems – two triphenylene cores linked by two rigid bridges to form a macrocycle – show a strong tendency towards nematic behaviour [Citation57–59]. Complementary to the design features that lead to nematic phases in the triphenylene hexaaryl esters, here the formation of columnar assemblies also leads to free space (through the centre of the column in this case) favouring the slipped (ND) arrangement [Citation57].
Figure 6. Triphenylene dimers and twins, linked through rigid bridges, showing nematic mesophases [Citation56–59].
![Figure 6. Triphenylene dimers and twins, linked through rigid bridges, showing nematic mesophases [Citation56–59].](/cms/asset/07a79299-1aee-4b27-9503-73f8e9ad5396/tlct_a_2259856_f0006_b.gif)
Further examples of dimers where the bridges are systematically engineered to modify core–core separation and conformational freedom have been investigated more recently, focusing on aryl ester [Citation52] and aryl alkyne [Citation60] links (). In the diester (phthalate) series, mesophase behaviour is completely suppressed when the core–core separation is enforced to be too short (o-phthalate where face-to-face packing is difficult to achieve) or too long (the stilbene system). The 2,5-thiophene diester shows an enantiotropic ND phase, while the similarly bent 2,7-naphthaloate bridge gives a rare example of a material that forms a columnar phase on cooling from the nematic (albeit alongside glass formation).
Figure 7. The influence of linker on mesophase behaviour in ester-linked triphenylene dimers [Citation52].
![Figure 7. The influence of linker on mesophase behaviour in ester-linked triphenylene dimers [Citation52].](/cms/asset/5cd58b77-a3f7-4168-9703-24fc3b24b9dc/tlct_a_2259856_f0007_b.gif)
The alkyne linked dimers [Citation60] are particularly interesting. The single aryl-diacetylene bridge between the triphenylenes provides materials that have intermediate conformational freedom compared to, for example, the complementary aryl diester series and rigid twinned (double bridge) systems. Similar to the o-phthalate system [Citation52], the 1,2-phenyl diacetylene bridge does not support any mesophase behaviour. However, opening the linking angle leads to mesophase formation over a wide temperature range. The unique combination of fixed core–core separation alongside limited conformational freedom provides a series of discotic materials that each form both nematic and columnar mesophases () [Citation60].
Figure 8. Aryl diacetylene linked triphenylene dimers showing both columnar and nematic mesophases [Citation60].
![Figure 8. Aryl diacetylene linked triphenylene dimers showing both columnar and nematic mesophases [Citation60].](/cms/asset/d03a6697-a9fb-49b6-b24a-50077acba447/tlct_a_2259856_f0008_b.gif)
Experimental
Synthesis of triphenylene-2,3-bis(1-naphthoate) ester 11a (general procedure)
2,3-Dihydroxy-6,7,10,11-tetrakis(hexyloxy)triphenylene (100 mg, 0.15 mmol), and 1-naphthoyl chloride (0.069 g, 0.36 mol) were stirred in dry CH2Cl2 (10 ml) at 0°C. Then, dry NEt3 (2 ml) was added slowly. After 2 h, the solvents were evaporated and the residue precipitated from methanol to give a pale yellow solid (0.055 g, 38%). 1H NMR (400 MHz, Chloroform-d) δ 9.05–9.00 (m, 2 H), 8.47 (s, 2 H), 8.40 (dd, J = 7.4, 1.3 Hz, 2 H), 7.94 (dt, J = 8.3, 1.1 Hz, 2 H), 7.89 (s, 2 H), 7.83 (s, 2 H), 7.82–7.79 (m, 2 H), 7.53–7.46 (m, 4 H), 7.25 (dd, J = 8.2, 7.3 Hz, 2 H), 4.25 (t, J = 6.6 Hz, 4 H), 4.21 (t, J = 6.6 Hz, 4 H),1.96–1.86 (m, 8 H), 1.58–1.43 (m, 8 H), 1.39–1.26 (m, 16 H), 0.93 (t, J = 7.2 Hz, 6 H), 0.88 (t, J = 7.2 Hz, 6 H). 13C NMR (101 MHz, CD2Cl2) δ 165.15, 149.92, 149.23, 141.40, 134.39, 133.80, 131.46, 131.38, 128.59, 128.11, 127.86, 126.36, 125.42, 125.00, 124.37, 124.18, 122.54, 117.61, 106.80, 106.59, 69.47, 69.25, 31.69, 31.63, 29.43, 29.37, 25.85, 25.78, 22.67, 22.62, 13.82, 13.78.
Diesters 11 and 12 were prepared following the same procedure using the 2,3-dihydroxytriphenylene (for 11) or the 3,6-dihydroxytriphenylene (for 12) and the appropriate acid chloride.
2,3-bis(2-naphthoate) ester 11b
(0.05 g, 34%). 1H NMR (400 MHz, Chloroform-d) δ 8.71 (br s, 2 H), 8.50 (s, 2 H), 8.13 (dd, J = 7.4, 1.3 Hz, 2 H), 7.88 (s, 2 H), 7.84 (s, 2 H), 7.78 (d, J = 8.3 Hz, 2 H), 7.77 (d, J = 8.2 Hz, 2 H), 7.72 (d, J = 8.5 Hz, 2 H), 7.54 (dt, J = 8.0, 1.0 Hz, 2 H), 7.43 (dt, J = 8.0, 1.0 Hz, 2 H), 4.26 (t, J = 6.6 Hz, 4 H), 4.22 (t, J = 6.6 Hz, 4 H), 1.99–1.88 (m, 8 H), 1.63–1.51 (m, 8 H), 1.46–1.29 (m, 16 H), 0.93 (t, J = 7.2 Hz, 6 H), 0.89 (t, J = 7.2 Hz, 6 H). 13C NMR (101 MHz, CD2Cl2) δ 165.15, 149.92, 149.23, 141.40, 134.39, 133.80, 131.46, 131.38, 128.59, 128.11, 127.86, 126.36, 125.42, 125.00, 124.37, 124.18, 122.54, 117.61, 106.80, 106.59, 69.47, 69.25, 31.69, 31.63, 29.43, 29.37, 25.85, 25.78, 22.67, 22.62, 13.82, 13.78.
2,3-bis(2-anthracenoate) ester 11c
(0.051 g, 32%). 1H NMR (400 MHz, Chloroform-d) 8.89 (s, 2 H), 8.59 (s, 2 H), 8.24 (s, 2 H), 8.16 (s, 2 H), 8.06 (dd, J = 8.9, 1.7 Hz, 2 H), 7.93 (s, 2 H), 7.90 (s, 2 H), 7.88 (s, 2 H), 7.86 (s, 2 H), 7.68 (d, J = 8.5 Hz, 2 H), 7.46 (t, J = 7.0 Hz, 2 H), 7.3t (t, J = 7.0 Hz, 2 H), 4.27 (t, J = 6.6 Hz, 4 H), 4.24 (t, J = 6.6 Hz, 4 H), 2.01–1.90 (m, 8 H), 1.65–1.51 (m, 8 H), 1.46–1.32 (m, 16 H), 0.95 (t, J = 7.0 Hz, 4 H), 0.90 (t, J = 7.0 Hz, 4 H). 13C NMR (101 MHz, CDCl3) δ 164.99, 149.75, 149.19, 141.29, 133.65, 133.18, 132.57, 131.82, 130.01, 128.83, 128.70, 128.33, 128.01, 127.86, 126.69, 126.07, 125.78, 125.59, 124.31, 123.92, 122.93, 117.40, 107.03, 69.66, 69.31, 31.72, 31.66, 29.43, 29.35, 25.87, 25.83, 22.68, 22.64, 14.07, 14.03 (2 signals unresolved).
2,3-bis(9-anthracenoate) ester 11d
(0.03 g, 19%). 1H NMR (400 MHz, CD2Cl2) δ 8.82 (s, 2 H), 8.47 (s, 2 H), 8.18 (d, J = 9.0 Hz, 4 H), 8.07 (s, 2 H), 7.94 (d, J = 9.0 Hz, 4 H), 7.92 (s, 2 H), 7.34 (t, J = 8.3 Hz, 4 H), 7.0 (t, J = 8.3, 4 H), 4.33 (t, J = 6.7 Hz, 4 H), 4.32 (t, J = 6.7 Hz, 4 H), 2.05–1.96 (m, 8 H), 1.70–1.38 (m, 24 H), 0.98 (t, J = 7.2 Hz, 4 H), 0.94 (t, J = 7.2 Hz, 4 H). 13C NMR (101 MHz, CDCl3) δ 167.38, 150.10, 149.24, 141.06, 130.68, 130.37, 128.81, 128.27, 128.10, 126.91, 125.79, 125.32, 124.87, 124.63, 122.85, 117.40, 107.75, 106.98, 69.73, 69.64, 31.94, 31.72, 29.67, 29.42, 29.37, 25.89, 25.87, 22.69, 22.66, 14.13, 14.08.
3,6-bis(1-naphthoate) ester 12a
(0.043 g, 61%). 1H NMR (500 MHz, Chloroform-d) 9.03 (d, J = 8.7 Hz, 2 H), 8.46 (dd, J = 7.3, 1.3 Hz, 2 H), 8.00 (d, J = 8.0 Hz, 2 H), 7.95 (d, J = 8.0 Hz, 2 H), 7.92 (s, 2 H), 7.91 (d, J = 7.5 Hz, 2 H), 7.80 (s, 2 H), 7.58–7.46 (m, 6 H), 4.20 (t, J = 6.4 Hz, 4 H), 4.15 (t, J = 6.5 Hz, 4 H), 1.84–1.75 (m, 4 H), 1.74–1.67 (m, 4 H), 1.46–1.38 (m, 4 H), 1.36–1.30 (m, 4 H), 1.29–1.25 (m, 8 H), 1.13–0.98 (m, 8 H), 0.84 (t, J = 7.0 Hz, 6 H), 0.64 (t, J = 7.2 Hz, 6 H). 13C NMR (126 MHz, CDCl3) 165.81, 149.76, 149.48, 140.12, 133.91, 133.88, 131.69, 131.15, 128.54, 127.89, 127.84, 126.46, 126.31, 126.08, 124.58, 124.10, 122.95, 117.07, 107.63, 105.98, 69.61, 68.87, 31.72, 31.55, 29.41, 29.40, 25.84, 25.74, 22.65, 22.44, 14.07, 13.86.
3,6-bis(2-naphthoate) ester 12b
(0.04 g, 54%). 1H NMR (500 MHz, Chloroform-d) 8.86 (br s, 2 H), 8.26–8.23 (m, 6 H), 8.00 (d, J = 8.0 Hz, 2 H), 7.95 (d, J = 8.0 Hz, 2 H), 7.92 (s, 2 H), 7.91 (d, J = 7.5 Hz, 2 H), 7.90 (s, 2 H), 7.62 (t, J = 8.5 Hz, 2 H), 7.57 (t, J = 8.5 Hz, 2 H), 4.27 (t, J = 6.4 Hz, 4 H), 4.24 (t, J = 6.5 Hz, 4 H), 1.99–1.92 (m, 4 H), 1.79–1.71 (m, 4 H), 1.63–1.55 (m, 4 H), 1.46–1.38 (m, 12 H), 1.22–1.09 (m, 8 H), 0.94 (t, J = 7.2 Hz, 6 H), 0.71 (t, J = 7.2 Hz, 6 H).; 13C NMR (126 MHz, CDCl3) 163.89, 148.65, 148.41, 139.15, 132.31, 129.24, 128.64, 127.44, 126.73, 122.99, 121.88, 115.83, 106.56, 105.23, 68.56, 67.91, 30.69, 30.49, 28.40, 28.23, 24.84, 24.63, 21.64, 21.48, 13.03, 12.89.
Conclusions
The development and commercial application of the (cyano) biphenyls can be seen to have influenced the later development of discotic materials where the triphenylene nucleus has become the most widely studied core. The search for discotic nematic materials was originally driven by interest in the potential of discotics to be active, switchable components in displays, but they proved both difficult to design and synthesise, and their properties did not compete. Nevertheless, nematic discotics have played vital role in display devices as a passive, thin-film optical compensating layers to widen viewing angles. Nematic discotics have remained intriguing within liquid crystal science. They are relatively rare and studies now indicate that columnar packing needs to be disfavoured by molecular design. This can be achieved by construction of systems where the side-chains themselves prevent columnar packing by providing a thick ring around the core (the aryl esters and phenyl acetylenes) or by rigidly linking cores such that they cannot achieve face-to-face assembly (rigid dimers and twins). Some examples are now known that show both columnar and nematic mesophases. The later development of more intricate molecular designs has been made possible by advances in synthetic chemistry that can deliver unsymmetrical systems. The chemistry here also sees a parallel with bi- (and ter-)phenyl development, not least because their pre-synthesis has been key to developing versatile new routes to triphenylene discotics. In this regard, the combination of transition metal catalysed cross-coupling and oxidative cyclisation has been proved the most powerful.
Acknowledgments
We thank Almajmaah University (SA) and Taibah University (SS) for their financial support.
Disclosure statement
No potential conflict of interest was reported by the author(s).
Additional information
Funding
References
- Chandrasekhar S, Sadashiva BK, Suresh KA. Liquid crystals of disc-like molecules. Pramana J Phys. 1977;9(5):471–480. doi: 10.1007/BF02846252
- Cammidge AN, Bushby RJ. Discotic liquid crystals: synthesis and structural features. In: Demus D, Goodby J, Gray G, Spiess H Vill V, editors. Handbook of liquid crystals. Vol. 2. Weinheim: Wiley-VCH; 1998. p. 693–748. doi: 10.1002/9783527620623.ch4
- Kumar S. Chemistry of discotic liquid crystals. Florida: CRC Press; 2010.
- Bock H, Helfrich W. Two ferroelectric phases of a columnar dibenzopyrene. Liq Cryst. 1995;18(3):387–399. doi: 10.1080/02678299508036636
- Kumar S. Recent developments in the chemistry of triphenylene-based discotic liquid crystals. Liq Cryst. 2004;31(8):1037–1059. doi: 10.1080/02678290410001724746
- Cammidge AN, Gopee H. Design and synthesis of nematic phases formed by disk-like molecules. In: Goodby J, Collings P, Kato T, Tschierske C, Gleeson H, Raynes P, editors. Handbook of liquid crystals. 2nd ed. Vol. 3. Weinheim: Wiley-VCH; 2014. p. 293–334. doi: 10.1002/9783527671403.hlc047
- Hunter CA, Sanders JKM. The nature of pi-pi interactions. J Am Chem Soc. 1990;112:5525–5534. doi: 10.1021/ja00170a016
- Dirand M, Bouroukba M, Briard AJ, et al. Temperatures and enthalpies of (solid+solid) and (solid+liquid) transitions of n-alkanes. J Chem Thermodyn. 2002;34(8):1255–1277. doi: 10.1006/jcht.2002.0978
- Destrade C, Tinh NH, Gasparoux H, et al. Disc-like mesogens: a classification. Mol Cryst Liq Cryst. 1981;71(1–2):111–135. doi: 10.1080/00268948108072721
- Tinh NH, Destrade C, Gasparoux H. Nematic disc-like liquid crystals. Phys Lett A. 1979;72(3):251–254. doi: 10.1016/0375-9601(79)90019-7
- Phillips TJ, Jones JC, McDonnell DG. On the influence of short range order upon the physical properties of triphenylene nematic discogens. Liq Cryst. 1993;15(2):203–215. doi: 10.1080/02678299308031951
- Hindmarsh P, Hird M, Styring P, et al. Lateral substitution in the peripheral moieties of triphenylen-2,3,6,7,10,11-hexayl hexakis(4-alkoxybenzoate)s: dimethyl-substituted systems. J Mater Chem. 1993;3:1117–1128. doi: 10.1039/jm9930301117
- Praefcke K, Kohne B, Singer D. Hexaalkynyltriphenylene: a new type of nematic-discotic hydrocarbon. Angew Chem Int Ed Engl. 1990;29:177–179. doi: 10.1002/anie.199001771
- Kohne B, Praefcke K. Liquid-crystallins compounds. 43. Hexaalkynylbenzene derivatives, the 1st hydrocarbon columnar-discotic or nematic-discotic liquid crystals. Chimia. 1987;41:196–198. doi: 10.1002/chin.198750161
- Toyota S. Rotational isomerism involving acetylene carbon. Chem Rev. 2010;110(9):5398–5424. doi: 10.1021/cr1000628
- Lozman OR, Bushby RJ, Vinter JG. Complementary polytopic interactions (CPI) as revealed by molecular modelling using the XED force field. J Chem Soc Perkin Trans. 2001;2(9):1446–1452. doi: 10.1039/b103390p
- Bushby RJ, Kawata K. Liquid crystals that affected the world: discotic liquid crystals. Liq Cryst. 2011;38(11–12):1415–1426. doi: 10.1080/02678292.2011.603262
- Mori H, Itoh Y, Nishiura Y, et al. Performance of a novel optical compensation film based on negative birefringence of discotic compound for wide-viewing-angle twisted-nematic liquid-crystal displays. Jpn J Appl Phys. 1997;36:143–147. Part 1. doi: 10.1143/JJAP.36.143
- Lawson KD, Flautt TJ. Magnetically oriented lyotropic liquid crystalline phases. J Am Chem Soc. 1967;89:5489–5491. doi: 10.1021/ja00997a054
- Charvolin J, Levelut AM, Samulski ET. Lyotropic nematics: molecular aggregation and susceptibilities. J De Phys Lett. 1979;40:L587–L592. doi: 10.1051/jphyslet:019790040022058700
- Holmes MC, Charvolin J. Smectic-nematic transition in a lyotropic liquid crystal. J Phys Chem. 1984;88(4):810–818. doi: 10.1021/j150648a039
- Boden N, Jackson PH, McMullen K, et al. Are “nematic” amphiphilic liquid crystalline mesophases thermodynamically stable? Chem Phys Lett. 1979;65(3):476–479. doi: 10.1016/0009-2614(79)80275-4
- Forrest BJ, Reeves LW. New lyotropic liquid crystals composed of finite nonspherical micelles. Chem Rev. 1981;81:1–14. doi: 10.1021/cr00041a001
- Saupe A. On the structure and the physical properties of micellar nematics. Il Nuovo Cimento D. 1984;3(1):16–29. doi: 10.1007/BF02452199
- Boden N, Bushby RJ, Hardy C. New mesophases formed by water soluble discoidal amphiphiles. J De Phys Lett. 1985;46:L325–L328. doi: 10.1051/jphyslet:01985004607032500
- Boden N, Bushby RJ, Ferris L, et al. Designing new lyotropic amphiphilic mesogens to optimize the stability of nematic phases. Liq Cryst. 1986;1(2):109–125. doi: 10.1080/02678298608086498
- Akinshina A, Walker M, Wilson MR, et al. Thermodynamics of the self-assembly of non-ionic chromonic molecules using atomistic simulations. The case of TP6EO2M in aqueous solution. Soft Matter. 2015;11:680–691. doi: 10.1039/C4SM02275K
- Henderson JR. Linear aggregation beyond isodesmic symmetry. J Chem Phys. 2009;130(4):045101. doi: 10.1063/1.3063099
- Boden N, Bushby RJ, Hardy C, et al. Phase behaviour and structure of a non-ionic discoidal amphiphile in water. Chem Phys Lett. 1986;123(5):359–364. doi: 10.1016/0009-2614(86)80021-5
- Potter TD, Walker M, Wilson MR. Self-assembly and mesophase formation in a non-ionic chromonic liquid crystal: insights from bottom-up and top-down coarse-grained simulation models. Soft Matter. 2020;16(41):9488–9498. doi: 10.1039/D0SM01157F
- Perez-Calm A, Esquena J, Salonen LM, et al. Pegylated perylene bisimides: chromonic building blocks for the aqueous synthesis of nanostructured silica materials. J Mol Liq. 2021;325:114657. doi: 10.1016/j.molliq.2020.114657
- Masters A. Chromonic liquid crystals: more questions than answers. Liq Cryst Today. 2016;25(2):30–37. doi: 10.1080/1358314X.2016.1149925
- McKeown NB, Painter J. Lyotropic and thermotropic mesophase formation of novel tetra- [oligo(ethyleneoxy)]-substituted Phthalocyanines. J Mater Chem. 1994;4:1153–1156. doi: 10.1039/jm9940401153
- Boden N, Bushby RJ, Donovan K, et al. 2,3,7,8,12,13-Hexakis[2-(2-methoxyethoxy)ethoxy]tricycloquinazoline: a discogen which allows enhanced levels of n-doping. Liq Cryst. 2001;28:1739–1748. doi: 10.1080/02678290110082383
- Al-Lawati ZH, Alkhairalla B, Bramble JP, et al. Alignment of discotic lyotropic liquid crystals at hydrophobic and hydrophilic self-assembled monolayers. J Phys Chem C. 2012;116(23):12627–12635. doi: 10.1021/jp302634r
- Lydon J. Chromonic review. J Mater Chem. 2010;20(45):10071–10099. doi: 10.1039/b926374h
- Hartshorne NH, Woodard GD. Mesomorphism in the System disodium chromoglycate-water. Mol Cryst Liq Cryst. 1973;23(3–4):343–368. doi: 10.1080/15421407308083381
- Attwood TK, Lydon JE, Jones F. The chromonic phases of dyes. Liq Cryst. 1986;1(6):499–507. doi: 10.1080/02678298608086274
- Boden N, Borner RC, Bushby RJ, et al. The synthesis of triphenylene-based discotic mesogens New and improved routes. Liq Cryst. 1993;15:851–858. doi: 10.1080/02678299308036504
- Kumar S, Lakshmi B. A convenient and economic method for the synthesis of monohydroxy-pentaalkoxy- and hexaalkoxytriphenylene discotics. Tetrahedron Lett. 2005;46(15):2603–2605. doi: 10.1016/j.tetlet.2005.02.099
- Ban J, Chen S, Zhang H. Synthesis and phase behaviour of mesogen jacketed liquid crystalline polymer with triphenylene discotic liquid crystal mesogen unit in side chains. RSC Adv. 2014;4:54158–54167. doi: 10.1039/C4RA07824A
- Kong X, He Z, Gopee H, et al. Improved synthesis of monohydroxytriphenylenes (MHTs) – important precursors to discotic liquid crystals. Tetrahedron Lett. 2011;52:77–79. doi: 10.1016/j.tetlet.2010.10.152
- Musgrave OC, Webster CJ. Formation of polycyclic quinones in oxidations of 3,3‘,4,4’-tetramethoxybiphenyl and veratrole. J Chem Soc Chem Commun. 1969;712–713. doi: 10.1039/C29690000712
- Boden N, Bushby RJ, Cammidge AN. A quick-and-easy route to unsymmetrically substituted derivatives of triphenylene: preparation of polymeric discotic liquid crystals. J Chem Soc Chem Commun. 1994;4:465–466. doi: 10.1039/c39940000465
- Boden N, Bushby RJ, Cammidge AN. Triphenylene-based discotic-liquid-crystalline polymers: a universal, rational synthesis. J Am Chem Soc. 1995;117(3):117;924–927. doi: 10.1021/ja00108a009
- Goodby JW, Hird M, Toyne KJ, et al. A novel, efficient and general synthetic route to unsymmetrical triphenylene mesogens using palladium-catalysed cross-coupling reactions. J Chem Soc Chem Commun. 1994;14:1701–1702. doi: 10.1039/c39940001701
- Cammidge AN, Gopee H. Structural factors controlling the transition between columnar-hexagonal and helical mesophase in triphenylene liquid crystals. J Mater Chem. 2001;11(11):2773–2783. doi: 10.1039/b103450m
- Cammidge AN, Gopee H. Mixed alkyl-alkoxy triphenylenes. Mol Cryst Liq Cryst. 2003;397:417–428. doi: 10.1080/714965603
- Cammidge AN, Gopee H. Synthesis and liquid crystal properties of mixed alkynyl-alkoxy-triphenylenes. Liq Cryst. 2009;36:809–819. doi: 10.1080/02678290903063000
- Cammidge AN, Chausson C, Gopee H, et al. Probing the structural factors influencing columnar mesophase formation and stability in triphenylene discotics. Chem Commun. 2009;47:7375–7377. doi: 10.1039/b913678a
- Cammidge AN. The effect of size and shape variation in discotic liquid crystals based on triphenylene cores. Philos Trans R Soc A 2006;364(1847):2697–2708. doi: 10.1098/rsta.2006.1847
- Alanazi BN, Alruwaili AM, Beskeni RD, et al. Pentalkoxytriphenylene monoester and their dyads; structural factors influencing columnar and nematic mesophase behaviour. Liq Cryst. 2022;49:1174–1183. doi: 10.1080/02678292.2021.1993456
- Kumar S. Triphenylene-based discotic liquid crystal dimers, oligomers and polymers. Liq Cryst. 2005;32(9):1089–1113. doi: 10.1080/02678290500117415
- Boden N, Bushby RJ, Cammidge AN, et al. The creation of long-lasting glassy columnar discotic liquid crystals using “dimeric” discogens. J Mater Chem. 1999;9:1391–1402. doi: 10.1039/a810045d
- Wang Y, Zhang C, Wu H, et al. Synthesis and mesomorphism of triphenylene-based dimers with a highly ordered columnar plastic phase. J Mater Chem C. 2014;2(9):1667–1674. doi: 10.1039/c3tc31986e
- Kumar S, Varshney SK. Design and synthesis of discotic nematic liquid crystals. Org Lett. 2002;4(2):157–159. doi: 10.1021/ol010200v
- Zhang L, Gopee H, Hughes DL, et al. Antiaromatic twinned triphenylene discotics showing nematic phases and 2-dimensional π-overlap in the solid state. Chem Commun. 2010;46:4255–4257.
- Zhang L, Hughes DL, Cammidge AN. Discotic triphenylene twins linked through thiophene bridges: controlling nematic behavior in an intriguing class of functional organic materials. J Org Chem. 2012;77(9):4288–4297. doi: 10.1021/jo3002886
- Cammidge AN, Turner RJ, Beskeni RD, et al. A modified route to unsymmetrically substituted triphenylenes, new functionalised derivatives and twins, and the smallest reported triphenylene mesogen. Liq Cryst. 2017;44:2018–2028. doi: 10.1080/02678292.2017.1346210
- Alsahli AO, Cammidge AN. Synthesis and mesophase properties of triphenylene dimers linked through linear and bent alkyne-aryl-alkyne bridges. Eur J Org Chem. 2022;2022:e202200990. doi: 10.1002/ejoc.202200990