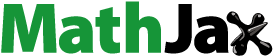
Abstract
Mandarin fish Siniperca chuatsi (Basilewsky) is a commercially important aquaculture species, mainly cultured in Chinese ponds and only fed live fish. We evaluated the effects of artificial vegetation on consumption and growth of mandarin fish foraging on live prey Cirrhinus mrigala (Hamilton) in outdoor pools. In comparison to the 0% vegetation treatment, mandarin fish captured more prey in all treatments where vegetation was added. However, prey consumption did not vary between 15, 30, 45 and 60% vegetation additions. Weight gain of mandarin fish in the 0% vegetation treatment was significantly lower than those in the four vegetated treatments, with the highest weight gain recorded for the 30% vegetation treatment. Similar differences in specific growth rate were observed. The specific growth rate increased with increased vegetation coverage, but then declined slowly as vegetation coverage continued to increase, with a peak in the 30% vegetation treatment. These results suggest that transplanting submerged vegetation into culture ponds in an effort to improve water quality would not affect or even improve prey consumption and growth of mandarin fish, and that suitable vegetation coverage would range between 30% and 60% in terms of growth rate.
Introduction
Mandarin fish, Siniperca chuatsi (Basilewsky) (Percichthyidae), is a demersal piscivore and native to many rivers and lakes from the northeast to the south of China. In China, this fish is commercially harvested, with a market price of 10–14 US dollars kg−1. In the past two decades, annual catches of wild mandarin have declined sharply because of overfishing, habitat degradation and (or) eutrophication. To meet the increasing demand of Chinese consumers, monocultures of mandarin fish fed live prey fish in ponds have become increasingly popular, with an annual yield of 4500–9000 kgˑha−1. However, in pond cultures, the nitrogen and phosphorus levels in water increase gradually with the accumulation of excrement of mandarin fish and its prey fish, and the water quality easily deteriorates, especially in the late stage of aquaculture. Poor water quality frequently leads to a lower survival rate because of an increased risk of disease outbreak. Aquatic macrophytes can efficiently absorb nitrogen, phosphorus and other nutrients in the water, thereby improving water quality (Ghaly et al. Citation2005; Li and Li Citation2009; Sikawa and Yakupitiyage Citation2010; Trang and Brix Citation2014). If some submerged vegetation was added to mandarin fish culture ponds, it is likely that water quality in culture ponds would improve. However, we do not know whether the transplanted vegetation would cause adverse effects on mandarin fish prey consumption and growth.
Previous studies have indicated that vegetation-based habitat complexity influences predator-prey interactions (Hayse and Wissing Citation1996; Warfe and Barmuta Citation2004; Stuart-Smith et al. Citation2007; Camp et al. Citation2012). Effects of habitat complexity on predators vary among predation strategies. Generally, habitat complexity deters foraging activities of chasing predators by interfering with their vision and attack movements, whereas it provides effective refuge for prey species, leading to increased capture time and predator energy expenditures (Savino and Stein Citation1982; Gotceitas Citation1990). Several studies have shown that the growth rate of European perch (Perca fluviatilis Linneaus) and juvenile pinfish (Lagodon rhomboides Linneaus) decreased with increasing vegetation density, as the vegetation inhibited their chasing behavior (Persson and Eklov Citation1995; Spitzer et al. Citation2000). Conversely, a complex habitat might also work as concealment and camouflage for ambush predators, concealing their predatory intent, disturbing swarming behavior of prey (Hobson Citation1979; Flynn and Ritz Citation1999; Gadomski and Parsley Citation2005) and resulting in increased predation success (Ostrand et al. Citation2004; Orrock et al. Citation2013). However, several studies have shown that high levels of habitat structure might disturb the anti-predatory behavior of prey, as well as limit the vision and mobility of the predator (Savino and Stein Citation1982; Winfield Citation1986; Flynn and Ritz Citation1999), whereas intermediate levels of vegetation might allow the fish to can feed efficiently (Winfield Citation1986). Also, Stahr and Shoup (Citation2016) found that there was no effect of habitat complexity on the foraging return of invertivorous (prepiscivorous) juvenile largemouth bass (Micropterus salmoides Lacepede).
To date, there is limited information on the feeding behavior of mandarin fish related to habitat complexity. Mandarin fish feed on only live fish and shrimps (not dead prey or artificial diets) throughout all life-history stages (Liang Citation1996; Li et al. Citation2014; Liu et al. Citation2015). This fish could capture prey fish when either eyes or lateral lines were intact or functional, but scarcely feed when deprived of both of these senses (Liang Citation1996; Liang et al. Citation1998). Thus, vision and mechanoreception play decisive roles in its predation strategy. Light intensity (daytime vs. night) had no significant influence on foraging success of the fish in either clay- or algae-induced turbidity environments (Li et al. Citation2013b). Additionally, mandarin fish larvae (21 days from hatching) and yearlings (total length: 14–16 cm) were able to feed in complete darkness, as well as in light (Liang et al. Citation2008).
In the present study, we evaluated the effect of adding artificial vegetation to the rearing environment on consumption and growth of mandarin fish in a 15-day experiment. The objective of this study was to test the hypotheses that moderate vegetation would not inhibit and could potentially increase prey consumption and growth of mandarin fish. Our findings will be useful for recommending habitat structure additions for improving water quality in pond cultures of mandarin fish.
Materials and methods
Predator and prey
We used mandarin fish as predators and demersal mrigal carp (Cyprinidae: Cirrhinus mrigala (Hamilton)) as prey. Both species were obtained from a fish hatchery in Wuhan City, and transported to the experimental base of the Institute of Hydrobiology, CAS, through cooperation with Wuhan Jiaheng Fisheries Co., Ltd. Mandarin fish were maintained on a diet of mrigal carp in two concrete pools (54 m2 per pool; a water depth of 80–90 cm), fed daily at maintenance rations. Prey fish were maintained in another three similar sized concrete pools, fed a commercial pellet diet twice per day. All experimental fish were allowed to acclimate for 1 week prior to use in predation trials. During the acclimation period, water temperatures, dissolved oxygen, and pH were maintained at 26.4 ± 1.5 °C, 5.8 ± 0.4 mgˑL−1, and 8.4 ± 0.4, respectively.
Experimental design and procedure
The experiment was conducted in 15 sub-pools () of five outdoor concrete pools (6 × 3 × 1 m; 18 m2) from 23 September to 8 October 2015. Prior to the experiment, each experimental pool was partitioned into three equal sub-pools (2 × 3 × 1 m; 6 m2) by two net-barriers with a mesh size of 5 mm. Artificial plastic plants, similar to Vallisneria spiralis, were used in this study, with maximal stem height of 60 cm and one plant crown area of approximately 0.1 m2. To construct vegetated habitats, a total of 270 artificial plants were used and set in a uniform arrangement along one side of each sub-pool for four vegetated treatments (). The plant root was based at the bottom of the pools. This allowed the artificial vegetation to float upwards and occupy the water column similar to rooted aquatic plants. Here, vegetation coverage was determined as the percentage of the area of artificial plants relative to the bottom area of each sub-pool. We used five vegetation treatments including 0% (no plants), 15% (9 plants per sub-pool), 30% (18 plants per sub-pool), 45% (27 plants per sub-pool) and 60% (36 plants per sub-pool), with three replicates for each vegetation treatment. The five vegetation treatments were randomly placed among 15 sub-pools of five pools (). Here, habitat complexity varied with an increase in vegetation coverage (Bean and Winfield Citation1995).
Figure 1. Experimental design. Five vegetation treatments randomly placed among 15 sub-pools (2 × 3 × 1 m) of five pools (6 × 3 × 1 m). A, B, C, D, and E indicate 0, 15, 30, 45 and 60% vegetation treatments, respectively. The broken lines indicate net barriers used to partition each pool into three equal sub-pools.
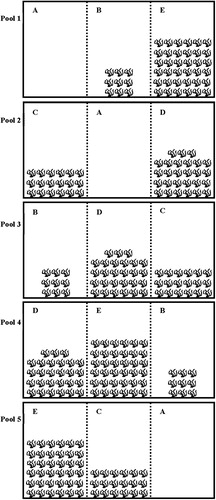
To reduce the influence of size-related differences in predation, the prey fish used for the experiment were relatively uniform in size (n = 137, L = 7.13 ± 0.53 cm; W = 2.95 ± 0.76 g), and fed a commercial pellet diet daily. The prey fish ranged in size from 30% to 40% of mandarin fish L and were within optimal sizes determined as prey for mandarin fish (Li et al. Citation2013a).
Prior to fish introduction into the experimental sub-pools, mandarin fish were fasted for 24 h, and 45 mandarin fish with relatively uniform size were selected from their holding pools, lightly anesthetized with eugenol and their total length (L) and body weight (W) measured (n = 45, L = 22.3 ± 0.62 cm; W = 193.2 ± 17.5 g). These mandarin fish were then randomly placed into the 15 experimental sub-pools (three individuals per sub-pool), where they were held 6 h without food to allow for acclimatization. Subsequently, mandarin fish in the experimental sub-pools were fed with live prey from their holding pools at the same ration across treatments. A total of three feeding trials for the mandarin fish were completed during the experiment. The prey released at each feeding was sufficient to meet the predation demand of mandarin fish. The feeding dates were 23 September, 28 September and 3 October ().
Table 1. The number and biomass of live prey Cirrhinus mrigala (Hamilton) released during the 15-day experiment, the number and biomass of the prey that survived at the end of the experiment, and the prey consumption of mandarin fish Siniperca chuatsi (Basilewsky) in five vegetation treatmentsTable Footnote*.
At the end of the experiment, we counted and weighed remaining prey fish in each of the 15 experimental sub-pools (). Also, the L and W of all individuals of mandarin fish in the experiment were measured. During the experiment, ambient photoperiods were maintained, water temperatures ranged from 22 to 27 °C, dissolved oxygen was 5.0–6.41 mgˑL−1, and pH 7.8–8.8.
Data collection and statistical analyses
The prey consumption (PC) of mandarin fish was calculated as:
where N1 was the total number of prey fish released during the experiment and N2 was the number of prey fish surviving at the end of the experiment.
Condition factor (CF) was calculated as:
where L and W were the total length (cm) and weight (g) of mandarin fish, respectively.
Weight gain (WG) was calculated as:
where W2 and W1 were the final and initial weight (g) of mandarin fish in the experiment, respectively.
The specific growth rate (SGR) was calculated as:
where W2 and W1 were the final and initial weight (g) of mandarin fish, respectively, d is the experimental period (15 days).
All mean values are presented with the accompanying standard deviation (SD). Difference between two mean values was assessed by a Student’s t-test. Difference in length, weight, condition factor, prey consumption and weight gain among different vegetation treatments was analyzed by one-way ANOVA, and Tukey’s HSD was applied for multiple comparisons. SGR data were not normally distributed, so the Kruskal-Wallis (K-W) test was used to test differences for the SGR of treatment groups. Differences were considered to be significant when p < 0.05. All data were analyzed with SPSS 22.0 (Systat Inc., Chicago, IL, USA).
Results
Prey consumption of mandarin fish
The total number of prey fish released in each of the 15 experimental sub-pools in the five vegetation treatments was equal and their total biomass was not significantly different when comparing groups ().
During the experiment, we did not observe any dead prey fish in their three holding pools with no predators. Experimental deaths were, therefore, attributed to predation by mandarin fish. Prey consumption of mandarin fish in the 0% vegetation treatment (212.0 ± 13.2) was significantly lower than that in the 15% and 30% vegetation treatments (293.7 ± 12.7; 267.3 ± 25.0) (F = 7.708, df = 14, p = 0.04; Turkey HSD: p = 0.003; p = 0.033), but not significantly different from that in the 45% and 60% vegetation treatments (256.3 ± 16.1; 251.0 ± 24.3) (F = 7.708, df = 14, p = 0.04; Turkey HSD: p = 0.098; p = 0.164) (). In the four vegetated treatments, we detected no significant differences in prey consumptions (Turkey HSD: all p > 0.05), but detected a downward trend with an increase in vegetation coverage. These results revealed that adding vegetation with 15–60% coverage could increase prey consumption of mandarin fish. In particular, there was higher consumption between 15% and 30% coverage.
Biological indices of mandarin fish
At the start of the experiment, mandarin fish used for the five vegetation treatments were uniform in L and CF (L: F = 1.636, df = 44, p = 0.184; CF: F = 1.643, df = 44, p = 0.182), whereas there was a significant difference (F = 2.999, df = 44, p = 0.03; Turkey HSD: p = 0.042) in W between the 15% and 60% vegetation treatments ().
Figure 2. Body weight (a), total length (b) and condition factor (c) of mandarin fish in different vegetation treatments at the beginning (dotted bars) and end (filled bars) of the experiment. Different lowercase letters above bars between treatments indicate significant differences (p < 0.05) in initial body weight, total length, and condition factor of mandarin fish. Different uppercase letters above bars between treatments indicate significant differences (p < 0.05) in final body weight, total length, and condition factor of mandarin fish. Vertical lines are +1 SD.
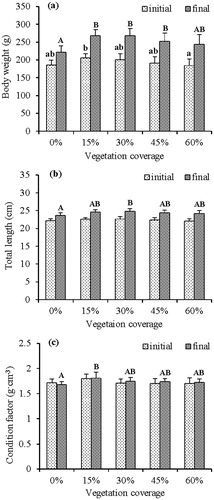
At the end of the experiment, the W of mandarin fish in the 0% vegetation treatment was significantly lower than those in the 15, 30 and 45% vegetation treatments (F = 7.696, df = 44, p < 0.001; Turkey HSD: p < 0.001; p < 0.001; p = 0.026), but not significantly different from that of the 60% vegetation treatment (Turkey HSD: p = 0.223). Further, there were no significant differences in W when comparing the 15, 30, 45 and 60% vegetation treatments (Turkey HSD: all p > 0.05) (). Similarly, the L of mandarin fish in the 0% vegetation treatment was significantly lower than that in the 30% vegetation treatment (F = 3.457, df = 44, p = 0.016; Turkey HSD: p = 0.011) (), but not significantly different from those in the 15, 45 and 60% vegetation treatments (Turkey HSD: all p > 0.05). The CF of mandarin fish in the 0% vegetation treatment was significantly lower than that in the 15% vegetation treatment (F = 3.253, df = 44, p = 0.021; Turkey HSD: p = 0.008), but not significantly different from those in the 30, 45 and 60% vegetation treatments (Turkey HSD: all p > 0.05) ().
The growth of mandarin fish
The weight gain of mandarin fish in the 0% vegetation treatment was significantly lower than those in the four vegetated treatments (F = 10.806, df = 44, p < 0.001; Turkey HSD: p < 0.001; p < 0.001; p < 0.001; p = 0.001, respectively). No significant differences in weight gain were observed between the 15, 30, 45 or 60% vegetation treatments (Turkey HSD: all p > 0.05). When vegetation coverage increased to 30%, weight gain reached its highest, then declined slowly with increased vegetation coverage (). Similar results were observed in SGR. SGR in the 0% vegetation treatment was not significantly different than the 15% vegetation treatment (Kruskal-Wallis Test, T = 0.02, df = 4, n = 45, p = 0.088), but significantly lower than that in the 30, 45 and 60% vegetation treatments (p = 0.013; p = 0.007; p = 0.003, respectively). There were no significant differences in SGR between 15, 30, 45 or 60% vegetation treatments (all p > 0.05) (). These results indicated that adding vegetation with 15–60% coverage to the environment could improve the growth of mandarin fish, and the growth rate of the fish was greater with 30–60% coverage.
Discussion
Our results showed that adding vegetation with a 15–30% coverage to the environment increased significantly prey consumption of mandarin fish, compared to the 0% vegetation treatment; no significant differences in prey consumption were observed among the 15, 30, 45 and 60% vegetation treatments. Numerous studies have reported a decrease in predator foraging efficiency, such as predation rate, number of prey consumed and foraging success, with increasing habitat complexity because of the inhibition of predator movement or by providing refuge for prey (Stein Citation1977; Heck and Thoman Citation1981; Gotceitas and Colgan Citation1989; Persson and Eklov Citation1995; MacRae and Jackson Citation2001; Valley and Bremigan Citation2002; Alexander et al. Citation2015). Most of this work was done with predators that use a search and attack strategy. Little work has been conducted to examine the effect of habitat complexity on ambush predation (Coen et al. Citation1981; Howard and Koehn Citation1985; James and Heck Citation1994; Flynn and Ritz Citation1999; Ostrand et al. Citation2004). Several studies have suggested that habitat complexity might aid ambush predator foraging, instead of inhibiting it, by offering the predator camouflage and reducing predator visibility to prey (Coen et al. Citation1981; Howard and Koehn Citation1985; Flynn and Ritz Citation1999). In contrast, small pipefish (Syngnathus fuscus Storer) that employs an ambush predation style did not respond to changes in habitat complexity when foraging on amphipods (Ryer Citation1988). Also, vegetation density did not affect the number of fathead minnows (Pimephales promelas Rafinesque) captured by adult spotted gar (Lepisosteus oculatus Winchell), an ambush predator (Ostrand et al. Citation2004). Similarly, the number of shrimp, Hippolyte zostericola (Smith), captured by an ambush predator, the lined sea horse (Hippocampus erectus Perry), was not influenced by increasing habitat complexity (James and Heck Citation1994). Thus, predator species and predation strategy efficiently affect the impacts of habitat complexity on predation.
Species-specific foraging may be on account of the flexible choice of the predator (Chick and McIvor Citation1997; Valinoti et al. Citation2011; Lyons et al. Citation2016) and prey (Gotceitas and Colgan Citation1989; Ostrand et al. Citation2004; DeBoom and Wahl Citation2013) in different refuge habitats. To gain maximal net energy in variable habitats, predators might alter their hunting modes with the changes in choice and distribution of prey, regardless of its predation type (Savino and Stein Citation1989a; Scharf et al. Citation2006; Murray et al. Citation2016). For example, in a low complexity habitat, lined seahorse, fish assemblages in the Novigrad Sea, and larvae of the diving water beetle (Dytiscus spp. Wehncke) were active searchers, whereas almost entirely conducted ambushing or stalk-attacking tactics under high structural complexity (James and Heck Citation1994; Michel and Adams Citation2009; Schultz and Kruschel Citation2010). In the present study, mandarin fish consumed more prey in vegetated treatments compared to unvegetated treatment with no significant differences in prey consumption between vegetated treatments. This may be explained by changes in predation behavior of the mandarin fish. Juveniles and adult mandarin fish use stalk-attacking, followed by ambushing, and chase-attacking modes to capture prey, with greatest capture success exhibited when ambushing and lowest capture success when chase-attacking (Cui Citation2013). Moreover, mandarin fish tend to inhabit vegetated area of the indoor tanks, with increased capture success (Cui Citation2013). It is generally believed that chase-attack predation is less successful than ambush predation or stalk-attack (Schultz and Kruschel Citation2010; Cui Citation2013). Therefore, in the artificial vegetation treatments, mandarin fish could consume more prey, perhaps by offering predator camouflage; thus, reducing predator visibility to prey, and allowing more ambush predation and increasing capture success.
In the present study, mandarin fish exhibited better growth (e.g. weight gain) in the 15, 30, 45 and 60% vegetation treatments compared to the 0% vegetation treatment. No significant differences were observed in weight gain and SGR among the four vegetated treatments, and weight gain and SGR tended to correlate with prey consumption. Thus, rapid growth resulted from increased prey consumption of mandarin fish in the four vegetated treatments. In addition, prey consumption by mandarin fish in the 0% vegetation treatment was the lowest, but not significantly different from those in the 45% and 60% vegetation treatments; in contrast, both weight gain and SGR of the former were significantly lower than those of the latter. Fish reared in bare tanks without visual barriers often experience continual interaction among conspecifics and display aggressive or competitive interactions towards other fish, resulting in disrupted feeding (Barton and Schreck Citation1987; Cooke et al. Citation2003). Visual barriers can act to decrease unnecessary activity and aggression for some fish species (Sundbaum and Näsland Citation1998). This suggests that other factors, such as lower foraging energy cost and basal metabolism, contributed to the faster growth of mandarin fish in the four vegetated treatments. For mandarin fish, we hypothesized that vegetation in pools would allow predators to disperse or hide, thereby decreasing foraging activity, increasing capture success, and reducing stress. Rather than spending more time pursuing prey in open water, northern pike (Esox lucius) adopt an ambush strategy in vegetation, which has a higher capture success and lower energy costs; thus, the predator would achieve greater growth (Savino and Stein Citation1989b).
Contrary to the results of the present study, the growth rate of the piscivorous perch (Perca Fluviatilis Linneaus) and juvenile pinfish (Lagodon rhomboids Linneaus) decreased with increased refuge efficiency, as complex habitat protected prey from predators, and in turn, increased the capture time and foraging energetic cost of predators (Diehl Citation1993; Persson and Eklov Citation1995; Spitzer et al. Citation2000). In another study, vegetation decreased the startle and crowding stress for Tiger Muskellunge (Muskellunge Esox masquinongy Mitchill × Northern Pike E. lucius Linneaus), but growth was not significantly different between non-vegetated and semi-vegetated treatments (Einfalt et al. Citation2013).
In summary, we used artificial vegetation coverage to represent habitat complexity and evaluated the effect of vegetation-structural complexity on prey consumption and growth of mandarin fish. Compared to the 0% vegetation treatment, the 15% and 30% vegetation treatments significantly enhanced prey consumption and the 45% and 60% vegetation treatments did not affect prey consumption of mandarin fish. In addition, the 30, 45 and 60% vegetation treatments significantly improved specific growth rate of mandarin fish. Hence, we recommended transplanting submerged vegetation in culture ponds to improve water quality while simultaneously improving prey consumption and growth of mandarin fish, and suitable vegetation coverage could range between 30% and 60% in terms of growth rate.
Notes on contributors
Yan Ren is a PhD candidate at the State Key Laboratory of Freshwater Ecology and Biotechnology in Institute of Hydrobiology, Chinese Academy of Sciences, with research interests in pond culture and ecology of mandarin fish.
Mantang Xiong is a PhD candidate at the State Key Laboratory of Freshwater Ecology and Biotechnology in Institute of Hydrobiology, Chinese Academy of Sciences, with research interests in fish ecology and fisheries in reservoirs.
Jixin Yu is a PhD candidate at the State Key Laboratory of Freshwater Ecology and Biotechnology in Institute of Hydrobiology, Chinese Academy of Sciences, with research interests in crayfish ecology and culture in rice fields.
Wei Li is an associate professor at the State Key Laboratory of Freshwater Ecology and Biotechnology in Institute of Hydrobiology, Chinese Academy of Sciences, with research interests in pond culture and lake fisheries.
Bo Li is a researcher in Wuhan Academy of Agricultural Sciences with research interests in artificial propagation and culture of freshwater fish.
Jiashou Liu is a professor at the State Key Laboratory of Freshwater Ecology and Biotechnology in Institute of Hydrobiology, Chinese Academy of Sciences, with research interests in aquaculture and fisheries.
Tanglin Zhang is a professor at the State Key Laboratory of Freshwater Ecology and Biotechnology in Institute of Hydrobiology, Chinese Academy of Sciences, with research interests in pond culture and lake fisheries.
Acknowledgements
This study was financially supported by the R & D Project (Grant Nos. 2015BAD13B02; 2012BAD25B05) of the Ministry of Science and Technology of China; Key Deployment Project (Grant No. KFZD-SW-106), Chinese Academy of Science, and the National Natural Science Foundation of China (Grant No. 31201994).
Disclosure statement
No potential conflict of interest was reported by the authors.
References
- Alexander ME, Kaiser H, Weyl OLF, Dick JTA. 2015. Habitat simplification increases the impact of a freshwater invasive fish. Environ Biol Fish. 98(2):477–486.
- Barton BA, Schreck CB. 1987. Metabolic cost of acute physical stress in juvenile Steelhead. Trans Am Fish Soc. 116(2):257–263.
- Bean CW, Winfield IJ. 1995. Habitat use and activity patterns of roach (Rutilus rutilus (L.)), rudd (Scardinius erythrophthalmus (L.)), perch (Perca fluviatilis (L.)) and pike (Esox lucius (L.)) in the laboratory: the role of predation threat and structural complexity. Ecol Freshwat Fish. 4(1):37–46.
- Camp EV, Gwinn DC, Iii WEP, Frazer TK. 2012. Changes in submersed aquatic vegetation affect predation risk of a common prey fish Lucania parva (Cyprinodontiformes: Fundulidae) in a spring-fed coastal river. Fisheries Manage Ecol. 19(3):245–251.
- Chick JH, McIvor CC. 1997. Habitat selection by three littoral zone fishes: effects of predation pressure, plant density and macrophyte type. Ecol Freshwat Fish. 6:122–122.
- Coen LD, Heck KL, Abele LG. 1981. Experiments on competition and predation among shrimps of seagrass meadows. Ecology. 62(6):1484–1493.
- Cooke SJ, Steinmetz J, Degner JF, Grant EC, Philipp DP. 2003. Metabolic fright responses of different-sized Largemouth Bass (Micropterus salmoides) to two avian predators show variations in nonlethal energetic costs. Can J Zool. 81(4):699–709.
- Cui F. 2013. Studies on the predatory behavior of mandarin fish (Siniperca chuatsi) as one species of piscivore (pp. 1–102) [Master thesis]. Beijing (China): University of Chinese Academy of Sciences. Chinese.
- DeBoom CS, Wahl DH. 2013. Effects of coarse woody habitat complexity on predator–prey interactions of four freshwater fish species. Trans Am Fish Soc. 142(6):1602–1614.
- Diehl S. 1993. Effects of habitat structure on resource availability, diet and growth of benthivorous perch, Perca Fluviatilis. Oikos. 67(3):403–414.
- Einfalt LM, Wojcieszak DB, Wahl DH. 2013. Behavior, growth and habitat selection of hatchery esocids reared with artificial vegetation. Trans Am Fish Soc. 142(2):345–352.
- Flynn AJ, Ritz DA. 1999. Effect of habitat complexity and predatory style on the capture success of fish feeding on aggregated prey. J Mar Biol Ass. 79(3):487–494.
- Gadomski DM, Parsley MJ. 2005. Effects of turbidity, light level, and coverage on predation of white sturgeon larvae by prickly sculpins. Trans Am Fish Soc. 134(2):369–374.
- Ghaly AE, Kamal M, Mahmoud NS. 2005. Phytoremediation of aquaculture wastewater for water recycling and production of fish feed. Environ Int. 31(1):1–13.
- Gotceitas V. 1990. Variation in plant stem density and its effects on foraging success of juvenile bluegill sunfish. Environ Biol Fish. 27(1):63–70.
- Gotceitas V, Colgan P. 1989. Predator foraging success and habitat complexity: quantitative test of the threshold hypothesis. Oecologia. 80(2):158–166.
- Hayse JW, Wissing TE. 1996. Effects of stem density of artificial vegetation on abundance and growth of age-0 bluegills and predation by largemouth bass. Trans Am Fish Soc. 125(3):422–433.
- Hobson ES. 1979. Interactions between piscivorous fishes and their prey. In: Stoud RH, Clepper H, editors. Predator-prey systems in fisheries management. Washington (DC): Sport Fishing Institute; pp. 231–241
- Howard RK, Koehn JD. 1985. Population dynamics and feeding ecology of pipefish (Syngnathidae) associated with eelgrass beds of Western Port, Victoria. Mar Freshwat Res. 36(3):361–370.
- James PL, Heck KL. 1994. The effects of habitat complexity and light-intensity on ambush predation within a simulated seagrass habitat. J Exp Mar Biol Ecol. 176:187–200.
- Heck KL, Jr, Thoman TA. 1981. Experiments on predator-prey interactions in vegetated aquatic habitats. J Exp Mar Biol Ecol. 53(2-3):125–134.
- Li W, Hicks BJ, Guo CB, Li ZJ, Liu JS, Zhang TL. 2014. Does hatchery-reared Siniperca chuatsi (Actinopterygii, Perciformes) compete significantly with two wild Siniperca populations for diets in a shallow lake? Hydrobiologia. 741(1):125–138. doi:10.1007/s10750-014-1866-9
- Li W, Li Z. 2009. In situ nutrient removal from aquaculture wastewater by aquatic vegetable Ipomoea aquatica on floating beds. Water Sci Technol. 59(10):1937–1943.
- Li W, Zhang TL, Ye SW, Liu JS, Li ZJ. 2013a. Feeding habits and predator-prey size relationships of mandarin fish Siniperca chuatsi (Basilewsky) in a shallow lake, central China. J Appl Ichthyol. 29(1):56–63.
- Li W, Zhang TL, Zhang CW, Li ZJ, Liu JS, Hicks BJ. 2013b. Effects of turbidity and light intensity on foraging success of juvenile mandarin fish Siniperca chuatsi (Basilewsky). Environ Biol Fish. 96(8):995–1002.
- Liang XF. 1996. The structure and behavioural response of lateral line of Siniperca chuatsi in relation to its feeding habit. Ocean Limnol Sin Chin. 27:457–462.
- Liang XF, Kiu JK, Huang BY. 1998. The role of sense organs in the feeding behaviour of Chinese perch. J Fish Biol. 52(5):1058–1067.
- Liang XF, Lin XT, Li SQ, Liu JK. 2008. Impact of environmental and innate factors on the food habit of Chinese perch Siniperca chuatsi (Basilewsky) (Percichthyidae). Aquac Res. 39(2):150–157.
- Liu LW, Liang XF, Fang JG. 2015. The optimal stocking density for hybrid of Siniperca chuatsi (female) x Siniperca scherzeri (male) mandarin fish fed minced prey fish. Aquac Res. 48(3):1342–1345.
- Lyons C, Eckert G, Stoner AW. 2016. Influence of temperature and congener presence on habitat preference and fish predation in blue (Paralithodes platypus Brandt, 1850) and red (P. camtschaticus Tilesius, 1815) king crabs (Anomura: Lithodidae). J Crustacean Biol. 36(1):12–22.
- MacRae PSD, Jackson DA. 2001. The influence of smallmouth bass (Micropterus dolomieu) predation and habitat complexity on the structure of littoral fish assemblages. Can J Fish Aquat Sci. 58:342–351.
- Michel MJ, Adams MM. 2009. Differential effects of structural complexity on predator foraging behavior. Behavior Ecol. 20(2):313–317.
- Murray GPD, Stillman RA, Britton JR. 2016. Habitat complexity and food item size modify the foraging behaviour of a freshwater fish. Hydrobiologia. 766(1):321–332.
- Orrock JL, Preisser EL, Grabowski JH, Trussell GC. 2013. The cost of safety: refuges increase the impact of predation risk in aquatic systems. Ecology. 94(3):573–579.
- Ostrand KG, Braeutigam BJ, Wahl DH. 2004. Consequences of vegetation density and prey species on spotted gar foraging. Trans Am Fish Soc. 133(3):794–800.
- Persson L, Eklov P. 1995. Prey refuges affecting interactions between piscivorous perch and juvenile perch and roach. Ecology. 76(1):70–81.
- Ryer CH. 1988. Pipefish foraging: effects of fish size, prey size and altered habitat complexity. Mar Ecol Prog Ser. 48:37–45.
- Savino JF, Stein RA. 1982. Predator-prey interaction between largemouth bass and bluegills as influenced by simulated, submersed vegetation. Trans Am Fish Soc. 111(3):255–266.
- Savino JF, Stein RA. 1989a. Behavioral interactions between fish predators and their prey: effects of plant-density. Anim Behav. 37:311–321.
- Savino JF, Stein RA. 1989b. Behavior of fish predators and their prey: habitat choice between open water and dense vegetation. Environ Biol Fish. 24(4):287–293.
- Scharf I, Nulman E, Ovadia O, Bouskila A. 2006. Efficiency evaluation of two competing foraging modes under different conditions. Am Nat. 168(3):350–357.
- Schultz ST, Kruschel C. 2010. Frequency and success of ambush and chase predation in fish assemblages associated with seagrass and bare sediment in an Adriatic lagoon. Hydrobiologia. 649(1):25–37.
- Sikawa DC, Yakupitiyage A. 2010. The hydroponic production of lettuce (Lactuca sativa L) by using hybrid catfish (Clarias macrocephalus×C. gariepinus) pond water: potentials and constraints. Agric Water Manage. 97(9):1317–1325.
- Spitzer PM, Mattila J, Heck KL. 2000. The effects of vegetation density on the relative growth rates of juvenile pinfish, Lagodon rhomboides (Linneaus), in Big Lagoon, Florida. J Exp Mar Biol Ecol. 244(1):67–86.
- Stahr KJ, Shoup DE. 2016. The effects of macrophyte stem density and structural complexity on foraging return of invertivorous juvenile largemouth bass. N Am J Fish Manage. 36(4):788–792.
- Stein RA. 1977. Selective predation, optimal foraging, and the predator-prey interaction between fish and crayfish. Ecology. 58(6):1237–1253.
- Stuart-Smith RD, Stuart-Smith JF, White RWG, Barmuta LA. 2007. The impact of an introduced predator on a threatened galaxiid fish is reduced by the availability of complex habitats. Freshwat Biol. 52(8):1555–1563.
- Sundbaum K, Näslund I. 1998. Effects of woody debris on the growth and behaviour of Brown Trout in experimental stream channels. Can J Zool. 76(1):56–61.
- Trang NTD, Brix H. 2014. Use of planted biofilters in integrated recirculating aquaculture-hydroponics systems in the Mekong Delta, Vietnam. Aquac Res. 45(3):460–469.
- Valinoti CE, Ho CK, Armitage AR. 2011. Native and exotic submerged aquatic vegetation provide different nutritional and refuge values for macroinvertebrates. J. Exp Mar Biol Ecol. 409(1-2):42–47.
- Valley RD, Bremigan MT. 2002. Effects of macrophyte bed architecture on largemouth bass foraging: implications of exotic macrophyte invasions. Trans Am Fish Soc. 131(2):234–244.
- Warfe DM, Barmuta LA. 2004. Habitat structural complexity mediates the foraging success of multiple predator species. Oecologia. 141(1):171–178.
- Winfield IJ. 1986. The influence of simulated aquatic macrophytes on the zooplankton consumption rate of juvenile roach, Rutilus rutilus, rudd, Scardinius erythrophthalmus, and perch, Perca fluviatilis. J Fish Biol. 29:37–48.