Abstract
To understand the effect of cyanobacterial blooms on nitrogen transformations in eutrophic Lake Taihu, the variation in nitrate concentration during a bloom was observed in a field simulation experiment. This result showed that the cyanobacterial bloom might cause nitrate depletion in an aquatic ecosystem. To further investigate this field result, nitrate transformations after the addition of cyanobacteria collected from Lake Taihu were traced in laboratory microcosms with the 15N isotope addition method. About 81.2% and 98.4% of nitrate was lost when 2 × 109 and 4 × 109 cells L−1 of cyanobacteria were added, respectively. The nitrate concentration decrease followed first-order kinetics with the rate constant 0.23 and 1.41 d−1 in the two treatments, respectively. Conventional denitrification was found to play a major role in the nitrate removal process while other pathways (e.g., dissimilatory nitrate reduction to ammonium [DNRA] and assimilation of nitrate into microbial biomass) were negligible. It was likely that the cyanobacterial respiration as well as their decomposition resulted in anoxic conditions and that cyanobacteria also served as a carbon source for denitrification. Based on the above results, it was estimated that 109 cells of cyanobacteria were enough for denitrifiers to remove 0.53 mmol nitrate in the eutrophic lake ecosystem. Therefore, cyanobacterial blooms have the potential for nitrogen removal by denitrification and this process could cause nitrogen limitation of primary production in summer in Lake Taihu. This interactive relationship of nitrogen and cyanobacteria potentially constitutes a negative feedback for mitigating cyanobacterial blooms in this eutrophic lake environment.
Introduction
Anthropogenic inputs of nitrogen to the environment have been increasing since the industrial revolution (Galloway et al. Citation2008), a substantial amount of which have reached aquatic systems and brought about numerous environmental problems (Duce et al. Citation2008). Lakes and reservoirs have been recognized as important sites for nitrogen removal, accounting for about 33% of the total global nitrogen removal (Harrison et al. Citation2009). However, excess external nitrogen loading into lakes often leads to the proliferation of phytoplankton (Valiela et al. Citation1997). Cyanobacterial blooms have afflicted lakes all over the world (Hallegraeff Citation1993) over the past few decades. While tremendous efforts have been made to understand the effects of nutrients on the abundance and composition of phytoplankton in lakes (Xie et al. Citation2003; Vrede et al. Citation2009) and the potentially harmful effects of cyanobacterial blooms (Donaghay and Osborn Citation1997; Smayda Citation1997), it is less well known whether phytoplankton can change the nutrient dynamics in lake ecosystems.
Four processes have been identified that reduce nitrogen concentrations in water bodies: denitrification, anaerobic ammonium oxidation (anammox), sedimentation and uptake by aquatic plants (Dalsgaard et al. Citation2005; Thouvenot et al. Citation2009). Of these, only denitrification and anammox lead to nitrogen export from the aquatic ecosystem. Anammox often occurs at the interface between surface water and sediment porewater and be limited to areas that are relatively low in labile carbon, which often is not the case for near-surface freshwater sediments that support high biological productivity (Burgin and Hamilton Citation2007). Thus, denitrification is typically of higher importance in freshwater ecosystem.
The first step of denitrification is the reduction of nitrate to nitrite. Then, the nitrite either can be reduced to ammonia (dissimilatory nitrate reduction to ammonia, DNRA) or sequentially transformed to nitric oxide, nitrous oxide, and nitrogen gas (conventional denitrification). Denitrification can be influenced by different environmental factors such as nitrate supply, pH, dissolved oxygen (DO), dissolved organic carbon and phosphorus, temperature, light, water residence time, and the presence of plants (Golterman Citation2004). The presence of algae may also strongly influence denitrification. For example, readily-degradable benthic algae, serving as a nitrate and organic carbon source, can facilitate the denitrification processes (McMillan et al. 2010; Sirivedhin and Gray Citation2006). Some algae (e.g., diatoms) even have a synergistic effect on denitrifying bacteria (Ishida et al. Citation2008).
Lake Taihu is a large shallow lake with an area of 2338 km2, a mean depth of 1.9 m and maximum depth of less than 3 m. There are annual microcystis blooms induced by eutrophication. Affected by the southeast monsoon winds in summer, large numbers of cyanobacteria accumulate at Meiliang Bay. As a result the density of cyanobacteria in this bay can be over 109 cells L−1 (Li Citation1999; Ye et al. Citation2010). The total nitrogen (TN) concentrations in Meiliang Bay in summer and fall, the period when cyanobacterial blooms break out, are always lower than in other seasons, which was suggested to be largely the result of rainfall dilution and phytoplankton uptake (Xu et al. Citation2010). McCarthy et al. (Citation2007) found that the denitrification rate was far higher in Meiliang Bay than in the main lake region. They proposed that nitrate limitation was the primary reason for the lower denitrification rate observed in the main lake. However, it is uncertain whether there is any interrelationship between cyanobacterial blooms and low TN or high denitrification rate in Meiliang Bay. The only report so far was by Wang et al. (Citation2006) who observed excessive N2O emission during cyanobacterial bloom periods in Lake Taihu. It was thus suggested that cyanobacterial bloom played an important role in the emission of N2O.
To reveal the inherent relationship between the nitrogen concentration and cyanobacterial blooms, a method capable of tracing the transformations of nitrogen is essential. One of the most important tools is the 15N isotope technique that has been widely used in nitrogen cycling research (Nielsen Citation1992; Halm et al. Citation2009) and includes the natural abundance method and isotope addition method. The natural abundance method is often used in large scale research with long duration, while 15N addition is more frequently applied to investigate short-term variation of different nitrogen species in pots or small catchments (Robinson Citation2001). Therefore, a 15N- addition method was selected for our laboratory microcosm experiment. The overall objective of this study was to determine if cyanobacterial blooms affected nitrogen removal and to investigate the mechanism involved.
Methods
The cyanobacterial sample was collected from the eastern side (31°28′23″ N; 120°13′26″ E) in Meiliang Bay of Lake Taihu during a cyanobacterial bloom period in July, 2009. Since the thickness of the cyanobacterial layer was about 20 cm, the cyanobacterial sample was simply collected with a container and kept cool until it was used in laboratory experiments. In addition, water samples (0.5 m depth) were collected with four 25-L bottles at the same site. Physical parameters of the water samples, including temperature, DO, pH, and electrical conductivity (EC), were measured using a multisensor probe (YSI-6600, Yellow Springs Instrument, Yellow Springs, Ohio, USA).
After being well mixed, subsamples of the algae sample were taken and diluted to 1 L with collected lake water. The algae were settled by 30 mL of 1% Lugol's iodine solution (Wei and Qi Citation2002). Then, 0.1 mL of the settled sample was counted directly in a 0.1 mL counting chamber at 400 × under a microscope. The algae were counted on three replicate slides and the counts averaged. Colonial Microcystis cells were separated using an ultrasonic crusher. Taxonomic identification was made according to Hu et al. (Citation1980). After being washed by distilled water, a sample of cyanobacteria were freeze-dried to determine the dry weight per cell.
To explore the denitrification potential in Lake Taihu during the cyanobacterial bloom period, three translucent tanks filled with 1 L of collected lake water and some cyanobacteria were fastened at the sampling site (0.2 m depth) in Meiliang Bay. The cyanobacterial density was adjusted to 4 × 109 cells as suggested by Pan et al. (Citation2006). Three tanks without addition of cyanobacteria were used as control. The nitrate, nitrite and dissolved oxygen concentrations in each tank were measured each day at 08:00 during a 6-d period. On certain days, the diurnal variation of DO (0.5 m depth of water body) in the cyanobacterial accumulation area near the tanks was quantified by measuring DO every two hours from 22:00 to 12:00 the following day.
To confirm the effect of cyanobacteria on denitrification, nitrate transformation in the presence of cyanobacteria was traced in laboratory microcosms. The cyanobacteria collected from Meiliang Bay were added to 6 L of collected lake water to achieve cyanobacterial densities of either 2 × 109 or 4 × 109 cells L−1. The treatment without addition of cyanobacteria was used as control. Each treatment had three replicates and was cultured at 25 ± 1°C with a light intensity of 35 µmol photons m−2 s−1 and a light/dark cycle of 12 h/12 h. 15N-labeled KNO3 (20 atom % 15N, from Research Institute of Chemical Industry of Shanghai, China) was added to each microcosm to a final -N concentration of about 2.1 mmol L−1. The experimental system is diagrammed in . After passing through 300 mL dilute sulfuric acid (1 mol L−1) to remove ammonium, air (0.1 L min−1) was blown over the water in the experimental microcosm and then bubbled through 50 mL of 2% boric acid to absorb the NH3 evolved from the experimental microcosm.
Figure 1. Laboratory experiment system containing the microcosm. After passing through dilute sulfuric acid to remove the ammonium, air (0.1 L min−1) was blown across the water surface and bubbled through 2% boric acid to absorb NH3 emitted from the experimental system. Sampling outtake was used to collect the gas sample for NO, N2O and N2.
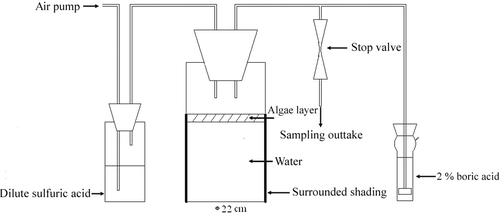
After an initial DO measurement, 10-mL samples were taken by suction from three different depths in each microcosm and mixed to get a combined water sample. Water samples and boric acid absorption solutions were collected every 24 h during a 9-day period. The water samples were filtered through 0.45 µm membrane filter and the concentrations of ,
, and
in the filtrates were determined. The TN concentrations in the water samples at the beginning and the end of the experiment were also measured. The boric acid solutions were mixed together at the end of the experiment to determine the gross amounts of NH3 adsorbed. Gas samples were collected in gas sample bags from the sampling outtake () every four hours to determine N2O flux.
Aqueous nitrate concentration was measured by the difference of UV absorbance at 220 and 275 nm and nitrite concentration was determined colorimetrically with N-(1-naphthyl)-1,2-diaminoethane dihydrochloride (Wei and Qi Citation2002). Ammonium concentration was determined with colorimetry based on the indophenol blue method. Water samples were digested in an autoclave for 30 min at 121°C with the addition of potassium persulfate to determine TN (under alkaline conditions), total phosphorus (TP), and total dissolved phosphorus (TDP). Then TN concentration was quantified with a UV spectrophotometer, while TP and TDP were measured with the molybdenum blue colorimetric method (Wei and Qi Citation2002).
N2O concentration was determined with a gas chromatograph (Hewlett-Packard 5890 series II, Palo Alto, California, USA) equipped with electron capture detector and a stainless steel Poropak Q column. The injection volume was 1 mL. Ultrapure N2 (99.99%) was used as the carrier gas with the flow rate of 15 mL min−1. The column and detector were operated at 40°C and 200°C, respectively.
The ammonium concentration in the collected lake water samples were adsorbed by cation exchange resin and eluted by 2 M hydrochloric acid. After alkalization, the eluents and the water samples collected at the end of experiment were distilled following the method developed by Kjeldahl (Wei and Qi Citation2002). After the detection of -N concentrations, these distillates were condensed in a water bath. 15N isotopic abundances in these condensed samples and freeze-dried cyanobacteria were determined with a Delta S isotope ratio mass spectrometer (Thermo Electron, Marietta, Ohio, USA).
Because the cyanobacterial samples were stored cold until they were used, the cyanobacteria were less active when experiments began. Thus, the assimilation of nitrogen can be ignored and the following mass-balance equations for ammonium in microcosms could be constructed:
Results
The physical and chemical properties of water collected from Meiliang Bay of Lake Taihu are presented in . The total nitrogen and total phosphorus were 0.17 and 0.006 mmol L−1, respectively, suggesting eutrophic conditions in Lake Taihu. Microscopic examination showed that cyanobacteria were the dominant species in algal samples. Other species, such as green algae or diatoms, only accounted for a small portion ().
Table 1. The physical and chemical characteristics of water collected from Lake Taihu.
Table 2. The composition of algae (based on cell numbers) used in experiments.
In the in situ experiment, the DO concentration in tanks decreased abruptly from the first day and reached a minimum value of 0.96 mg L−1 on the third day. The nitrate concentration decreased continuously during the 6-day period and a corresponding increase in nitrite concentration was observed (). The DO concentration in the cyanobacteria accumulation area near tanks decreased from 22:00 h (2.5 mg L−1) and reached a minimum at 06:00 the next morning (0.52 mg L−1; ). However, DO increased during 00:00 to 04:00 h because of the wave disturbance by wind. The variation of pH in the Bay with algae cover was similar to that of DO.
Figure 2. Temporal variations of nitrate, nitrite and dissolved oxygen concentrationsin submerged tanks with 4 × 109 cells L−1 cyanobacteria added and control tanks in the field experiment. Data are means ± SD (n = 3).
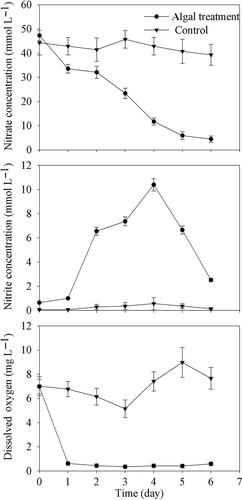
Figure 3. The variations of DO and pH in the water column of Meiliang Bay from 22:00 h to 12:00 on 3 days. Data are the means ± SD (n = 3).
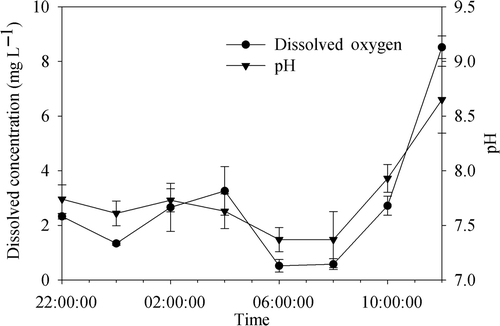
To determine whether or not cyanobacterial blooms affect nitrogen removal, nitrate transformation in the presence of cyanobacteria was traced in laboratory microcosms. It was found that the water quality was greatly affected by the cyanobacteria added in the microcosms. After a dramatic decline in the first day, pH values increased gradually in both cyanobacteria-added microcosms with their final pH similar to the initial values (). On the other hand, dissolved oxygen contents decreased abruptly and reached a minimum of 0.21 and 0.003 mg L−1 after 60 h in microcosms in the 2 × 109 and 4 × 109 cells L−1 cyanobacteria treatments, respectively ().
Figure 4. Temporal variation of pH (a) and dissolved oxygen (b) in laboratory microcosms with different cyanobacterial densities. Data are means ± SD (n = 3).
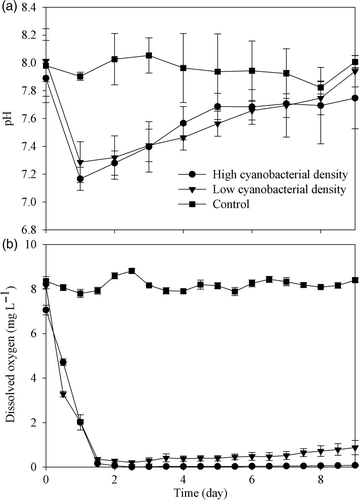
Approximately 19.7% and 37.4% of TN were removed by the end of the microcosm experiment in the low and high cyanobacterial treatments, respectively (). However, the nitrate removal was very efficient. Approximately 81.2% and 98.4% of the initial nitrate was removed in microcosms with the lower and higher cyanobacterial densities, respectively. The final nitrite concentration in microcosms with the lower cyanobacterial density was 0.88 mmol L−1, which was far higher than that in microcosms with the higher cyanobacterial density (0.015 mmol L−1). Although some amount of NH3 escaped from microcosms when cyanobacteria were added, it only amounted to a fraction of the final concentration in the water body. In addition, the isotope analysis results showed that the 15N-
abundance increased in both types of microcosms with cyanobacteria added at the end of the experiment.
Table 3. The initial and final concentrations of different nitrogen compounds and 15N isotopic abundances obtained from a laboratory incubation of spiked lake water for 9 days. The two treatments were addition of 2 × 109 cells L−1 and 4 × 109 cells L−1 of cyanobacteria.
Nitrate concentration decreased steadily to zero in microcosms with the high cyanobacterial density after 48 h. However, nitrate concentrations in microcosms with the lower cyanobacterial density decreased more slowly during the first 48 h (). With the decrease of nitrate concentration, nitrite concentration in the high cyanobacteria addition treatment increased quickly to its maximum (1.36 mmol L−1) in the first day and decreased thereafter to zero on the 6th day. However, the nitrite concentration in the treatment with low cyanobacterial density varied within a narrow range after reaching a relatively high concentration of 0.92 mmol L−1 on the 2nd day ().
Figure 5. Temporal variation of concentrations of (a),
(b),
(c), and N2O emission rates (d) in microcosms with different cyanobacterial densities. Data are means ± SD (n = 3).
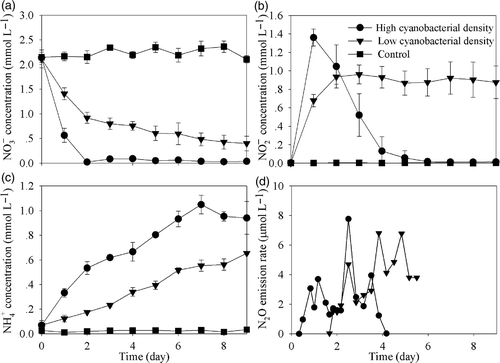
The ammonium concentration in the high cyanobacterial treatment continuously increased until reaching a maximum on the seventh day and then decreased slightly. However, in the low cyanobacteria treatment the ammonium concentration continuously increased until the ninth day (.
Aqueous nitrogen compounds can be transformed to gaseous nitrogen compounds in aquatic ecosystems. N2O flux from microcosms was not observed until 8 and 44 h after initiation of the experiment in high and low cyanobacterial densities treatments, respectively. However, no more N2O emission was observed in the microcosms with the high cyanobacterial density 100 h later. After integration of the N2O emission rates over time, the gross amounts of N2O emission were approximately 0.039 and 0.055 mmol L−1 for the high and low treatments, respectively ().
Discussion
The in situ experiment revealed that nitrate transformation occurred when a cyanobacterial bloom was occurring in Lake Taihu. Thus, the laboratory microcosms experiment was performed to explore the underlying mechanism of nitrate transformation in lake water. About 0.83 and 2.03 mmol L−1 of total nitrogen were removed from microcosms with the low and the high cyanobacterial densities, respectively, which indicated some gaseous nitrogen had escaped from microcosms. Since NH3 efflux was less than 0.03 mmol L−1 (), most of the gaseous nitrogen compounds removed from the microcosms must have been NO, N2O, or N2, suggesting the presence of denitrification in microcosms with added cyanobacteria. The dramatic variations in nitrate and nitrite concentrations also indicated that denitrification occurred. Denitrification can raise the alkalinity of the experimental media (van Rijn et al. Citation2006) that explains why pH kept rising from the second day until the nitrite was nearly exhausted on the fifth day in the high cyanobacteria treatment or until the end of the experiment in the low cyanobacteria treatment. At the end of the experiment, 15N abundance of aqueous ammonium was higher in the two cyanobacterial treatments than in the collected water sample or in cyanobacteria cells, which indicated that DNRA may also be involved in the nitrate transformation.
Nitrate can be reduced to nitrite, nitric oxide, nitrous oxide, and nitrogen gas through conventional denitrification. Thus, nitrate transformation by conventional denitrification in microcosms was the sum of nitrite increment and the flux of NO, N2O, and N2. Since the flux of NO, N2O, and N2 was the difference of total nitrogen removal and the flux of NH3, it can be calculated that about 1.69 and 2.01 mmol L−1, respectively, of initial nitrate were reduced through conventional denitrification in the low and high treatments.
Consequently, the amounts of nitrate removed by DNRA and assimilation were calculated to be 31 and 94 µmol L−1, respectively, which was in accordance with the supposition of added cyanobacteria being relatively inactive. In addition, most of the nitrogen compounds assimilated by cyanobacteria are reported to be 14N- (Dokulil and Teubner Citation2000). Therefore, AM in Equation (Equation2) can be considered as the 15N abundance in the original cyanobacteria cells. Although cyanobacteria prefer 14N to 15N under the nitrogen-enriched condition, the nitrogen isotope abundance will be close to the original value of nitrogen resource when nitrogen is exhausted (Waser et al. Citation1998). Thus, when most of the nitrate was reduced, the 15N abundance in ammonium produced through DNRA (A
D) approached that of the nitrate added. The value of A
E, which ranged between the initial and the final abundance of 15N-
in water solution, was difficult to determine. Fortunately, C
E was too small to influence the calculation of results (). AE was thus set to be the average of the initial and final abundance of aqueous 15N-
.
When the values of the parameters obtained in our experiment above were applied to Equation (Equation1) and Equation (Equation2), the -N concentrations produced by DNRA and mineralization could be calculated. In the low and high treatments, ammonium concentrations transformed from nitrate by DNRA were 0.01 mmol L−1 and 0.03 mmol L−1, respectively, while ammonium concentrations mineralized from cyanobacteria were 0.63 mmol L−1 and 0.91 mmol L−1, respectively (). Thus, the increasing ammonium in microcosms mainly came from the cyanobacterial decomposition.
Table 4. The variations of ammonium concentration in microcosms with different cyanobacterial densities obtained from a laboratory incubation of spiked lake water for 9 days.
The contribution of each nitrogen transformation process above to nitrate removal was calculated (). The conventional denitrification pathway from nitrate to nitrogen gas was the dominant process removing approximately 80.1–94.0% of nitrate in microcosms with cyanobacteria added. The amounts of nitrate assimilated by cyanobacteria were less than 0.07 mmol L−1, which indicated that assuming A M to be equal to the original 15N abundance in cyanobacterial cells was suitable. Veraart et al. (Citation2011) investigated the effect of floating vegetation (Lemna spp.) on denitrification rates and found that the nitrate removal in the microcosm was dominated by plant uptake rather than denitrification, which was quite the opposite of this study. This discrepancy may be accounted for by differences in the nitrate uptake rates of cyanobacteria and Lemna spp. As mentioned earlier cyanobacteria prefer to absorb ammonia, while Lemna spp. prefer to assimilate nitrate (Cedergreen and Madsen Citation2002). In addition, the DO concentration in microcosms in this study was far lower than that in the microcosms with duckweed. Thus, the DO may be another factor causing the conflicting results.
Table 5. The aqueous nitrate transformation (NT) pathways in microcosms with different cyanobacterial densities.
DNRA is an important nitrogen transformation process that might account for over 75% of total nitrate reduction in some lakes (Pelegrí and Blackburn Citation1996). However, less than 1.4% of the initial nitrate was reduced to ammonium through dissimilatory pathways in this study. Relatively high reduced sulfur concentrations (including H2S, , and S) might favor DNRA in aquatic sediments (Burgin and Hamilton Citation2007). However, the gross amounts of N2O emission were less than 0.33 mmol (about 3.2% of the total nitrate reduction), which indicates that the microcosms were short of reduced sulfur (Senga et al. Citation2006). Therefore, the absence of reduced sulfur may explain the reason why DNRA made a relatively small contribution to the total
reduction in microcosms with cyanobacteria added.
Previous research in our laboratory illustrated that the bacteria that are suspended or attached to algae rapidly proliferated when a cyanobacterial bloom declined. Most of the aforementioned bacteria were denitrifiers, such as Pseudomonas spp. and Bacillus spp. (Zheng et al. Citation2008). The proliferation of denitrifiers indicated that the environment created by declining cyanobacterial populations was suitable for denitrification. In this study, the heterotrophic bacterial activity reduced dissolved oxygen levels in cyanobacterial microcosms and the algal mucus also acted as a potential barrier to re-aeration that made denitrification possible. In addition, the declining cyanobacteria were readily degradable and thus served as an additional carbon source for denitrifiers (Arango et al. Citation2007).
The denitrification rate was influenced by the environmental conditions greatly. About 0.88 mmol L−1 nitrite remained in the microcosm with 2 × 109 cells L−1 cyanobacteria until the end of experiment. The incomplete denitrification in this treatment might be due to the absence of organic carbon from decomposition of cyanobacterial bloom (Kelso et al. Citation1999).
Based on the stoichiometry of denitrification, the theoretical ratio of chemical oxygen demand (COD) to nitrate for complete denitrification is 7.6 g O2 per gram N (Sobieszuk and Szewczyk, Citation2006). The chemical formula CH1.4O0.4N0.2 is frequently used to indicate the elemental composition of cyanobacteria. Analysis of cyanobacterial cells showed that the dry weight of a cell was about 1.5 × 10−7 mg. Thus, according to the chemical formula, CODs were 0.49 and 0.98 g O2 L−1 in microcosms with 2 × 109 and 4 × 109 cells L−1, respectively. Therefore, it seems that the organic carbon created by cyanobacterial decomposition was enough for complete denitrification. However, decomposition is not instantaneous, so there could be a temporary shortage of organic carbon during denitrification in microcosms with the lower cyanobacterial density. On the other hand, the lower cyanobacterial density could have caused less oxygen consumption and earlier reaeration (). Therefore, the relatively high dissolved oxygen concentration (over 0.88 mg L−1) appeared in microcosms with the low cyanobacterial density; thus, this environmental condition may also cause incomplete denitrification. Substituting the initial and final concentrations of nitrate and nitrite in microcosms with different cyanobacterial densities to Equation (Equation3), it could be deduced that the organic carbon from the degradation of 109 cells cyanobacteria could support denitrifiers to reduce the 0.53 ± 0.01 mmol (n = 6) nitrate completely. This value would become even larger if the influence of dissolved oxygen induced by cyanobacterial bloom was considered.
The water-sediment interface has been recognized to be the main denitrification location in lake ecosystems. It has been proposed that nitrate in the upper water layer diffuses down to the sediments and gets denitrified at the water-sediment interface (Andersen et al. Citation1984). However, we found that water body itself could be another important niche for denitrification during cyanobacterial blooms. The minimum dissolved oxygen concentration in the cyanobacterial accumulation area was 0.65 mg L−1 (). Such low DO would enhance denitrification rates in the water column (Pina-Ochoa and Álvarez-Cobelas Citation2006). Therefore, the nitrate could be removed in the water column directly instead of diffusing into the sediments (Thouvenot et al. Citation2007), which would greatly accelerate nitrogen removal from the lake system.
In our laboratory experiment, the denitrification process followed first-order kinetics with 0.23 and 1.41 d−1 being the rate constant in the low and high cyanobacterial treatments, respectively (r 2 ranged from 0.91 to 0.99; ). According to the nitrate concentration in the original water collected from Lake Taihu (), the denitrification rates were estimated to be 9.2 and 60.0 µmol L−1 d−1 in water with 2 × 109 and 4 × 109 cells L−1 cyanobacteria, respectively, which were higher than that in the sediments of Lake Taihu (McCarthy et al. Citation2007).
Several novel nitrogen removal processes recently have been developed to enhance the efficiency of nitrification and denitrification in wastewater treatment systems (Zhu et al. Citation2008). Compared with these processes, the total nitrogen removal efficiency obtained in this experiment (less than 37.44%) was relatively lower due to the great organic nitrogen input from cyanobacteria. However, the nitrate removal efficiency was about 98.6% in the high cyanobacterial treatments, which is more efficient than that in many bioreactors of wastewater treatment facilities (Koenig and Liu 2001; Aslan and Turkman 2006).
The cyanobacteria density in some lake regions, such as in Meiliang Bay, was sometimes far higher than that in microcosms in this experiment. Higher cyanobacterial density means more oxygen would be consumed and more organic carbon could be supplied for denitrification (Fallon and Brock Citation1979). In this study, the nitrate was sufficient for denitrification. However, if nitrate availability is limited, the nitrogen removal rates may be largely dependent on the nitrate concentration produced by nitrification. Due to the photosynthesis of cyanobacteria, the DO in surface water at 12:00 was about 8.6 mg L−1 at the cyanobacterial accumulation area (). Such a high concentration of DO could enhance nitrification (Eriksson and Weisner Citation1999). Thus, there may exist a cycle of diurnal nitrification and nightly denitrification when cyanobacterial blooms occur. Therefore, the high cyanobacterial density that arose with the increasing inputs of nutrients may promote nitrogen removal capability. This could eventually result in N limitation of the primary production in the lake ecosystem and, consequently, cyanobacterial blooms would be alleviated that just reflects the ecological homeostasis of the lake ecosystem.
In conclusion, our field investigation and laboratory experimental results demonstrated that cyanobacterial blooms can form anaerobic conditions and serve as an organic carbon source, causing the water column to be another key site for denitrification in addition to sediment, thereby promoting denitrification and the removal of nitrogen efficiently and quickly. Conventional denitrification was found to play a major role in the nitrate removal process. It could be deduced that 109 cells of cyanobacteria were enough for denitrifiers to remove 0.53 mmol L−1 nitrate in eutrophic lake ecosystems. Thus, denitrification may be an important driving force for decreasing the nitrogen content during the period of the cyanobacterial bloom in Lake Taihu. This interactive relationship of nitrogen and cyanobacteria may constitute a negative feedback system of alleviating cyanobacterial bloom which is facilitated by high nitrogen inputs to the eutrophic lake environment.
Acknowledgments
This work is supported by grants from Water Special Program (No. 2009ZX07106-001-02) and National Basic Research Program of China (973 Program) (No. 2008CB418102).
References
- Andersen , TK , Jensen , MH and Sørensen , J . 1984 . Diurnal variation of nitrogen cycling in coastal, marine sediments: I . Denitrification. Marine Biology , 83 : 171 – 176 .
- Arango , C , Tank , JL , Schaller , JL , Royer , T , Bernot , MJ and David , MB . 2007 . Benthic organic carbon influences denitrification in streams with high nitrate concentration . Freshwater Biology , 52 : 1210 – 1222 .
- Aslan S, Turkman A. 2006. Nitrate and pesticides removal from contaminated water using biodenitrification reactor. Process Biochemistry. 41:882–886
- Burgin , AJ and Hamilton , SK . 2007 . Have we overemphasized denitrification in aquatic ecosystems: a review of nitrate removal pathways . Frontiers in Ecology and the Environment , 5 : 89 – 96 .
- Cedergreen , N and Madsen , TV . 2002 . Nitrogen uptake by the floating macrophyte Lemna minor . New Phytologist , 155 : 285 – 292 .
- Dalsgaard , T , Thamdrup , B and Canfield , DE . 2005 . Anaerobic ammonium oxidation (anammox) in the marine environment . Research in Microbiology , 156 : 457 – 464 .
- Dokulil , MT and Teubner , K . 2000 . Cyanobacterial dominance in lakes . Hydrobiologia , 438 : 1 – 12 .
- Donaghay , PL and Osborn , TR . 1997 . Toward a theory of biological-physical control of harmful algal bloom dynamics and impacts . Limnology and Oceanography , 42 : 1283 – 1296 .
- Duce , RA , LaRoche , J , Altieri , K , Arrigo , KR , Baker , AR , Capone , DG , Cornell , S , Dentener , F , Galloway , J Ganeshram , RS . 2008 . Impacts of atmospheric anthropogenic nitrogen on the open ocean . Science , 320 : 893 – 897 .
- Eriksson , PG and Weisner , SEB . 1999 . An experimental study on effects of submersed macrophytes on nitrification and denitrification in ammonium-rich aquatic systems . Limnology and Oceanography , 44 : 1993 – 1999 .
- Fallon , RD and Brock , TD . 1979 . Decomposition of blue-green algal (cyanobacterial) blooms in Lake Mendota, Wisconsin . Applied and Environmental Microbiology , 37 ( 5 ) : 820 – 830 .
- Galloway , JN , Townsend , AR , Erisman , JW , Bekunda , M , Cai , Z , Freney , JR , Martinelli , LA , Seitzinger , SP and Sutton , MA . 2008 . Transformation of the nitrogen cycle: recent trends, questions, and potential solutions . Science , 320 : 889 – 892 .
- Golterman , HL . 2004 . The chemistry of phosphate and nitrogen compounds in sediments , Dordrecht : Kluwer Academic Publishers .
- Hallegraeff , GM . 1993 . A review of harmful algal blooms and their apparent global increase . Phycologia , 32 ( 2 ) : 79 – 99 .
- Halm , H , Musat , N , Lam , P , Langlois , R , Musat , F , Peduzzi , S , Lavik , G , Schubert , CJ , Singha , B LaRoche , J . 2009 . Co-occurrence of denitrification and nitrogen fixation in a meromictic lake, Lake Cadagno (Switzerland) . Environmental Microbiology , 11 ( 8 ) : 1945 – 1958 .
- Harrison , JA , Maranger , RJ , Alexander , RB , Harrison , JA , Maranger , RJ , Alexander , RB , Giblin , AE , Jacinthe , P , Mayorga , E Seitzinger , SP . 2009 . The regional and global significance of nitrogen removal in lakes and reservoirs . Biogeochemistry , 93 : 143 – 157 .
- Hu , HJ , Li , YY and Wei , YX . 1980 . The freshwater algae of China , Shanghai : Shanghai Science and Technology Press .
- Ishida , CK , Arnon , S , Peterson , CG , Kelly , JJ and Gray , KA . 2008 . Influence of algal community structure on denitrification rates in periphyton cultivated on artificial substrata . Microbial Ecology , 56 : 140 – 152 .
- Kelso , BHL , Smith , RV and Laughlin , RJ . 1999 . Effects of carbon substrates on nitrite accumulation in freshwater sediments . Applied and Environmental Microbiology , 65 ( 1 ) : 61 – 66 .
- Koenig A, Liu LH. 2001. Kinetic model of autotrophic denitrification in sulphur packed-bed reactors. Water Research. 35:1969–1978
- Li , K . 1999 . Management and restoration of fish communities in Lake Taihu, China . Fisheries Management and Ecology , 6 : 71 – 81 .
- McCarthy , MJ , Lavrentyev , PL , Yang , L , Zhang , L , Chen , Y , Qin , B and Gardner , WS . 2007 . Nitrogen dynamics and microbial food web structure during a summer cyanobacterial bloom in a subtropical, shallow, well-mixed, eutrophic lake (Taihu Lake, China) . Hydrobiologia , 581 : 195 – 207 .
- McMillan SK, Piehler MF, Thompson SP, Paerl HW. 2010. Denitrification of Nitrogen Released from Senescing Algal Biomass in Coastal Agricultural Headwater Streams. Journal of Environmental Quality. 39:274–281
- Nielsen , LP . 1992 . Denitrification in sediments determined from nitrogen isotope pairing . FEMS Microbiology Ecology , 86 : 357 – 362 .
- Pan , G , Zou , H , Chen , H and Yuan , X . 2006 . Removal of harmful cyanobacterial blooms in Taihu Lake using local soils: III. Factors affecting the removal efficiency and an in situ field experiment using chitosan-modified local soils . Environmental Pollution , 141 : 206 – 212 .
- Pelegrí , SP and Blackburn , TH . 1996 . Nitrogen cycling in lake sediments bioturbated by Chironomusplumosus larvae, under different degrees of oxygenation . Hydrobiologia , 325 : 231 – 238 .
- Pina-Ochoa , E and Álvarez-Cobelas , M . 2006 . Denitrification in aquatic environments: a cross-system analysis . Biogeochemistry , 81 : 111 – 130 .
- Robinson , D . 2001 . 15N as an integrator of the nitrogen cycle . Trends in Ecology and Evolution , 16 ( 3 ) : 153 – 162 .
- Senga , Y , Mochida , K , Fukumori , R , Okamoto , N and Seike , Y . 2006 . N2O accumulation in estuarine and coastal sediments: the influence of H2S on dissimilatory nitrate reduction . Estuarine, Coastal and Shelf Science , 67 : 231 – 238 .
- Sirivedhin , T and Gray , KA . 2006 . Factors affecting denitrification rates in experimental wetlands: Field and laboratory studies . Ecological Engineering , 26 : 167 – 181 .
- Smayda TJ. 1997. Harmful algal blooms: Their ecophysiology and general relevance to phytoplankton bloom in the sea. Limnology and Oceanography. 42:1137–1153
- Sobieszuk , P and Szewczyk , KW . 2006 . Estimation of (C/N) ratio for microbial denitrification . Environmental Technology , 27 : 103 – 108 .
- Thouvenot , M , Billen , G and Garnier , J . 2007 . Modelling nutrient exchange at the sediment-water interface of river systems . Journal of Hydrology , 341 : 55 – 78 .
- Thouvenot , M , Billen , G and Garnier , J . 2009 . Modelling benthic denitrification processes over a whole drainage network . Journal of Hydrology , 379 : 239 – 250 .
- Valiela , I , McClelland , J , Hauxwell , J , Behr , PJ , Hersh , D and Foreman , K . 1997 . Macroalgal blooms in shallow estuaries: Controls and ecophysiological and ecosystem consequences . Limnology and Oceanography , 42 : 1105 – 1108 .
- van , R J , Tal , Y and Schreier , HJ . 2006 . Denitrification in recirculating systems: theory and applications . Aquacultural Engineering , 34 : 364 – 376 .
- Veraart , AJ , de Bruijne , WJJ , de Klein , JJM , Peeters , ETHM and Scheffer , M . 2011 . Effects of aquatic vegetation type on denitrification . Biogeochemistry , 104 : 267 – 274 .
- Vrede , T , Ballantyne , A , Mille-Lindblom , C , Algesten , G , Gudasz , C , Lindahl , S and Brunberg , AK . 2009 . Effects of N: P loading ratios on phytoplankton community composition, primary production and N fixation in a eutrophic lake . Freshwater Biology , 54 : 331 – 344 .
- Wang , H , Wang , W , Yin , C , Wang , Y and Lu , J . 2006 . Littoral zones as the “hotspots” of nitrous oxide (N2O) emission in a hyper-eutrophic lake in China . Atmospheric Environment , 40 : 5522 – 5527 .
- Waser , NAD , Harrison , PJ , Nielsen , B and Calvert , SE . 1998 . Nitrogen isotope fractionation during the uptake and assimilation of nitrate, nitrite, ammonium, and urea by a marine diatom . Limnology and Oceanography , 43 ( 2 ) : 215 – 224 .
- Wei , FS and Qi , WQ . 2002 . Monitoring analysis methods for water and wastewater , 4th , Beijing : China Environmental Science Press .
- Xie , L , Xie , P , Li , S , Tang , H and Liu , H . 2003 . The low TN: TP ratio, a cause or a result of Microcystis blooms? . Water Research , 37 : 2073 – 2080 .
- Xu , H , Paerl , HW , Qin , B , Zhu , G and Gao , G . 2010 . Nitrogen and phosphorus inputs control phytoplankton growth in eutrophic Lake Taihu, China . Limnology and Oceanography , 55 ( 1 ) : 420 – 432 .
- Ye , L , Wu , X , Tan , X , Shi , X , Li , D , Yu , Y , Zhang , M and Kong , F . 2010 . Cell lysis of cyanobacteria and its implications for nutrient dynamics . International Review of Hydrobiology , 95 ( 3 ) : 235 – 245 .
- Zheng , X , Xiao , L , Ren , J and Yang , L . 2008 . The effect of a Microcystisaeruginosa bloom on the bacterioplankton community composition of Lake Xuanwa . Journal of Freshwater Ecology , 23 ( 2 ) : 297 – 304 .
- Zhu , G , Peng , Y , Li , B , Guo , J , Yang , Q and Wang , S . 2008 . Biological removal of nitrogen from wastewater . Reviews of Environmental Contamination and Toxicology , 192 : 159 – 195 .