Abstract
Ammonium (NH4+) is an important nitrogen nutrition for plant growth; however, it is toxic to many plants at high concentration. Eutrophication induces NH4+enrichment in shallow lakes and increases risk of NH4+ toxicity to submersed macrophytes. Potassium (K+) was known as an alleviant to the toxic syndromes of plant under NH4+ stress. In the present study, we examined the single effects and co-effects of NH4+ and K+ enrichments (∼0.65 mg L−1 NH4–N, ∼45 mg L−1 K+) in water column on submersed macrophyte Vallisneria natans L. with focus on carbon (C) and nitrogen (N) metabolism, life history and asexual reproduction of the plant in outdoor aquariums lasting for about five months. We found that the NH4+ enrichment alone increased growth and decreased death rate of the plant, leading to higher biomass, longer survivorship and more tuber production and ramets of the plants, indicating that the NH4+ enrichment was not stressful to the plant. The combination of NH4+ and K+ enrichments enhanced the plant growth to less extent as did the NH4+ enrichment alone, and reduced the plant death rate. The NH4+ enrichment increased the concentrations of free amino acids and nitrogen, and decreased the concentrations of soluble carbohydrate in leaves and tubers of the plant irrespective of the K+ enrichments. The K+ enrichments alone did not affect growth, death rates and C–N metabolism of the plant. The K+ alone or together with NH4+ enrichments had negligible effect on tuber production and tuber C–N metabolism of the plant. The present results indicated that the K+ enrichment had negligible effects on the plant; the NH4+ enrichment (0.65 mg L−1 NH4–N) benefited the plant growth, while it might negatively affect the asexual reproduction due to low carbohydrate contents of the tubers particularly under low light stress in eutrophic lake.
Introduction
Ammonium (NH4+) is an important nitrogen nutrition for plant. Moderate NH4+ availability benefits plant growth; however, high NH4+ concentration is toxic to many plants (Britto & Kronzucker Citation2002; Cao et al. Citation2007). In shallow eutrophic lakes, high NH4+ concentration in water column is not uncommon due to overloading of sewage, agricultural runoff and depletion of oxygen in the lake, and is considered as one main cause for the succession and/or decline of submersed vegetation (Farnsworth-Lee & Baker Citation2000; Smolders et al. Citation2006; Cao et al. Citation2007; Zhang et al. Citation2011; Su et al. Citation2012).
Submersed macrophytes can take up NH4+ and NO3− by roots and shoots and prefer NH4+ over NO3− when both are equivalently available (Nichols & Keeney Citation1976; Short & McRoy Citation1984; Barko et al. Citation1991). In experimental studies, NH4+ supplement in water column induced the accumulation of nitrogen and free amino acids (FAA) and depletion of soluble carbohydrates (SC) in the tissues of submersed macrophytes (Best Citation1980; Smolders et al. Citation1996; Kohl et al. Citation1998; Cao et al. Citation2009), and stimulated plant growth in oligotrophic waters but inhibited plant growth in eutrophic waters (Cao et al. Citation2007). In mesocosm experiments, NH4+ supplement in water column led to low SC contents in rhizomes of Vallisneria natans L. and seagrass Posidonia oceanica (Invers et al. Citation2004; Cao et al. Citation2007), and inhibited ramet production of V. natans possibly due to low SC contents in the rhizomes (Cao et al. Citation2007).
Mechanism of NH4+ toxicity to plants is far from clear, possible mechanisms including acidification of growth media, uncoupling of photosynthetic phosphorylation, oxidative stress, futile recycling of NH4+ across membrane and unbalance of C–N metabolism of plants (Kohl et al. Citation1998; Britto et al. Citation2001; Britto & Kronzucker Citation2002; Nimptsch & Pflugmacher Citation2007; Cao et al. Citation2011). Potassium (K+) is similar to NH4+ with respect to molecular radius, electron charge and hydration energy, implying that they share some common components in membrane transport (Tsay et al. Citation2011). Addition of K+ can alleviate NH4+ toxicity to terrestrial plants in both experimental and field studies (Barker et al. Citation1967; Lips et al. Citation1990; Feng & Barker Citation1992; Cao et al. Citation1993; Britto & Kronzucker Citation2002). In roots of rice and barley, high concentration of K+ reduced the influx and efflux of NH4+ across cytosol membrane, decreased the efflux-to-influx ratio of NH4+ and alleviated the futile NH4+ recycling across cytosol membrane (Szczerba et al. Citation2008; Balkos et al. Citation2010). Submersed macrophytes and seagrass are capable of taking up K+ through their roots and shoots (Nichols & Keeney Citation1976; Short & McRoy Citation1984; Barko et al. Citation1991; Zhong et al. Citation2013). It is not clear whether K+ supplement in cultural or cultivation solution could alleviate NH4+ toxicity to submersed macrophytes when both K+ and NH4+ are absorbed by the shoots of macrophytes, in contrast to the uptaking of K+ and NH4+ only by the roots in terrestrial plants.
In a field survey of 31 lakes along the lower reaches of the Yangtze River, Cao et al. (Citation2007) found that the submersed macrophyte V. natans preferring mesotrophic over eutrophic lakes. V. natans is a common submersed rosette macrophyte in lakes along the lower reaches of the Yangtze River, and declines in most of these lakes due to serious eutrophication (Zhang et al. Citation1999). V. natans produces tubers in early winter when its aboveground part is dying, and seedlings will germinate from both seeds and tubers in the coming spring, but seedlings germinating from tubers are stronger and more effective in clonal growth than those from seeds (Xiong & Li Citation2000). In eutrophic lakes, reproduction of V. natans depends mainly on overwintering tubers (Xiong & Li Citation2000; Chen et al. Citation2006). In experimental studies, NH4+ enrichment in water column disturbed C–N metabolism of V. natans, but affected its growth either positively or negatively as dependent on NH4+ concentration and light availability (Cao et al. Citation2007, Citation2009, Citation2011). Cao et al. (Citation2007) suggested 0.56 mg L−1 NH4–N in water column as an upper threshold for growth of V. natans in eutrophic lakes. As tuber production is a key linkage between individual growth and reproduction of V. natans, examining effects of NH4+ enrichment on quantity and quality of the tuber would help to understand population dynamics of this species in eutrophic lakes.
In the present study, we examined the single effects and co-effect of NH4+ and K+ enrichment in water column on V. natans in an aquaria experiment lasting for about five months, which covered the key stages in the plant life history (i.e. tuber production and death of the aboveground part), with focus on C–N metabolism, growth and tuber production of the plant; and tested if high K+ concentrations in water column could alleviate stressful effects of NH4+ enrichment on the plant.
Materials and methods
The experiments were conducted in Donghu Experimental Station of Lake Ecosystem (30°32′53.41″N, 114°21′15.63″E) from 19 July to 4 December in 2011. Seedlings of V. natans were collected from the mesotrophic Tanglinhu area of Lake Donghu. The seedlings selected for the experimental treatments were similar in size and healthy in appearance, with an average dry weight of 0.37 g per individual plant. Sediment for culturing the plant was collected from deep bottom near to the stands of V. natans in the lake and then mixed thoroughly in a big tank.
Experimental design and procedures
The experiment was a full factorial design with NH4+ and K+ enrichments in water column as two factors, including four treatments: tap water being the control (indicated by C) and supplement with (NH4)2SO4 (NH4+ treatment, A for short), and supplement with K2SO4 (K+ treatment, K for short), and both (AK for short), respectively. Each treatment had five replicates.
One hundred and twenty of the seedlings were uniformly transplanted into 20 aquaria (50 × 50 × 80 cm) that contained 18-cm sediment in the bottom, with six seedlings in each aquarium, and then the aquaria were filled with tap water to 60-cm depth. The aquaria were placed outdoor under a black net roof, which shade about 80% of sun light, and the plants were kept for acclimation in tap water for 10 days before the supplements of NH4+ and K+ were added. Concentrations of PO4–P, NH4–N, NO3–N and K+ in water column of each aquarium were measured in every three days; stocking solutions of (NH4)2SO4 and K2SO4 were added to the water column to achieve appropriate concentrations of NH4–N and K+ in every 3 and 15 days, respectively. During the experiment, water in each aquarium was renewed nine times in order to minimize algal growth in the water column.
Measurements of environmental indices
Photosynthetically active radiation (PAR) was measured just below water surface in each aquarium at noon in every three days using an underwater irradiance sensor (UWQ-7893) connected to a data logger (Li-1400, Li-Cor Company, Lincoln, NE, USA). Water samples from each aquarium were filtered through GF/C glass fiber membrane (1.2 μm in pore diameter; Whatman company, Middlesex, UK), and used for the analysis of PO4–P, NH4–N, NO3–N and K+ concentrations. NH4–N was analyzed by the Nessler method and NO3–N by the UV spectrophotometric method (Eaton et al. Citation1995). PO4–P was measured following the method described by Golterman (Golterman & Clymo Citation1969). K+ was measured by a Dionex DX-100 ion chromatograph (DIONEX, Sunnyvale, CA, USA) equipped with an IonPac CS12A analytical column (250 × 4 mm). At the beginning of the experiment, five replicates of 50-mL sediment from the mixed sediment were centrifuged at 5000g, and the supernatants were collected for examining the concentrations of PO4–P, NH4–N, NO3–N and K+ following the methods described as above.
Measurements of C–N metabolites, growth and tuber production of V. natans
In each aquarium, ramet numbers (NR) of V. natans were counted and 3–5 mature leaves from the 3–5 robust plants were randomly collected every 15 days. The leaves from each aquarium were separately oven dried at 80 °C to a constant weight for further biochemical analysis. At the end of the experiment, all tubers of V. natans buried in the sediment were collected in each aquarium, clearly washed by distilled water, counted and weighted. Ten tubers randomly chosen from each aquarium were oven dried at 80 °C to a constant weight for further biochemical analysis.
The oven-dried leaves and tubers were ground into fine powders separately. About 50 mg of the powder was extracted twice with 5 mL 80% ethanol at 80 °C for 20 min, the extracts were pooled together and centrifuged at 10,000g for 15 min, and the supernatant was used to examine FAA and SC concentrations using alanine and glucose as standards, respectively (Yemm & Willis Citation1954; Yemm et al. Citation1955). The residue was used to examine starch content following the method of Dirk et al. (Citation1999). Concentrations of C and N were measured by an elemental analyzer (Flash EA 1112, CE Instruments, Italy).
As biomass of V. natans is linearly correlated to its ramet numbers (unpublished data of Cao et al.), relative growth rate (RGR) of V. natans was calculated from the changes in NR of the plant in each aquarium using the following formula:
where NR1 and NR2 are the ramet numbers counted at the date of T1 and T2, respectively. Negative value of RGR means death of the plants, and hereafter is expressed as death rate of the plant.
Data analysis
Analysis of data was conducted with R software running in Win 7 [R Core Team (2012). R: A language and environment for statistical computing. R Foundation for Statistical Computing, Vienna, Austria. URL http://www.R-project.org/.]. Data were tested for normality and equality of variance, and when necessary, transformed by log10(x) or sqrt(x), prior to analysis. Significant differences of tuber properties between different treatments were verified through one-way analysis of variance (ANOVA) followed by a Tukey honestly significant difference (HSD)'s test. Two-way ANOVA was then conducted to examine the effects of NH4+ (A) and K+ (K) enrichments in water column and their combination on growth, tuber production and biochemical parameters of V. natans. Spearman's rank correlation was used to evaluate the relationships between biochemical parameters in tubers and those in the leaves of V. natans. Partial correlation was used to examine the relationships between maximum number of the ramets during the experiment (NM), death rate of V. natans and the total tuber biomass.
Results
Environmental parameters of the treatments
PAR was 34–296 μmol m−2 s−1 and was not significantly different among the treatments. Concentrations of PO4–P, NH4–N and NO3–N in the sediment pore water were 0.26, 1.3 and 0.16 mg L−1, respectively, at the beginning of the experiments (). Addition of NH4+ and K+ to the water column significantly increased their concentrations, with 0.04, 0.04, 0.63 and 0.66 mg L−1 NH4–N and 1.3, 45, 1.2 and 45 mg L−1 K in the C, K, A and AK treatments, respectively. NO3–N concentrations increased from 0.66 to 1.54 mg L−1 caused by supplying NH4+ to the water column. PO4–P concentration was 7.5–8.8 μg L−1 in the water column and did not differ significantly among the treatments ().
Table 1. Concentrations of PO4–P, NH4–N and NO3–N in the water column (measured every three days during the experimental period) and the sediment pore water (measured at the beginning of the experiments) of the treatments.
Clonal growth and tuber production of V. natans in response to NH4+ and K+ enrichments
According to RGR values of V. natans, its growth could be divided into three stages: rapid growth stage (RGS, 1st–41st day), maintenance stage (MS, 42nd–93rd day) and dying stage (DS, 94th–137th day) during the experimental period ().
Figure 1. Temporal changes in ramets number of V. natans grown in different treatments. C: the control with tap water only; A: NH4+ enriched tap water; K: K+ enriched tap water; and AK: NH4+ and K+ enriched tap water. Values are the mean ± SD (n = 5).
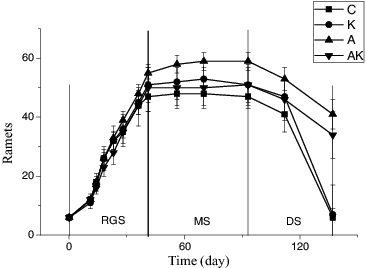
Compared with the control, the NH4+ enrichment alone (A treatment) increased clonal growth of V. natans and prolonged its survivorship period by about three weeks, resulting in 21% higher NR at the MS stage. The K+ alone (K+ treatment) or together with NH4+ enrichments (AK treatment) marginally increased the NR by 6%. The NH4+ enrichment decreased the plant death rates (negative RGR) irrespective of the K+ treatments, consequently still having 67% of the maximum NR at the end of the experiment in the treatments with NH4+ enrichment (, ).
Table 2. Percentage of explained variance in ANOVA results for growth, asexual reproduction and biochemical parameters of V. natans in response to NH4+ (A) and K+ (K) enrichments in water column of the different treatments.
The NH4+ and/or K+ enrichments affected tuber production and biomass of V. natans by means similar to those of the plant NR at the MS stage, with the tuber number and biomass ranged by A > AK and K > the control. The K+ enrichments marginally decreased individual tuber biomass of V. natans irrespective of the NH4+ treatments (, ).
Table 3. Spearman's correlation coefficients of relationships between the biochemical parameters in tubers and those in the leaves of V. natans at the MS and DS stages.
Figure 2. Total tuber number, individual tuber biomass and total tuber biomass of V. natans grown in different treatments at the end of the experiment. C: the control with tap water only; A: NH4+ enriched tap water; K: K+ enriched tap water; and AK: NH4+ and K+ enriched tap water. Values are the mean ± SD (n = 5). Different letters indicate significant differences (p < 0.05).
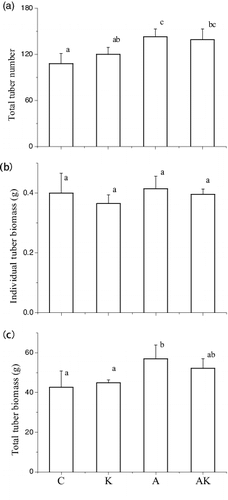
Carbon and nitrogen metabolism of V. natans in response to NH4+ and K+ enrichments
The NH4+ enrichments greatly enhanced N contents in the leaves of V. natans. The N contents of the plant leaves increased in the A and AK treatments gradually with the experimental time until the DS stage; however, in C and K treatments, it increased only slightly at the early RGS stage and then gradually decreased. The K+ enrichments did not affect the N contents irrespective of the NH4+ treatments. At the end of the experiment, the N contents were around 39.7 and 26.1 mg g−1 in the treatments with (A and AK) and without NH4+ addition (C and K), respectively (, ). Carbon contents in the leaves of V. natans were around 370 mg g−1, maintained relatively stable values and did not differ among the treatments during the RGS and MS stages. The NH4+ enrichments enhanced the C contents but the K+ enrichments reduced the C contents at the DS stage as compared to the control (, ).
Figure 3. Temporal changes in nitrogen and carbon contents in leaves of V. natans grown in different treatments. RGS: rapid growth stage; MS: maintenance stage; DS: dying stage; C: the control with tap water only; A: NH4+ enriched tap water; K: K+ enriched tap water; and AK: NH4+ and K+ enriched tap water. Values are the mean ± SD (n = 5).
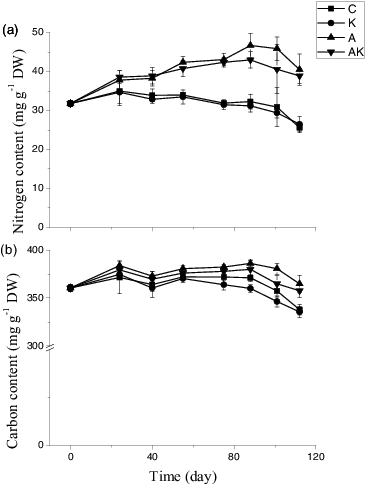
FAA contents in the leaves of V. natans increased gradually with the experimental time in the treatments with NH4+ enrichment and reached 2 mg g−1 at the end of the experiment, while maintained relatively stable values (0.65 mg g−1) during the experimental period in the treatments without NH4+ enrichment ((a), ). SC contents in the leaves of V. natans decreased gradually from 32 to 11.53 mg g−1 during the experiment in the A and AK treatments, and maintained to be stable (42.83 mg g−1) in the control and K treatments until the late DS stage when the SC contents increased subsequently ((b), ). The K+ enrichments did not affect the FAA and SC contents. Starch contents in the leaves of V. natans increased at the early RGS stage and then gradually decreased in all the treatments ((c), ).
Figure 4. Temporal changes in contents of free amino acids (FAA), soluble carbohydrate (SC) and starch in leaves of V. natans grown in different treatments. C: the control with tap water only; A: NH4+ enriched tap water; K: K+ enriched tap water; and AK: NH4+ and K+ enriched tap water. Values are the mean ± SD (n = 5).
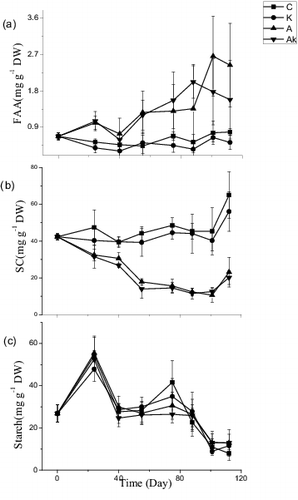
The NH4+ enrichments enhanced FAA and N contents and decreased SC and starch contents in the tubers of V. natans, with 1.80, 1.65, 2.71 and 3.04 mg g−1 FAA, 20.16, 20.01, 28.47 and 28.26 mg g−1 nitrogen, 29.43, 29.47, 22.18 and 22.94 mg g−1 SC and 675.10, 678.44, 615.28 and 612.79 mg g−1 starch in the control, K, A and AK treatments, respectively. The K+ enrichments did not affect the biochemical indices. Carbon contents of the tubers did not differ among the treatments ( and ).
Figure 5. Contents of FAA, SC of and starch in tubers of V. natans grown in different treatments at the end of the experiment. C: the control with tap water only; A: NH4+ enriched tap water; K: K+ enriched tap water; and AK: NH4+ and K+ enriched tap water. Values are the mean ± SD (n = 5). Different letters indicate significant differences (p < 0.05).
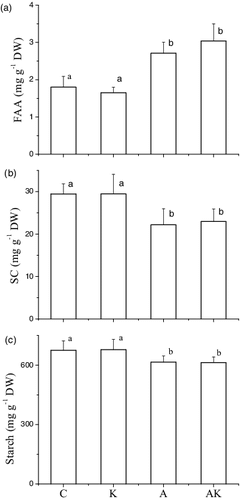
Figure 6. Contents of nitrogen and carbon in tubers of V. natans grown in different treatments at the end of the experiment. C: the control with tap water only; A: NH4+ enriched tap water; K: K+ enriched tap water; and AK: NH4+ and K+ enriched tap water. Values are the mean ± SD (n = 5). Different letters indicate significant differences (p < 0.05).
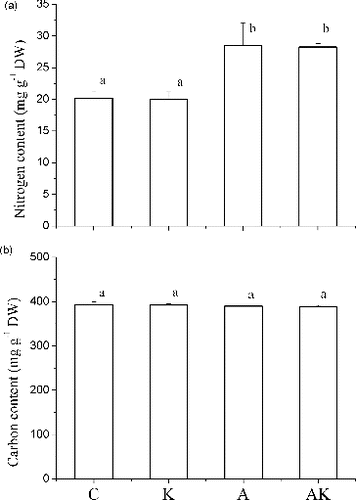
Relationships between C–N metabolites, growth and tubers production of V. natans
The FAA and N contents in the tubers of V. natans positively correlated with their contents in the leaves and negatively correlated with the SC contents in the leaves at the MS and DS stages (p < 0.01 for all). The SC and starch contents in tubers positively correlated with the SC contents and negatively correlated with the FAA and N contents in the leaves at the MS and DS stages (p < 0.05 for all). The starch contents in the leaves positively correlated with the SC contents and negatively correlated with the FAA contents in the tubers at the MS stage (p < 0.05 for all). No significant relationship was found between the biochemical indices of the tubers and the starch contents in the leaves at the DS stage ().
The total tuber biomass of V. natans significantly positively correlated with the maximum NR in the MS stage (r = 0.633, p = 0.004), and negatively correlated with the death rates (r = −0.500, p = 0.029; ).
Table 4 The partial correlation of total tuber biomass, maximum number of the ramets (NM) and death rate of V. natans during the experiment.
Discussion
In the present study, a moderate NH4+ enrichment (ca. 0.65 mg L−1 NH4–N) in water column enhanced ramet production and reduced the death rate of V. natans, and consequently resulted in more tubers of the plant as compared to those in the treatments without NH4+ enrichment at the end of the experiment. Water column NH4–N concentration in the present study is slightly higher than the concentration (0.56 mg L−1 NH4–N) toxic to V. natans in a field survey reported by Cao et al. (Citation2007); however, it did not inhibit the plant growth as observed by Cao et al. (Citation2007). Considering low PAR commonly occurring in eutrophic lakes along the middle-lower reach of the Yangtze River (Wu et al. Citation2012), the high PAR and the high carbohydrate contents might have alleviated NH4+ toxicity to the plant in our experiment (Cao et al. Citation2009, Citation2011).
As NH4+ toxicity to submersed macrophytes depended on both of NH4+ concentration and light availability (Ariz et al. Citation2011; Cao et al. Citation2011); high NH4–N concentration of 7.5 mg L−1 in water column was not toxic to Vallisneria spinulosa at high PAR (Li et al. Citation2008), while 0.25 mg L−1 NH4–N inhibited the growth of Ceratophyllum demersum and Myriphyllum spicatum at low PAR (Cao et al. Citation2011); and species-specific NH4–N concentrations were reported toxic to different submersed macrophytes, with an NH4–N threshold of ca. 0.25–3.7 mg L−1 (Van Katwijk et al. Citation1997; Cao et al. Citation2007, Citation2011; Li et al. Citation2007). In our experiments, the PAR of 245 μmol m−2 s−1 reached photosynthetic saturation light intensity of V. natans (unpublished data of Cao et al.) and Vallisneria americana (Madsen et al. Citation1991), and was much higher than 120 μmol m−2 s−1 at which 0.81 mg L−1 NH4–N in water inhibited the growth of V. natans (Cao et al. Citation2007). Therefore, the high PAR might have enhanced the tolerance of V. natans to NH4+ enrichment in the present study (Cao et al. Citation2009, Citation2011). Death of V. natans initiated at almost the same time in all treatments, but the NH4+ enrichment reduced the death rates and prolonged the survivorship, which is in consistent with the results usually observed in annual herbaceous crops with supplement of N fertilizer (Dingkuhn et al. Citation1992; Gutiérrez-Boem et al. Citation2004).
In our experiment, the NH4+ enrichment changed C–N metabolism of V. natans greatly, led to increase contents of FAA and N and decreased contents of SC in the leaves and tubers, and decreased the starch contents in the tubers. The similar results were also observed in the leaves and/or rhizomes of many submersed macrophytes under NH4+ stress condition, i.e. Stratiotes aloides, C. demersum, Potamogeton crispus and M. spicatum (Smolders et al. Citation1996; Cao et al. Citation2009, Citation2011). As the leaves of submersed macrophytes cannot prevent the absorption of NH4+ from water column (Van Katwijk et al. Citation1997; Britto et al. Citation2001), to avoid NH4+ accumulation in their tissue, the plants incorporate NH4+ into nitrogenous organic compounds instead of transporting it out of the tissue, processes that both are energy and thus carbohydrate demanding (Britto et al. Citation2001; Britto & Kronzucker Citation2002; Cao et al. Citation2009). Reduction of SC contents of V. natans had been found to inhibit the ramet production as a result of NH4+ stress coincident with low PAR (Cao et al. Citation2007). In the study of Cao et al. (Citation2007), the leaf SC contents dropped to about 50% by the NH4+ enrichment, a value twofold than that examined in the present study. The differences in SC responses of V. natans to NH4+ enrichments in the present study and the study of Cao et al. (Citation2007) implied various photosynthetic carbohydrate production of the plant at different PAR in the two experiments, with more carbohydrate production at high PAR and thus compensating for the SC consumed by the NH4+ enrichment and ensuring carbohydrate requirement for the production of ramets and tubers in our experiment. In eutrophic lakes, stands of V. natans are accessible to high PAR by moving from pelagic to littoral zone, where moderate NH4+ enrichment in water column benefits the expansion of V. natans stands by producing more tubers, in contrast to the decline of the plant stands induced by low PAR and NH4+ enrichment in the pelagic zone.
In the present study, the K+ enrichment did not affect C and N metabolism of V. natans as indicated by the contents of FAA, SC, starch, N and C in the leaves and tubers irrespective of the NH4+ enrichment, indicating that the K+ enrichments did not further prevent the uptake of NH4+ by the plant. Plants absorb K+ by high-affinity transporting system (HATS) and low-affinity transporting system (LATS), which function at low and high external K+ concentrations, respectively (Szczerba et al. Citation2008). The high K+ concentrations in our experiment fall within the working range of K+ concentration of LATS (Szczerba et al. Citation2008) and might not compete with NH4+ for LATS when the concentrations of both K+ and NH4+ were high.
The NH4+ or K+ enrichments enhanced ramet production of V. natans separately at the MS stage; however, their combination decreased the ramet production as compared with the NH4+ enrichment alone. A plausible mechanism for the lower ramet production induced by the K+ and NH4+ enrichments was that massive influx of NH4+ and K+ into the plant cell might have caused H+ to efflux from the cell (Taylor & Bloom Citation1998; Hoopen et al. Citation2010; Marschner & Marschner Citation2012), inducing deionization of NH4+ and accumulation of NH3 in the cell (Hoopen et al. Citation2010), which is about two orders of magnitude more toxic to plants than NH4+ ion (Körner et al. Citation2003; Nimptsch & Pflugmacher Citation2007).
Conclusion
This study aims to examine long-term single effects and co-effects of NH4+ and K+ enrichment in water column on carbon and nitrogen metabolism, life history and asexual reproduction of submersed macrophyte. We found that the K+ enrichment had negligible effects on the plant, and the NH4+ enrichment (0.65 mg L−1 NH4–N) benefited the plant growth but reduced the tuber carbohydrate contents, and their interaction effect on the plant was negligible.
Additional information
Funding
Reference
- Ariz I, Artola E, Asensio AC, Cruchaga S, Aparicio-Tejo PM, Moran JF. 2011. High irradiance increases NH4+ tolerance in Pisum sativum: higher carbon and energy availability improve ion balance but not N assimilation. J Plant Physiol. 168:1009–1015.
- Balkos KD, Britto DT, Kronzucker HJ. 2010. Optimization of ammonium acquisition and metabolism by potassium in rice (Oryza sativa L. cv. IR-72). Plant Cell Environ. 33:23–34.
- Barker AV, Maynard DN, Lachman WH. 1967. Induction of tomato stem and leaf lesions, and potassium deficiency, by excessive ammonium nutrition. Soil Sci. 103:319–327.
- Barko JW, Gunnison D, Carpenter SR. 1991. Sediment interaction with submersed macrophyte growth and community dynamics. Aquat Bot. 41:41–65.
- Best EPH. 1980. Effects of nitrogen on the growth and nitrogenous compounds of Ceratophyllum demersum. Aquat Bot. 8:197–206.
- Britto DT, Kronzucker HJ. 2002. NH4+ toxicity in higher plants: a critical review. J Plant Physiol. 159:567–584.
- Britto DT, Siddiqi MY, Glass AD, Kronzucker HJ. 2001. Futile transmembrane NH4+ cycling: a cellular hypothesis to explain ammonium toxicity in plants. Proc Natl Acad Sci USA. 98:4255–4258.
- Cao T, Ni LY, Xie P, Xu J, Zhang M. 2011. Effects of moderate ammonium enrichment on three submersed macrophytes under contrasting light availability. Freshw Biol. 56:1620–1629.
- Cao T, Xie P, Ni LY, Wu AP, Zhang M, Wu SK, Smolders A. 2007. The role of NH4+ toxicity in the decline of the submersed macrophyte Vallisneria natans in lakes of the Yangtze River basin, China. Mar Freshw Res. 58:581–587.
- Cao T, Xie P, Ni L, Zhang M, Xu J. 2009. Carbon and nitrogen metabolism of an eutrophication tolerative macrophyte, Potamogeton crispus, under NH4+ stress and low light availability. Environ Exp Bot. 66:74–78.
- Cao Y, Glass AD, Crawford NM. 1993. Ammonium inhibition of Arabidopsis root growth can be reversed by potassium and by auxin resistance mutations aux1, axr1, and axr2. Plant Physiol. 102:983–989.
- Chen KN, Lan CJ, Shi LX, Chen WM, Xu H, Bao XM. 2006. [Reproductive ecology of Vallisneria natans]. Chin J Plant Ecol. 30:487–495. Chinese.
- Dingkuhn M, De Datta S, Pamplona R, Javellana C, Schnier H. 1992. Effect of late-season N fertilization on photosynthesis and yield of transplanted and direct-seeded tropical flooded rice. II. A canopy stratification study. Field Crops Res. 28:235–249.
- Dirk L, van der Krol AR, Vreugdenhil D, Hilhors HW, Bewley JD. 1999. Galactomannan, soluble sugar and starch mobilization following germination of Trigonella foenum-graecum seeds. Plant Physiol Biochem. 37:41–50.
- Eaton A, Clesceri L, Greenberg A, Franson M. 1995. Standard methods for the examination of water and wastewater. Washington (DC): American Public Health Association.
- Farnsworth-Lee LA, Baker LA. 2000. Conceptual model of aquatic plant decay and ammonia toxicity for shallow lakes. J Environ Eng-ASCE. 126:199–207.
- Feng JN, Barker AV. 1992. Ethylene evolution and ammonium accumulation by nutrient-stressed tomato plants. J Plant Nutr. 15:137–153.
- Golterman H, Clymo R. 1969. Methods for chemical analysis of freshwater. IBP Handbook, No. 8. Oxford: Blackwell Scientific Publications.
- Gutiérrez-Boem FH, Scheiner JD, Rimski-Korsakov H, Lavado RS. 2004. Late season nitrogen fertilization of soybeans: effects on leaf senescence, yield and environment. Nutr Cycl Agroecosys. 68:109–115.
- Hoopen FT, Cuin TA, Pedas P, Hegelund JN, Shabala S, Schjoerring JK, Jahn TP. 2010. Competition between uptake of ammonium and potassium in barley and Arabidopsis roots: molecular mechanisms and physiological consequences. J Exp Bot. 61:2303–2315.
- Invers O, Kraemer GP, Perez M, Romero J. 2004. Effects of nitrogen addition on nitrogen metabolism and carbon reserves in the temperate seagrass Posidonia oceanica. J Exp Mar Biol Ecol. 303:97–114.
- Kohl JG, Woitke P, Kuhl H, Dewender M, Konig G. 1998. Seasonal changes in dissolved amino acids and sugars in basal culm internodes as physiological indicators of the C/N-balance of Phragmites australis at littoral sites of different trophic status. Aquat Bot. 60:221–240.
- Körner S, Vermaat JE, Veenstra S. 2003. The capacity of duckweed to treat wastewater. J Environ Qual. 32:1583–1590.
- Li HJ, Cao T, Ni LY. 2007. Effects of ammonium on growth, nitrogen and carbohydrate metabolism of Potamogeton maackianus A. Benn. Fundam Appl Limnol. 170:141–148.
- Li W, Zhang Z, Jeppesen E. 2008. The response of Vallisneria spinulosa (Hydrocharitaceae) to different loadings of ammonia and nitrate at moderate phosphorus concentration: a mesocosm approach. Freshwater Biol. 53:2321–2330.
- Lips S, Leidi E, Silberbush M, Soares M, Lewis O. 1990. Physiological aspects of ammonium and nitrate fertilization. J Plant Nutr. 13:1271–1289.
- Madsen JD, Hartleb C, Boylen C. 1991. Photosynthetic characteristics of Myriophyllum spicatum and six submersed aquatic macrophyte species native to Lake George, New York. Freshwater Biol. 26:233–240.
- Marschner H, Marschner P. 2012. Marschner's mineral nutrition of higher plants. Boston (MA): Academic Press.
- Nichols DS, Keeney DR. 1976. Nitrogen nutition of Myriophyllum spicatum uptake and translocation of N15 by shoots and roots. Freshwater Biol. 6:145–154.
- Nimptsch J, Pflugmacher S. 2007. Ammonia triggers the promotion of oxidative stress in the aquatic macrophyte Myriophyllum mattogrossense. Chemosphere. 66:708–714.
- Short FT, McRoy CP. 1984. Nitrogen uptake by leaves and roots of the seagrass Zosera marinal L. Bot Mar. 27:547–555.
- Smolders A, Lamers L, Lucassen E, Van der Velde G, Roelofs J. 2006. Internal eutrophication: how it works and what to do about it – a review. Chem Ecol. 22:93–111.
- Smolders AJP, den Hartog C, van Gestel CBL, Roelofs JGM. 1996. The effects of ammonium on growth, accumulation of free amino acids and nutritional status of young phosphorus deficient Stratiotes aloides plants. Aquat Bot. 53:85–96.
- Su S, Zhou Y, Qin JG, Wang W, Yao W, Song L. 2012. Physiological responses of Egeria densa to high ammonium concentration and nitrogen deficiency. Chemosphere. 86:538–545.
- Szczerba MW, Britto DT, Balkos KD, Kronzucker HJ. 2008. Alleviation of rapid, futile ammonium cycling at the plasma membrane by potassium reveals K+-sensitive and -insensitive components of NH4+ transport. J Exp Bot. 59:303–313.
- Taylor A, Bloom A. 1998. Ammonium, nitrate, and proton fluxes along the maize root. Plant Cell Environ. 21:1255–1263.
- Tsay YF, Ho CH, Chen HY, Lin SH. 2011. Integration of nitrogen and potassium signaling. Annu Rev Plant Biol. 62:207–226.
- Van Katwijk M, Vergeer L, Schmitz G, Roelofs J. 1997. Ammonium toxicity in eelgrass Zostera marina. Mar Ecol Prog Ser. 157:159–173.
- Wu JL, Zeng HA, Yu H, Ma L, Xu LS, Qin BQ. 2012. Water and sediment quality in lakes along the middle and lower reaches of the Yangtze River, China. Water Resour Manag. 26:3601–3618.
- Xiong BH, Li W. 2000. [Ecological studies on Vallisneria L. in China]. J Wuhan Bot Res. 18:500–508. Chinese.
- Yemm E, Cocking E, Ricketts R. 1955. The determination of amino-acids with ninhydrin. Analyst. 80:209–214.
- Yemm E, Willis A. 1954. The estimation of carbohydrates in plant extracts by anthrone. Biochem J. 57:508–514.
- Zhang M, Wang ZQ, Xu J, Liu YQ, Ni LY, Cao T, Xie P. 2011. Ammonium, microcystins, and hypoxia of blooms in eutrophic water cause oxidative stress and C–N imbalance in submersed and floating-leaved aquatic plants in Lake Taihu, China. Chemosphere. 82:329–339.
- Zhang S, Wang G, Pu P, Chigira T. 1999. Succession of hydrophytic vegetation and swampy tendency in the East Taihu Lake. J Plant Resour Environ. 8:1–6.
- Zhong AW, Cao T, Ni LY, Xie P. 2013. Growth and membrane permeability of two submersed macrophytes in response to ammonium enrichment. Aquat Biol. 19:55–64.