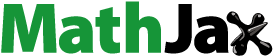
Abstract
From November 2016 to April 2017, the decomposition of Carex. cinerascens Kükenth litter along the natural gradients of groundwater level at Poyang Lake wetland beach were simulated using litterbag technique. The depth of the underground water table of the G-L was −25 to −50 cm, while those of G-ML, G-MH and G-H ranged from −15 to −25 cm, −5 to −15 cm, and −5 to 5, respectively. Additionally, the dry weight loss rate increased gradually with increasing days, with that of G-H being highest, followed by G-MH, G-ML and G-L. The decomposition rate of the four gradients increased rapidly to day 30, then decreased slowly until stabilizing. Additionally, the decomposition rate was highest in G-H, followed by G-MH, G-ML and G-L. The Olson negative exponential model could simulate the decomposition good and predicted the time required for 50% decomposition of the G-H, G-MH, G-ML and G-L gradients was about 166, 197, 205 and 247 days, respectively, while required for 95% was about 1.97, 2.23, 2.43 and 2.92 years, respectively. The overall trend in the relative return index of carbon, nitrogen and phosphorus increased with increasing decomposition days, and the order was G-H > G-MH > G-ML > G-L. Differences in soil pH, bulk density, soil total nitrogen, soil organic matter, microbial biomass carbon and soil sand content in the decomposed environment due to groundwater had a significant impact on decomposition and nutrient release. The relative return index of carbon was significantly positively correlated with soil pH, but significantly negatively correlated with soil bulk density, soil nitrogen content, microbial biomass nitrogen and soil organic matter. The above results show that groundwater is the key factor influencing litter decomposition in Poyang Lake wetland during the dry season, with higher groundwater levels promoting the decomposition.
1. Introduction
Litter decomposition is a key link in regulating nutrient biogeochemical cycles in wetlands, as well as a key process of linking matter and energy fixation and return and is an important ecological process to maintain energy flow, material circulation and nutrient balance in wetland ecosystem (Alexandra et al. Citation2013; Mitsch and Gosselink Citation2015). Wetland plants have high productivity, and the decomposition process of litter will directly affect the return and accumulation of essential nutrients such as carbon, nitrogen and phosphorus in wetland ecosystems (Xiong and Nilsson Citation1999; Chapin et al. Citation2002). Indeed, more than 90% of nitrogen and phosphorus and about 60% of mineral nutrients absorbed by wetland plants are derived from the decomposition of plant litter (Chapin et al. Citation2002). Litter decomposition can even affect the construction of plant communities and competition among populations by affecting the reproduction, germination, growth, species abundance and aboveground biomass of new plants (Xiong and Nilsson Citation1999). In addition, litter decomposition is a key progress in wetland as it relates to global change. Because of the waterlogging and anaerobic characteristics of wetlands, as well as their low nitrogen utilization efficiency, and lower decomposition rate, wetlands become an important carbon reservoir (Currey et al. Citation2009). Accordingly, acceleration or slowdown of the litter decomposition process can cause significant changes in carbon and nitrogen pools locally, regionally, and even globally (Zhao et al. Citation2015; Mueller et al. Citation2018), which in turn affects climate change. These problems have been fully investigated in forest ecosystems, grassland ecosystems and farmland ecosystems, but little information is available for wetland ecosystems (Sun et al. Citation2012). Therefore, it is important to study the decomposition process and nutrient dynamics of wetland litter to improve understanding of the global scale carbon-nitrogen biogeochemical cycle as well as to contribute to the protection and management of wetlands.
Research regarding decomposition of litter in wetlands conducted to date has focused on the changes in material components (Davis et al. Citation2003; Zhang et al. Citation2018), element release features during the process of decomposition (Neckles and Neill Citation1994; Davis et al. Citation2003; Kalbitz et al. Citation2006; Zhao et al. Citation2015), influence factor analysis (Rejmánková and Houdková Citation2006; Ferreira and Chauvet Citation2011; Alexandra et al. Citation2013) and responses to global change (Mueller et al. Citation2018). However, few studies have investigated the regulate factors of litter decomposition and the characteristics of nutrient release such as carbon, nitrogen and phosphorus under different ground water conditions. The decomposition process of plant litter is subject to many internal factors such as plant community type, physical and chemical quality characteristics of the litter itself (Chimney and Pietro Citation2006; Yan Baoshan and Zheyuan Citation2012), and external factors such as water status (Langhans and Tockner Citation2006; Peltoniemi et al. Citation2012), temperature status, microbial status (Ferreira and Chauvet Citation2011) and the influence of environmental conditions such as nutrient supply and availability of exogenous nutrient sources in wetland ecosystems (Rejmánková and Houdková Citation2006). A large number of studies have shown that hydrological processes (such as groundwater level, depth, duration and frequency of flooding) are the main factors affecting the decomposition of litter (Day Citation1983; Valk et al. Citation1991; Neckles and Neill Citation1994; Langhans and Tockner Citation2006; Hou et al. Citation2012; Peltoniemi et al. Citation2012; Alexandra et al. Citation2013; Wu et al. Citation2017). Wetland water condition has great influence on leaching process and microbial degradation processes. Specifically, increasing water can increase the leaching rate of soluble substances in litter, which can shorten the retention time of nutrient leaching and retention and enhance the microbial utilization efficiency of nutrients in litter, thereby promoting decomposition. Conversely, if water is reduced, the leaching rate of soluble matter in litter will decrease, as will the microbial activity, which will inhibit the decomposition (Valk et al. Citation1991; Neckles and Neill Citation1994). However, excessive water content for a long period of time may also inhibit the decomposition of litter by inducing an anaerobic environment, and microbial activity will decrease in anaerobic environment, which will decrease microbial activity. Under anaerobic conditions, the decomposition of roots is significantly affected and the decrease in decomposition rate becomes greater with increasing water depth (Neckles and Neill Citation1994; Langhans and Tockner Citation2006). The main role of fungi and bacterial are to crush litter and change its physical and chemical properties (e.g. softening and crushing through mycelium) making litter more susceptible to invasion by detrital invertebrates (Suberkropp Citation1995). It is not the higher groundwater level or the deeper the water in the wetland that promotes the decomposition. Long-term over wetness or water accumulation will reduce the content of O2 and CO2 in the litter and thus significantly slow down the decomposition process (Sun et al. Citation2012). As the amount of water in the decomposing environment increases and a long-term accumulation of water occurs, the O2 in the litter will be rapidly depleted and the metabolism of the decomposing microorganisms will be suppressed (Laiho et al. Citation2004). Anderson and Smith found that the decomposition rate of litter was the lowest during long-term flooding and the highest in short-term flooding (Anderson and Smith Citation2002). Zhao et al. found that the dry weight loss rate of litter was the highest in areas subjected to long-term flooding, followed by wetlands subjected to flooding for a moderate length of time and then those subjected to short-term flooding (Zhao et al. Citation2015). However, Edward and Day studied that the root decomposition of plants was the slowest in the places in which soil water supersaturation was the longest (Tupacz and Day Citation1990). Additionally, Wrubleski et al. showed that there were no significant differences in dry mass loss rate of litter of four wetland plants under the conditions of unflooded or flooded conditions or different depths of flooding (Wrubleski et al. Citation1997). Although previous studies of litter decomposition in wetlands have provided many important results, the effects of ground water condition on litter decomposition and element dynamics in wetlands is still unclear and worthy of further study.
Poyang Lake, the largest freshwater lake in China, covers an area about 3283 km2. Poyang Lake Wetland is the most important overwintering area East Asia–Australasia Flyway of migratory birds, and is the only way and important habitat for the migration of many migratory birds (Lei Mark and Gang Citation2008), and plays an important role in maintaining the unique biota of the Yangtze River flood plain ecosystem. Poyang Lake wetland is affected by the water coming from the watershed and the water level of the Yangtze River. The boundary between the wet season and the dry season is obvious during the year, and differences in the water level can reach more than 10 m in the flood period and dry period (Dai He and Rong-Rong Citation2017). This unique hydrological variation makes Poyang Lake wetland a typical seasonal flood plain wetland with a large area of wet and dry alternate wetlands. During the dry season, the groundwater level changes along the different elevations of the continental beach are obviously zonal. Therefore, the Poyang Lake wetland is an excellent natural experimental site for studying the effects of water conditions on the decomposition and elemental dynamics of wetland litter.
Carex. cinerascens Kükenth is one of the earliest sprouting plants in the Poyang Lake wetland during the dry season, and is the most dominant and the most widely distributed hygrophyte plant in Poyang Lake wetland. Carex. cinerascens Kükenth mainly distributes on the beach at the middle elevation. The height of the plant is about 40–90 cm, and the covering of canopy is about 60–100 cm. The autumn grass sprouts on the beach after the water recession in September every year and grows until December to March of the next year. During winter, the aboveground part begins to wither and decompose gradually. After January, the ‘spring grass’ sprouts again before the ‘autumn grass’ has all died, and this grows until April when it is gradually submerged by the lake water and then die and are decomposed (Zhang Yu and Hu Citation2013). The matching of sprouting period of Carex. cinerascens Kükenth and overwintering period of waterfowl makes it an important food source for overwintering herbivorous waterfowl, especially geese and ducks in Poyang Lake [Guan et al. Citation2014).
There is a large distribution area and high productivity of Carex. cinerascens Kükenth in Poyang Lake Wetland. Although its growth process has a huge carbon and nitrogen fixation ability, its litter decomposition will also release a large amount of carbon, nitrogen and phosphorus to the environment. Therefore, the slight change of decomposition rate will cause changes of carbon and nitrogen pool in wetland, and even lead to eutrophication, and then affect the germination, growth and distribution pattern of plants in wetland in the coming year (Xiaolong et al. Citation2014), which affects the habitat quality of wintering waterbirds. For this investigation, the decomposition bag technology and the groundwater level gradient formed naturally by the Poyang Lake wetland were used to simulate the decomposition process of Carex. cinerascens Kükenth under different groundwater level gradients. The purpose of this study was to investigate the differentiation of carbon, nitrogen and phosphorus along the gradient of underwater level during the decomposition of Carex. cinerascens Kükenth litter in Poyang Lake Wetland during the dry season.
2. Materials and methods
2.1. Site description
The research area was in the dish lake (Baisha Lake) of Nanji Wetland National Nature Reserve of Poyang Lake wetland. The reserve is in the southern part of the main lake area of Poyang Lake, on the alluvial delta front formed by merging of the north, the middle and the south branches of the Gan Jiang River merge into the open waters of Poyang Lake. The altitude is between 12 and 16 m (Wusong), and the area is characterized by a subtropical warm and wet monsoon climate with hot and rainy summer and low temperature = with little rain during winter (Committee "Poyang Lake Research" editorial Citation1988).
The study area has an average annual rainfall of 1358–1823 mm, most of which occurs from April to June. Additionally, the mean temperature of the coldest month (January) was 4.5 °C, while that of the hottest month (July) was 29.1 °C, and the annual average temperature was 17.3 °C. There are obvious flood seasons and dry seasons in the reserve area, with the entire area except for Nanshan Island and Jishan Island (total area ≤ 4 km2) being flooded during the wet season (April to September) and in a typical lacustrine hydrological state. However, during the dry season (October to March of the following year), the lake water recedes into river courses and some dish-shaped depressions, after which different elevation beaches emerge one after another and large and small rivers and dish lakes were scattered in the area. The entire delta area presents an interlaced landscape of rivers, lakes and continents.
The unique hydrological rhythm, which alternates periodically from high to low water season year after year, leads to the formation of a large amount of fertile soil, abundant hydrothermal conditions, and the development of wet and aquatic plants with very complex floristic composition and extremely high productivity. The dominant wetland plant species in the region are Carex. cinerascens Kükenth, Triarrhena lutarioriparia, and Triarrhena lutarioriparia and so on (Wang et al. Citation2013).
2.2. Field experiment design and samples analysis method
In November 2016, Baisha Lake was selected for establishment of fixed sample plots (). This lake is subjected to few anthropogenic disturbances, contains well developed plant communities, and has beaches that differ significantly in elevation. More than 90% of the plots in the study area were covered by Carex. cinerascens Kükenth and there were scattered patches of Triarrhena lutarioriparia, and Triarrhena lutarioriparia. According to variations in elevation of the dish lake beach, four gradients transect belts covering four gradients from the lake shore to the center of the lake were selected. The interval between each adjacent sample belt was about 100 m. Specifically, the G-L gradient was close to the shore of the lake, had the longest exposure time, had a groundwater depth that fluctuated between 25 and 50 cm; The G-ML gradient was located in the middle part of grass beach, and had a relatively long time of emergence. The depth of groundwater fluctuates between 15 and 25 cm in the G-ML; The G-MH gradient was also in the middle part of grass beach but had a relatively short time of emergence. The groundwater depth in the G-MH fluctuated between 5 and 15 cm; The G-H gradient, which was close to the water body in the center of the lake, had a groundwater depth that fluctuates between −5 and 5 cm. There were six sample plots in each gradient zone (). Determination of the groundwater level in each sample plot was conducted using buried the Pipe method (Grootjans et al. Citation1988).
Additionally, soil samples were collected in each sample plot by a 50-mm diameter stainless steel soil augur. Samples were collected to a depth of 20 cm from five subplots near each sample plots and mixed into a composite sample, and then packed into the polyethylene self-sealing bag. After numbering, samples were placed into a storage box and transported back to the laboratory. Upon arrival in the laboratory, debris such as stones were picked out, part of samples were air-dried and then ground to fine powder for measuring soil organic carbon (SOC), total nitrogen (TN) and total phosphorus (TP), and part of samples were kept fresh for measuring soil moisture, soil microbial biomass carbon (MBC) and microbial biomass nitrogen (MBN).
The decomposition bags were made of white nylon Nytex mesh bags (pore size 0.15 mm, sized 15 cm × 20 cm). This kind of net bag can not only avoid the non-decomposition loss of decomposing residue in the decomposing bag, but also ensures that the decomposition function is not limited (Zhang et al. Citation2018). The leaves of the old Carex. cinerascens Kükenth in a small area of grass near the shore of the lake were harvested. The harvested plants were washed with deionized water in the laboratory, then cut into 10 cm long sections and mixed evenly (to eliminate the effect of size on decomposition) in a Kraft paper envelope. All of the envelopes were placed in the oven at temperature of 120 °C for 1 hour, and then changed the temperature to 60 °C for 72 hours. After taking a part of dried Carex. cinerascens Kükenth sample to measure its initial nutrient content, the remaining sample was weighed 5.00 g and placed into each of the decomposition bag. A total of 150 decomposition bags were subsequently taken to the sample plots and fixed with bamboo poles in 15 preset sample plots, with 10 samples placed for each sample plot. Each decomposition bag was fully exposed to the surface of the ground existing leaves litter and in full contact with the surface. When water accumulated on the surface, the decomposition bag was fixed by the bamboo poles making sure the bags were still close to the ground surface. The decomposition bags were retrieved from various points on day 15, 30, 60, 90, 120 and 150 respectively of the experiment. Upon retrieval, samples were returned to the laboratory, where the dirt was removed, after which the Carex. cinerascens Kükenth and other debris were rinsed with deionized water and dried in a 60 °C oven to constant weight, weigh the residual dry matter, and use the ball milling instrument. The sample was then ground to a powder with a particle size of 0.06 μm and then sealed in a numbered polyethylene sample bag until subsequent analysis.
The methods used for analysis of samples in decomposition bags analysis were as follows: Total carbon (TC) and total nitrogen (TN) content were measured using an element analyzer (Vario Max CN Analyzer, Elementar Analysensysteme GmbH, Germany) Total phosphorus (TP) content was measured using an Inductively Coupled Plasma Optical Emission Spectrometer (ICP-OES) (Optima 5300 DV, Perkin-Elmer, America)
Soil moisture content was determined by the oven drying method (weighing before and after drying at 105 °C for 72 h); Soil particle fractions were analyzed with a Longbench Mastersizer 2000 instrument (Malvern Instruments, Malvern, England).Soil pH was determined using a pH meter (Sartorius, Germany). SOC and TN were determined using an element analyzer (Elementar, Vario Max CN). TP was determined by ashing followed by an automated colorimetric procedure (United States Environmental Protection Agency 1993, Method 365.1). MBC and MBN were determined by chloroform fumigation K2SO4 extraction method.
2.3. Data processing and statistical analysis
The percentage of litter dry weight loss was calculated using the following equation (Zhao et al. Citation2015):
where Lt is the percentage of litter dry weight loss at time t, Mt is the dry matter weight of the litter at the time t, and M0 is the initial dry matter weight.
The instantaneous decay rate of litter dry mass() can be calculated using the Olson negative exponential attenuation model (Olson Citation1963):
where k is the instantaneous decay rate of litter at time t, with a higher value associated with a faster decomposition rate.
The dry matter residual rate(R) can be simulated using negative exponential attenuation model (orthogonal distance regression) (Wider and Lang Citation1982):
where R is the residual rate of litter, a is the fitting parameter and e is the natural base number.
The relative return index (RRI) of C, N or P of plant litter is given by (Zhao et al. Citation2015):
where Ct is the concentration of an element in the litter at time t and C0 is the initial concentration of an element in litter. The CRRI is the carbon relative return index, NRRI is the nitrogen relative return index and PRRI is the phosphorus relative return index. A positive RRI indicates that the element has a net release during the decomposition of the litter, while a negative RRI indicates that the net accumulation has occurred.
The effects of different groundwater levels on the release rate of carbon, nitrogen and phosphorus in the process of decomposition were analyzed in Excel2016 and Origin9.0 at the significant level of α = 0.05, and one-way analysis of variance (ANOVA) and LSD in spss19.0 software. In R v3.4.0 (R Development Core Team, 2013), redundancy analysis (RDA) was used to study the relationship between carbon, nitrogen and phosphorus release characteristics and soil environmental factors in the decomposition process of Carex. cinerascens Kükenth under the environmental gradient of different groundwater level on the 60th day of decomposition.
3. Results and analysis
3.1. Decomposition process of Carex. cinerascens Kükenth litter
Calculation of the dry weight loss rate, Lt. The results showed that the decomposition time had a significant effect on the dry matter loss rate of the Carex. cinerascens Kükenth litter (F = 22.056, p < 0.0001), and that the dry matter loss rate of the four groundwater level gradients gradually increased with the number of decomposition days ().
Figure 2. Variations in the percentage of litter dry mass loss (a) and decay rate (b) during Carex decomposition along water table gradients.
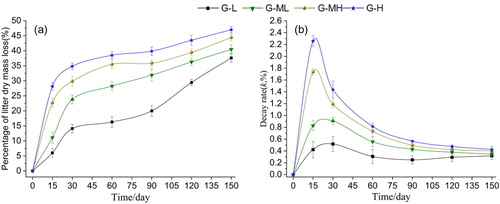
From day 15 to 120, the difference between the four gradients was significant (15d: F = 8.451, p = 0.001;30d: F = 17.015, p < 0.0001;60d: F = 15.85, p < 0.0001;90d: F = 33.563, p < 0.0001;120d: F = 7.147, p = 0.002).On day 150 after the decomposition, the difference between the four gradients began to be inconspicuous (F = 2.401, p = 0.098). With the groundwater level rising from high to low, the dry matter loss rate decreased gradually, and after 150 days, G-L was 47.01%, G-ML was 44.38%, G-MH was 40.07% and G-H was 37.62%, respectively ().
The instantaneous attenuation coefficient (decay rate, k) of each decomposition time point was calculated using the Olson negative exponential decay model. The results showed that the decomposition rate of Carex. cinerascens Kükenth was significant at different decomposition time points (F = 332.29, p < 0.0001). The instantaneous attenuation coefficients of the dry grasses in the four groundwater level gradients showed a rapid increase and then decreased slowly until stabilizing (). The maximum k-values of the G-H and G-MH gradients, which occurred on day 15, were 2.258 and 1.737, respectively. However, the maximum k-value of the G-ML and G-L gradients appeared on day 30, when their maximum values were 0.907 and 0.518, respectively.
During day 15 to 120, the difference in k-values between the four gradients was significant (15d: F = 8.176, p = 0.01;30d: F = 16.522, p < 0.0001;60d: F = 15.105, p < 0.0001; 90d: F = 34.087, p < 0.0001;120d: F = 6.985, p = 0.002). On the day 150 after the decomposition, the difference in k-values between the four gradients was not significant (F = 2.356, p = 0.102). In addition, the k-values increased as the increase of water level at each decomposition time point ().
The k-value calculated by the Olson model was simulated by the orthogonal distance regression of the negative exponential decay model. The results revealed that the fitting effect was very good, whit R2 values was all above 0.97 (p < 0.0001). The parameters of the fitting equation are shown in . Based on the equation, the predicted time required for 50% decomposition of litter in the four gradients of G-L, G-ML, G-MH and G-H is approximately 247 days, 205 days, 197 days and 166 days, respectively, while that required for 95% is approximately is 1066 days, 866 days, 851 days and 718 days. The decomposition rates of the four gradients were significantly different, occurring in the order G-H > G-MH > G-ML > G-L ().
Table 1. Fitting parameters of Olson decay model for Carex decomposition along water table gradients.
3.2. Relative return index of carbon, nitrogen and phosphorus dynamic of Carex. cinerascens Kükenth litter
The four gradients of carbon elements throughout the decomposition process are shown as net release mode (CRRI > 0). The CRRI values of the four gradients differed significantly from day 15 to day 120 (15 d: F = 11.264, p < 0.0001; 30 d: F = 20.250, p < 0.0001; 60 d: F = 21.974, p < 0.0001; 90 d: F = 46.069, p < 0.0001; 120 d: F = 18.212, p = 0.002). After 150 days, the difference decreased, but there was still a significant difference (F = 5.721, p = 0.005) and the CRRI value increased with the increase in groundwater level ().
Figure 3. Variations of CRRI(a), NRRI(b), PRRI(c) during Carex decomposition along water table gradients.
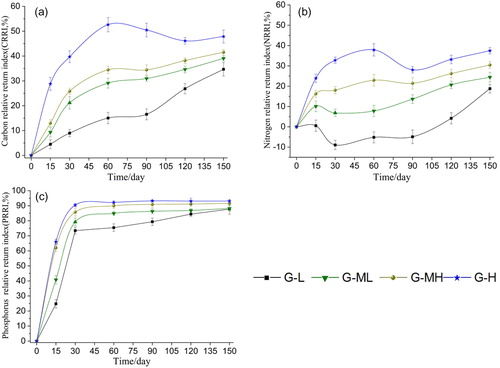
As shown in , the trend in NRRI and CRRI differed. During the decomposition process, the nitrogen element of G-L showed a pattern of initial net accumulation (NRRI < 0) and then net release (NRRI > 0). However, G-ML, G-MH and G-H were always showed a net release of nitrogen. Before 120 days of decomposition, the four gradient NRRI values differed significantly (15d: F = 6.262, p = 0.004; 30d: F = 18.604, p < 0.0001; 60d: F = 10.593, p < 0.0001; 90d: F = 11.833, p < 0.0001; 120d: F = 9.115, p = 0.001), but this difference decreased after 120 days (150d: F = 3.004, p = 0.055). As the groundwater level rose, the NRRIs increased ().
The variation trend in PRRI in the four gradient bands was consistent () and increased rapidly within 30 days, after which it stabilized. The phosphorus elements in the four gradients showed a net release pattern (PRRI > 0) throughout the whole decomposition process. There was a very significant difference in PRRI among the four gradients during the 90 days in the early stage of decomposition (15d: F = 30.503, p < 0.0001; 30d: F = 38.098, p < 0.0001; 60d: F = 95.445, p < 0.0001; 90d: F = 74.611, p < 0.0001). However, there was no significant difference among the four gradients at 120 and 150 days in the late stage of decomposition (120d: F = 3.466, p = 0.036; 150d: F = 4.150, p < 0.019). During each decomposition stage, the minimum degradation occurred in the G-L gradient, followed by G-ML and GMH, while the maximum was in the G-H gradient ().
3.3. Carbon, nitrogen and phosphorus stoichiometry dynamic of Carex. cinerascens Kükenth litter
The ratio of C/N in the four gradients decreased first, then increased with decomposition time, with a range of about 19.37–13.32 (). The C/N ratio did not differ significantly among the four gradients throughout the decomposition process (p > 0.005), and it remained relatively stable.
Figure 4. Variations of C, N and P stoichiometric ratio during Carex decomposition along water table gradients.
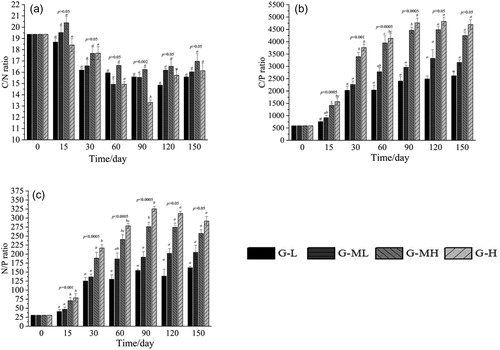
The C/P ratio of the four gradients was significantly different from day 15 to 120 (15d: F = 10.791, p < 0.0001; 30d: F = 8.771, p < 0.0001; 60d: F = 12.613, p < 0.0001; 90d: F = 13.275, p < 0.0001). However, there were no significant differences among the four gradients at day 120 and 150 (120d: F = 1.118, p = 0.365; 150d: F = 1.408, p = 0.270). The C/P ratio increased as the groundwater level gradient increased ().
From day 15 to 90, the difference in the N/P ratio between the four gradients was significant (15d: F = 7.866, p = 0.001; 30d: F = 12.034, p < 0.0001; 60d: F = 12.506, p < 0.0001; 90d: F = 19.080, p < 0.0001). From day 120 and 150, the difference between the four gradients began to be inconspicuous (120d: F = 1.127, p = 0.362; 150d: F = 0.896, p = 0.461). Overall, the lowest N/P ratio was observed in the G-L gradient, followed by G-ML and G-MH, and the maximum was observed in the G-H gradient ().
3.4. Response of carbon, nitrogen and phosphorus release to soil environment
In addition to soil moisture and total phosphorus content, other soil properties such as soil pH, bulk density, sand, silt and clay content, organic matter and total nitrogen, microbial biomass carbon and nitrogen content were significantly different in the four gradients (). As the groundwater levels increased, the pH value and sand content of the soil increased significantly (p < 0.001), while the bulk density, silt and clay content, organic matter, total nitrogen and phosphorus and microbial biomass carbon and nitrogen content decreased significantly (p < 0.001).
Table 2. Descriptive statistics of soil physical, chemical and microbial indicators along the water table gradient.
Upon redundancy analysis of the parameters (C/N, C:P, N/P, CRRI, NRRI, PRRI, k) to determine the carbon, nitrogen and phosphorus release characteristics during all decomposition processes, the cumulative value of the first two sorting axes was 99.97%, of which the first sorting axis explained 99.91% of the variation (). Monte Carlo testing revealed that, among all soil environmental factors, the release of carbon, nitrogen and phosphorus during decomposition was significantly correlated with soil pH, bulk density, soil total nitrogen, soil organic matter, microbial biomass carbon and soil sand content (p < 0.05). Additionally, pH, bulk density and soil total nitrogen had the strongest correlation with the first sorting axis, which was the main reason for the differentiation of carbon, nitrogen and phosphorus release characteristics during the decomposition of different groundwater level gradients. The relative return index of carbon was positively correlated with soil pH value, but it was negatively correlated with soil bulk density, soil nitrogen content and microbial biomass nitrogen, and soil organic matter. The relative return index of nitrogen and phosphorus was also affected by soil moisture content and sand content. In the process of carbon, nitrogen and phosphorus release, the correlation between C/N ratio and soil environmental factors was not strong, and the spatial differentiation was not obvious.
Figure 5. Redundancy analysis (RDA) of the characteristic parameters of carbon, nitrogen and phosphorus release during Carex decomposition along water table gradients. SOM:soil organic matter, STN:soil total nitrogen, STP:soil total phosphorus, MBC:soil microbial carbon, MBN:soil microbial nitrogen, BD: soil bulk density, Moisture: soil moisture content, sand:soil sand content, silt:soil silt content, clay:soil clay content, CN:litter C/N ratio, CP:litter C/P ratio, NP:litter N/P ratio, K:decay rate, namely k.
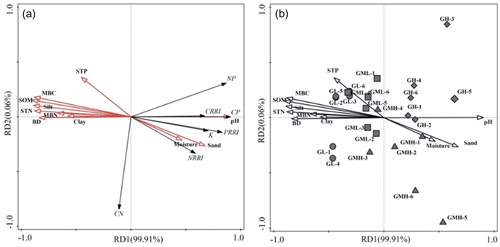
4. Discussion
4.1. Influence of groundwater level gradients on decomposition process of Carex. cinerascens Kükenth litter
The results of this study revealed that the dry matter loss rate and decomposition rate of Carex. cinerascens Kükenth were significantly different in different decomposition time stages (p < 0.001). The loss rate of dry matter increased as the decomposition time increased, and the value of the decomposition rate increased rapidly to the maximum within 15–30 days of decomposition, after which it slowly decreased until it became stable. This may have been because there is more water in the wetland environment than in the terrestrial environment, and the soluble components in the litter of Carex. cinerascens Kükenth in the early stage of decomposition are rapidly leached, the water-soluble components and non-lignin carbohydrates are preferentially decomposed, and the decomposition rate is very fast (Davis et al. Citation2003).
However, in the middle and late stages of decomposition, the lignified undecomposed carbohydrates and the original lignin degraded at the same time under microbial action, and the process was relatively slow, until the content of lignin in the last litter tended to stabilized, and the residual matter gradually decayed. At this point, the decomposition rate was almost zero (Kalbitz et al. Citation2006). Zhang et al. found that cellulose and lignin in the litter of Carex. cinerascens Kükenth would quickly decompose in the first 60 days in Poyang Lake wetland (Zhang et al. Citation2018), and that the decomposition rate during the early stage of decomposition of this study might be related to this.
The results also showed that the gradients of groundwater level have a great influence on the decomposition process of litter in Poyang Lake wetland, with the dry matter loss rate and decomposition rate both differing significantly among the four gradients of groundwater level. The values of G-H > G-MH > G-ML > G-L are all shown for the total decomposition process, and the values of both were much smaller in the G-L gradients than in other gradients. Similar results were obtained by the model simulation (). These findings indicate that the high groundwater level or the environmental conditions of water accumulation are more conducive to the decomposition of litter, which were consistent with the results reported by Sun et al. for Calamagrostis angustifolia (Sun et al. Citation2012) and Hou et al. for the litter of Carex lasiocapa (Hou et al. Citation2012). Water conditions of the wetland decomposing environment have a great influence on the leaching process and microbial degradation process of litter. The groundwater level indirectly affects the mineralization and decomposition rate of organic matter by influencing soil moisture status, aeration status, dissolved oxygen content in soil water and microbial activity (Peltoniemi et al. Citation2012). The lower water level or shallow water accumulation can maintain sufficient moisture and moisture on the soil surface, and the long-term repeated infiltration of litter can accelerate the leaching loss process of soluble matter in litter. Under low groundwater conditions, the surface water is lack of water scour, so the process of leaching loss is slower (Neckles and Neill Citation1994).
Another reason for faster decomposition of litter under high groundwater level or stagnant water environment in this study may be that a large number of microbial decomposers play an important role in the process of decomposition (Keiluweit et al. Citation2015). Because the groundwater level gradient also has a great impact on microbes (Zhang et al. Citation2018). Zhang et al. found that the gradients of groundwater level in Poyang Lake wetland is an important factor limiting the composition and activity of the microbial community during the dry season, and the surface soil water content increases significantly as the groundwater level increases. Specifically, soil microbial biomass, bacterial biomass, actinomycetes biomass, Gram-positive bacteria and Gram-negative bacterial biomass all increased significantly as the groundwater level increased (Zhang et al. Citation2018). Proper water condition can improve the nutrient utilization efficiency of microorganisms in litter, as well as enhance the microbial colonization ability and metabolic rate of decomposers (Neckles and Neill Citation1994; Molles et al. Citation1995), which subsequently promotes decomposition. Studies have shown that there is a significant positive correlation between the respiration rate of microorganisms and the availability of water (Kuehn and Suberkropp Citation1998). However, if there is insufficient water in the decomposing environment, the bioactivity of the decomposer will be reduced, and the decomposition rate will be slow (Valk et al. Citation1991). Because microbes degrade refractory substances in litter, microbial activity can produce protease, cellulase and lignin degrading enzymes, and increase the decomposition rate of litter. In most wetlands, bacteria control the decomposition rate of litter. The amount of decomposition action accounted for 90% of the total loss of litter (Barik et al. Citation2000). However, this study only evaluated the dry season of Poyang Lake, and the effects of duration and frequency of flooding on the decomposition of litter need to be further investigated.
Acidity and alkalinity in the decomposition environment are also important factors influencing litter decomposition because microbes all have different suitable ranges of pH, higher or lower pH values may significantly inhibit microbial activity (Grosso et al. Citation2016), thus affecting the decomposition process. Hohmann and Neely found that increased acidity could inhibit the decomposition of Sparganium eurycarpum litter (Hohmann and Neely Citation1993), while Leuven and Wolfs found that pH had a more important impact on decomposition of Juncus bulbosus L., with the dry weight loss of total organic matter being 55 and 59% after 37 days in the control treatment with a pH value of 3.5 and 5.6, respectively (Leuven and Wolfs Citation1988). In fact, the groundwater level gradient had a great influence on the acidity and alkalinity of the decomposing environment (Zhang et al. Citation2018). The results of the present study showed that, as the groundwater level rose, soil pH values also increased significantly in the decomposition environment (), and there was a significant positive correlation between pH value and decomposition rate (), which indicated that acidity in the decomposition environment had a great influence on the decomposition of litter (Andersson and Ingvar Citation2001). The relatively high gradient zone of groundwater level has higher soil water content and higher sand content in dry season, which further increases soil pH, weakens soil acidity and increases soil permeability (Zhang et al. Citation2018). These create a suitable micro-ecological environment for soil microbial metabolism and promote plant decomposition (Leuven and Wolfs Citation1988).
4.2. Effects of groundwater table gradient on the dynamics of carbon, phosphorus and phosphorus release from the decomposition of Carex. cinerascens Kükenth litter
The results showed that groundwater had a significant effect on the release dynamics of carbon, nitrogen and phosphorus during decomposition of the litter. The relative return indexes (RRIs) of carbon, nitrogen and phosphorus were G-H > G-MH > G-ML > G-L throughout the decomposition process. These findings suggest that a high groundwater level or a small amount of water accumulation can promote nutrient release. At four water level gradients, carbon and phosphorus showed net release in all stages of decomposition. However, a net release of nitrogen occurred in the G-ML, G-MH and G-H gradients, whereas it accumulated first and was then released in the G-L gradient. Moreover, the dry matter loss rate of the four groundwater level gradients was significantly positively correlated with the relative nutrient return index (p < 0.0001). Taken together, these findings indicate that the absolute return of these four gradient nutrients depends to a large extent on the amount of dry matter lost (). These results are similar to those reported by Sun et al. for the Sanjiang Plain wetland (Sun et al. Citation2012) and Zhao et al. for the Yellow River Delta wetland in China (Zhao et al. Citation2015).
In general, the overall change in the carbon return index consists of two processes: a rapid increase and a slow increase (Zhao et al. Citation2015). During the process of leaching, the dissolved carbohydrates are rapidly leached, the carbon return index will increase rapidly, and then the decomposition rate will be accelerated in response to stimulation by external environmental factors or the input of exogenous nutrients. The amount and vitality of soil microorganisms increased, and the residual lignin, cellulose and tannins were gradually used by microbes, after which the carbon return index rate of increase began to slow or even decrease (Davis et al. Citation2003).
For the nitrogen return index in this study, the entire decomposition process does not show an increasing trend but increases and decreases. Nevertheless, the overall performance is a net release or net accumulation. Although nitrogen decreases rapidly because of the loss of nitrogen-containing salts during leaching, it is more likely to be present in complex, less leached macromolecular proteins, and its decomposition is mainly affected by microorganisms. The increased of microbial demand for nitrogen during the decomposition of these macromolecular proteins will lead to nitrogen fixation, and the increase in the nitrogen return index will slow down or even decrease significantly (Wu Xian and Yang Citation2007), because microbial nitrogen fixation is the main reason for nitrogen accumulation during decomposition (Aber and Melillo Citation1980).
The change in phosphorus return is affected by both physical and biological factors. The leaching of phosphorus in the early stages of decomposition was relatively strong, and the rate of phosphorus return in the decomposition residue was very fast. When phosphorus is released to a certain extent, the rate of phosphorus return tends to be stable (Wrubleski et al. Citation1997). This may be because phosphorus in plant tissues is mainly in the form of phosphate or compounds and is prone to leaching loss. During microbial degradation in the later stages of decomposition, when the nutrient requirements of microbes are not limited by phosphorus, excessive phosphorus will limit the microbial activity and phosphorus release will occur (Sun et al. Citation2012).
In this study, the decomposition rates of G-MH and G-H gradients were significantly correlated with C/P and N/P (), which showed that the accumulation or release of carbon, nitrogen, phosphorus and other nutrients in the residual litter changes with the decomposition of litter, as does the stoichiometric ratio will also change, and these changes will affect the decomposition process in the next stage (Manzoni et al. Citation2010). This also confirms that under certain conditions, the stoichiometric ratio of carbon, nitrogen and phosphorus is the best indicator to predict the decomposition rate. This is because they reflect the ratio of carbohydrate to protein in the litter (Chen Citation1999), and the decomposition of carbon nitrogen, and phosphorus nutrition in the environment may also affect the decomposition process of litter (Sun et al. Citation2012). Xie et al. studied the effects of nitrogen and phosphorus availability on the decomposition of aquatic plants and found that the increase of phosphorus availability increased the decomposition rate of Hyacinth by 68–87%, but the effects of nitrogen utilization efficiency were not significant. These findings show that the response of the decomposition rate to nutrient availability depends on the type of nutrient (Xie et al. Citation2004). Zhang et al. found that there were very significant differences in carbon, nitrogen and phosphorus in soil environment under different groundwater level gradients during the dry season in Poyang Lake wetland (Zhang et al. Citation2018), which made the different availability of carbon, nitrogen and phosphorus under different ground water level conditions. This may also be an important reason for the differences in nutrient element return observed during litter decomposition of Carex. cinerascens Kükenth.
5. Conclusions
Simulated decomposition, experiments of Carex. cinerascens Kükenth litter under different water table conditions in the dry season of Poyang Lake wetland revealed the following.
The groundwater gradient during the dry season of Poyang Lake wetland is a key factor influencing the decomposition process of litter and the return of carbon, nitrogen and phosphorus. A higher groundwater level, especially when there are alternating shallow and high groundwater levels, can promote the decomposition of litter and return of carbon, nitrogen and phosphorus.
The Olson negative exponential decay model predicted that the time required for 50% decomposition in the G-H, G-MH, G-ML and G-L gradients was about 166, 197, 205 and 247 days, respectively, while about 1.97, 2.23, 2.43 and 2.92 years were required for 95% decomposition.
Under different groundwater level gradients, the difference in C/N in litter was not significant, but there was a significant difference between C/P and N/P at certain decomposition stages.
Soil pH, bulk density, soil total nitrogen, soil organic matter, microbial biomass carbon and soil sand content had significant effects on litter decomposition and nutrient release. The relative return index of carbon was positively correlated with soil pH but negatively correlated with soil bulk density, soil nitrogen content, microbial biomass nitrogen and soil organic matter.
Overall, firstly, the current study lends support for optimizing groundwater level management strategies to minimize litter decomposition rates and the mineralization of carbon and nitrogen to prevent C,N release from the system. Secondly, in this study, it is found that plant decomposition is sensitive to changes of environmental water conditions at sample scale. Therefore, under the background of regional and global scale, the process of plant decomposition may have more significant response to global climate change, especially the change of precipitation pattern, which needs to be further studied.
Acknowledgments
We thank the Nanji Wetland National Nature Reserve Agency for cooperation and support with the field work and sampling conducted in this study. We also appreciate Meng Zhu Jian, a graduate student of Nanchang University, and Rao Didi, a graduate student from Jiangxi Normal University, for their help setting up and sampling in the field. We thank Jeremy Kamen, MSc for editing the English text of a draft of this manuscript.
Additional information
Funding
Notes on contributors
Quanjun Zhang
Quanjun Zhang is currently a PhD candidate for research on wetland monitoring and biogeochemistry at Key Laboratory of Ecosystem Network Observation and Modeling, Institute of Geographic Sciences and Natural Resources Research, Chinese Academy of Sciences.
Guangshuai Zhang
Guangshuai Zhang is currently a research assistant of National Marine Environmental Monitoring Center, People's Republic of China, and a PhD of Institute of Geographic Sciences and Natural Resources Research, Chinese Academy of Sciences.
Xiubo Yu
Xiubo Yu is the research program leader in Poyang Lake wetland ecology, and a researcher of Key Laboratory of Ecosystem Network Observation and Modeling, Institute of Geographic Sciences and Natural Resources Research, Chinese Academy of Sciences. He is currently a coordinator of Ecosystem Monitoring and Capacity Building at UNEP International Ecosystem Management Partnership.
Yu Liu
Yu Liu is an associate research fellow of Key Laboratory of Ecosystem Network Observation and Modeling, Institute of Geographic Sciences and Natural Resources Research, Chinese Academy of Sciences and specialized in the trade-off of wetland ecological service.
Shaoxia Xia
Shaoxia Xia is currently a research assistant of Key Laboratory of Ecosystem Network Observation and Modeling, Institute of Geographic Sciences and Natural Resources Research, Chinese Academy of Sciences and specialized in wetland ecology and ecological service.
Li Ya
Li Ya is currently a PhD of Institute of Geographic Sciences and Natural Resources Research, Chinese Academy of Sciences.
Binhua Hu
Binhua Hu is currently a director general of Poyang Lake Nanji Wetland National Nature Reserve Agency, People's Republic of China.
Songxian Wan
Songxian Wan is currently a staff of Poyang Lake Nanji Wetland National Nature Reserve Agency, People's Republic of China.
References
- Aber JD, Melillo JM. 1980. Litter decomposition: measuring relative contributions of organic matter and nitrogen to forest soils. Can J Bot. 58(4):416–421.
- Alexandra S, Richards JH, Scinto LJ. 2013. Plant decomposition in wetlands: effects of hydrologic variation in a re-created everglade. J Environ Qual. 42(2):562–572.
- Anderson JT, Smith LM. 2002. The effect of flooding regimes on decomposition of Polygonum pensylvanicum in playa wetlands (Southern Great Plains, USA). Aquat Bot. 74(2):97–108.
- Andersson S, Ingvar SN 2001. Influence of pH and temperature on microbial activity, substrate availability of soil-solution bacteria and leaching of dissolved organic carbon in a mor humus. Soil Biol Biochem. 33(9):1181–1191.
- Barik SK, Mishra S, Ayyappan S. 2000. Decomposition patterns of unprocessed and processed lignocellulosics in a freshwater fish pond. Aquat Ecol. 34(2):185–204.
- Chapin FS III, Matson PA, Vitousek PM, Mooney HA. 2002. Principles of Terrestrial Ecosystem Ecology. New York: Springer.
- Chen H. 1999. Root decomposition in three coniferous forests: effects of substrate quality, temperature, and moisture. United States: Oregon State University.
- Chimney MJ, Pietro KC. 2006. Decomposition of macrophyte litter in a subtropical constructed wetland in South Florida (USA). Ecol Eng. 27(4):301–321.
- Committee "Poyang Lake Research" editorial. 1988. Poyang Lake Research (in Chinese). Shanghai: Shanghai Science and Technology Publishing House.
- Currey P, Johnson D, Sheppard L, Leith I, Toberman H, Van Der Wal R, Dawson L, Artz R. 2009. Turnover of labile and recalcitrant soil carbon differ in response to nitrate and ammonium deposition in an ombrotrophic peatland. Global Change Biol. 16(8):2307–2321.
- Dai He X, Rong-Rong ZW. 2017. Variation of seasonal water-level fluctuations in riverconnected lakes in the middle reaches of Yangtze river in the recent three decades (in Chinese). Resour Environ Yangtze Basin. 26(1):118–125.
- Davis SE, Corronado-Molina C, Childers DL, Day JW. 2003. Temporally dependent C, N, and P dynamics associated with the decay of Rhizophora mangle L. Leaf litter in oligotrophic mangrove wetlands of the southern everglades. Aquat Bot. 75(3):199–215.
- Day FP. 1983. Effects of flooding on leaf litter decomposition in microcosms. Oecologia. 56(2-3):180–184.
- Ferreira V, Chauvet E. 2011. Synergistic effects of water temperature and dissolved nutrients on litter decomposition and associated fungi. Global Change Biol. 17(1):551–564.
- Grootjans AP, Diggelen R, Wassen MJ, Wiersinga WA. 1988. The effects of drainage on groundwater quality and plant species distribution in stream valley meadows. Vegetatio. 75(1-2):37.
- Grosso F, Bååth E, De Nicola F. 2016. Bacterial and fungal growth on different plant litter in Mediterranean soils: Effects of C/N ratio and soil pH. Appl Soil Ecol. 108:1–7.
- Guan L, Wen L, Feng D, Zhang H, Lei G. 2014. Delayed flood recession in central Yangtze floodplains can cause significant food shortages for wintering geese: results of inundation experiment. Environ Manage. 54(6):1331–1341.
- Hohmann J, Neely RK. 1993. Decomposition of Sparganium eurycarpum under controlled pH and nitrogen regimes. Aquat Bot. 46(1):17–33.
- Hou C, Song C, Li Y, Guo Y, Yang G. 2012. Litter decomposition and nutrient dynamics of Carex Lasiocapa under different water conditions (in Chinese). Acta Ecol Sin. 32(2):650–658.
- Kalbitz K, Kaiser K, Bargholz J, Dardenne P. 2006. Lignin degradation controls the production of dissolved organic matter in decomposing foliar litter. Eur J Soil Sci. 57(4):504–516.
- Keiluweit M, Bougoure JJ, Nico PS, Pett-Ridge J, Weber PK, Kleber M. 2015. Mineral protection of soil carbon counteracted by root exudates. Nat Clim Change. 5(6):588–595.
- Kuehn KA, Suberkropp K. 1998. Diel fluctuations in rates of CO2 evolution from standing dead leaf litter of the emergent macrophyte Juncus Effusus. Aquat Microb Ecol. 14(2):171–182.
- Laiho R, Laine J, Trettin CC. 2004. Scots pine litter decomposition along drainage succession and soil nutrient gradients in peatland forests, and the effects of inter-annual weather variation. Soil Biol Biochem. 36(7):1095–1109.
- Langhans SD, Tockner K. 2006. The role of timing, duration, and frequency of inundation in controlling leaf litter decomposition in a river-floodplain ecosystem. Oecologia. 147(3):501–509.
- Lei Mark C, Gang BL. 2008. New anatidae population estimates for Eastern China: implications for current flyway estimates. Biol Conserv. 141(9):2301–2309.
- Leuven RSEW, Wolfs WJ. 1988. Effects of water acidification on the decomposition of Juncus bulbosus L. Aquat Bot. 31(1-2):57–81.
- Manzoni S, Trofymow JA, Jackson RB, Porporato A. 2010. Stoichiometric controls on carbon, nitrogen, and phosphorus dynamics in decomposing litter. Ecol Monogr. 80(1):89–106.
- Mitsch WJ, Gosselink JG. 2015. Wetlands, 5th ed. Hoboken, NJ: Wiley.
- Molles M-C, Crawford C-S, Ellis L-M. 1995. Effects of an experimental flood on litter dynamics in the middle Rio Grande riparian ecosystem. Regul Rivers Res Manag. 11(3):275–281.
- Mueller P, Schile-Beers LM, Mozdzer TJ, Chmura GL, Dinter T, Kuzyakov Y, de Groot AV, Esselink P, Smit C, D’Alpaos A. 2018. Global-change effects on early-stage decomposition processes in tidal wetlands – implications from a global survey using standardized litter. Biogeosciences. 15(10):3189–3202.
- Neckles HA, Neill C. 1994. Hydrologic control of litter decomposition in seasonally flooded prairie marshes. Hydrobiologia. 286(3):155–165.
- Olson JS. 1963. Energy storage and the balance of producers and decomposers in ecological systems. Ecology. 44(2):322–331.
- Peltoniemi K, Straková P, Fritze H, Iráizoz PA, Pennanen T, Laiho R. 2012. How water-level drawdown modifies litter-decomposing fungal and actinobacterial communities in Boreal Peatlands. Soil Biol Biochem. 51(3):20–34.
- Rejmánková E, Houdková K. 2006. Wetland plant decomposition under different nutrient conditions: what is more important, litter quality or site quality?. Biogeochemistry. 80(3):245–262.
- Suberkropp K. 1995. The influence of nutrients on fungal growth, productivity, and sporulation during leaf breakdown in streams. Can J Bot. 73(73:1361–1369.
- Sun Z, Mou X, Liu JS. 2012. Effects of flooding regimes on the decomposition and nutrient dynamics of Calamagrostis angustifolia litter in the Sanjiang Plain of China. Environ Earth Sci. 66(8):2235.
- Tupacz EG, Day FP. 1990. Decomposition of roots in a seasonally flooded swamp ecosystem. Aquat Bot. 37(3):199–214.
- Valk AG, Rhymer JM, Murkin HR. 1991. Flooding and the decomposition of litter of four emergent plant species in a prairie wetland. Wetlands. 11(1):1–16.
- Wang Y, Yu X, Zhang L, Lei G. 2013. Seasonal variability in baseline δ15n and usage as a nutrient indicator in Lake Poyang, China. J Freshwater Ecol. 28(3):365–373.
- Wider RK, Lang G-E. 1982. A critique of the analytical methods used in examining decomposition data obtained from litter bags. Ecology. 63(6):1636.
- Wrubleski DA, Murkin HR, van der Valk AG, Nelson JW. 1997. Decomposition of emergent Macrophyte Roots and Rhizomes in a Northern Prairie Marsh. Aquat Bot. 58(2):121–134.
- Wu S, He S, Huang J, Gu J, Zhou W, Gao L. 2017. Decomposition of emergent aquatic plant (cattail) litter under different conditions and the influence on water quality. Water Air Soil Pollut. 228(2):70.
- Wu Xian H, Yang GQ. 2007. Early-stage litter decomposition and its influencing factors in the Wetland of the Sanjiang Plain, China (in Chinese). Acta Ecol Sin. 27(10):4027–4035.
- Xiaolong W, Jingyi H, Ligang X, Rongrong W, Yuwei C. 2014. Soil characteristics in relation to vegetation communities in the wetlands of Poyang Lake, China. Wetlands. 34(4):829–839.
- Xie Y, Yu D, Ren B. 2004. Effects of nitrogen and phosphorus availability on the decomposition of aquatic plants. Aquat Bot. 80(1):29–37.
- Xiong S, Nilsson C. 1999. The effects of plant litter on vegetation: a meta‐analysis. Ecology. 87(6):984–994.
- Yan Baoshan L, Zheyuan CY. 2012. Litter decomposition of six macrophytes in a eutrophic shallow lake (Baiyangdian Lake, China). Clean Soil Air Water. 40(10):1159–1166.
- Zhang G, Yu X, Zhang Q, Li Y, Liu Y, Duan H. 2018. Variation in the distribution of soil microbial community structure along ground water level gradients in the Poyang Lake Wetland (in Chinese). Acta Ecol Sin. 38(11):1–12.
- Zhang Yu Q, Hu XB. 2013. Research on the characteristics of plant communities in the Poyang Nanji Wetlands, China (in Chinese). Resour Sci. 35(1):42–49.
- Zhang G, Yu X, Gao Y, Li Y, Zhang Q, Liu Y, Rao D, Lin Y, Xia S. 2018. Effects of water table on cellulose and lignin degradation of Carex Cinerascens in a large seasonal floodplain. J Freshwater Ecol. 33(1):311–325.
- Zhao Q, Bai J, Liu P, Gao H, Wang J. 2015. Decomposition and carbon and nitrogen dynamics of Phragmites Australis litter as affected by flooding periods in coastal wetlands. Clean Soil Air Water. 43(3):441–445.