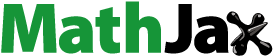
Abstract
For the purpose of studying the response of the Vallisneria natans antioxidant enzyme defense system to different water depths, we selected seedlings with well-preserved rhizomes, consistent germination and positive growth as experimental plants and put them into 10 plexiglass barrels at depth gradients. After 60 days of simulation experiments, we tested the malondialdehyde (MDA), superoxide dismutase (SOD), peroxidase (POD), catalase (CAT), chlorophyll and soluble protein contents and root activity. The results showed that (1) the contents of Chla, Chlb, Chl (a + b) and Car first increased with water depth and then decreased, reaching maximum values of 0.99, 0.38, 1.37 and 0.20 mg/g, at 110 cm respectively; (2) SOD and POD decreased with increased water depth, with a significant decreasing trend particularly between 30 and 60 days, and MDA showed the same trend as POD. CAT decreased with increased water depth but then showed a two-fold increase trend, probably due to the gradual effects of long-term water stress the antioxidant enzyme system in V. natans leaves. (3) Soluble protein had the same trend as MDA. Root activity implied that the more active the root system is, the more ramets and biomass are produced. In this experiment, the plant grew well at depths of less than 120 cm and showed clear signs of aging or death beyond this depth; the results showed that the antioxidant enzyme defense system engaged a series of antioxidant protection mechanisms to increase plant stress resistance ability and reduce or eliminate the damage of plants, but when the stress increased beyond the tolerance of the plant, the antioxidant defense system was destroyed, which eventually led to the death of the plant.
Introduction
The submerged macrophyte is one of the main primary producers of lake ecosystems, which play an important role in the terrestrial nutrient and carbon cycles and provide a critical habitat for a number of other biological elements for the overall lake ecosystem (Jian et al. Citation2015; Li et al. Citation2014; Liu et al. Citation2016). The submerged macrophyte can reduce the nutrient mass ratio of the water, promote the deposition of suspended substances and improve sewage self-purification capacity. Due to long-term immersion in the water, submerged macrophytes can directly absorb dissolved oxygen and other nutrients, provide food for fish and become attachment substrates for algae (Toporowska et al. Citation2008; Ji et al. Citation2015). Moreover, they also produce O2 through photosynthesis, increase dissolved oxygen and absorb, precipitate and enrich nitrogen-phosphorus in water. They can absorb pollutants (such as phenol and cyanide) in the water and then transform and decompose them through enzymes and biochemical functions to eliminate their toxicity (Shang et al. Citation2015). As a result, many scientists and management agencies have focused on protecting and restoring macrophytes in shallow waters (Novak and Chambers Citation2014; Verhofstad et al. Citation2017).
With the intensification of human activities, the eutrophication of water is exacerbated, and algae outbreaks in the lake affect the underwater light and lead to the reduction or even disappearance of submerged macrophytes, which in turn leads to the disruption of the lake ecosystem (Egertson et al. Citation2004; Orth et al. Citation2010). Related studies have shown that water depth can affect multiple water indicators, such as water temperature, pH, DO, light, nutrition, chlorophyll, sediment, wind and waves, of which light is the most affected factor (Bai et al. Citation2011; Zhu et al. Citation2012; Fu et al. Citation2014). Moreover, water depth is an important environmental factor affecting the growth, reproduction and distribution of the submerged macrophyte (Xiao et al. Citation2010; Ye et al. Citation2018). Increasing the water depth and the action of dissolved substances, suspended substances and algae results in a corresponding change in underwater light and oxygen dissolved in water, thus inhibiting the growth of the submerged macrophyte (Li et al. Citation2008; Xie et al. Citation2012). Generally, the maximum growing depth of submerged macrophytes has been widely used as a simple variable in monitoring European lakes. Moreover, a certain water depth is conducive to the maintenance of stable macrophyte communities (Geest et al. Citation2005) because excessively high or low water depth is detrimental to the stability of submerged macrophyte communities (Zhu et al. Citation2012; Li et al Citation2015). However, the relationships between submerged macrophyte growth stages and water depth still need to be uncovered.
Vallisneria natans is often used as a pioneer plant for vegetation restoration and sewage treatment in wetlands. It has a strong ecological adaptability, a great ability to absorb pollutants, and low light stress tolerance (Yin et al. Citation2007). The morphological and physiological characters of V. natans will change to adapt to adversity. To date, researches has mainly focused on the effects of the water depth gradient on the distribution (Wen et al. Citation2014), growth, biomass (Cao et al. Citation2014), photosynthetic fluorescence characteristics (Yang et al. Citation2014) and photosynthesis and enzyme activity of V. natans (Yang et al. Citation2016), as well as the effects of elevated carbon dioxide and temperature (Cao and Ruan Citation2015), heavy metal stress (Hu et al. Citation2011) and long-term low light stress on V. natans (Li and Xie Citation2013). However, there have been few studies on the effect of water depth gradient on the physiological characteristics of V. natans or on the role of V. natans in the restoration and reconstruction of wetlands, which could help determine the optimal submerged macrophyte biomass and regulate restoration. Our research through the V. natans, pioneer plant of Poyang Lake wetland to to studied the effects of water depth on the physiological parameters and explored the response mechanisms of V. natans to water depth. Therefore, based on the changes in V. natans physiological indicators, this study further studied the effects of water depth on the physiological parameters and explored the response mechanisms of V. natans to water depth in order to provide the most suitable aquatic ecosystem restoration and reconstruction program with V. natans.
Methods
The experimental plant, V. natans, was collected from the river surrounding the campus of Jiangxi Normal University (E 115°55′, N 28°41′) in March 2015. We selected V. natans seedlings 15.0 ± 0.52 cm in height with well-preserved rhizomes, consistent germination and positive growth as the experimental plants, and each V. natans seedling had four leaves on average. The selected seedlings were planted in plastic basins (34 × 26.5 × 13 cm) with 10 cm sediment, and then the basins were placed in a pool (3.5 × 1.7 × 0.92 m) in the Key Laboratory of Poyang Lake Wetland and Watershed Research and Education (glasshouse).
This experiment was an indoor control experiment. In this experiment, the selected plants were planted in pots (top diameter: 16 cm, bottom diameter: 14 cm, height: 17.5 cm). The substrate was collected from the sediment from the river surrounding the campus (substrate thickness: 10 cm), total nitrogen (TN) was 3.64 g·kg−1 and total phosphorus (TP) was 0.68 g·kg−1. Every pot was tied with a rope in order to facilitate the measurement of data, and each pot contained three plants. There were a total of 90 pots, with 3 pots set at each tested depth in an plexiglass barrel (height: 60, 70, 80, 90, 100, 110, 120, 130, 140, 150 cm). We placed three pots in an plexiglass barrel (transparent, no cover), filled it with water from the test pool, and added fresh water to maintain the level every 15 days. We maintained the water at 20 ± 6 °C, pH 7.28, and each treatment was repeated three repititions. The test was conducted from April 3 to June 3, 2015, and the temperature was controlled at 20–30 °C. The whole experiment was conducted under natural light.
Every 5 days, we used a MODEL ZDS-10 underwater illumination meter to measure the light intensity at different water depths of 10, 20, 30, 40, 50, 60, 70, 80, 90, 100, 110, 120, 130, 140 and 150 cm, and repeated five times due to weather condition. The data varied widely, so we took the average of the measured data as the effective data. The tendency of the light intensity within the water depth range is shown in .
The chlorophyll content of plant leaves was determined by spectrophotometry of a 95% ethanol extraction. SOD was determined by the nitro-blue tetrazolium method. POD was determined by the guaiacol method. CAT was determined by permanganate titration. MDA was determined by the thiobarbituric acid method. Soluble protein was determined by the Coomassie Brilliant Blue G-250 staining method. Root activity was determined by triphenyl-tetrazolium chloride (TTC). The TP contents of the soil and water were determined by the ammonium molybdate spectrophotometric method. The TN content was determined by the UV spectrophotometric method. Nessler’s reagent spectrophotometry method was used for the determination of NH4+-N, P-aminobenzamide-naphthalene-ethylenediamine spectrophotometric method for the determination of NO2−-N, spectrophotometric method with phenol disulfonic acid for the determination of NO3−-N, and iodimetry for the determination of dissolved oxygen (Water and wastewater monitoring and analysis methods of the state environmental protection bureau Citation2002). The results of the main water quality indicator content measurements are shown in ().
Table 1. The average values of water parameters followed by standard deviation during the experiment mg/L.
Repeated measures ANOVA were used to analyze and test the significance of differences in V. natans chlorophyll and enzyme activity. Excel and SPSS 19.0 software were used to analyze the test data. Correlation analysis of V. natans each indicator using Pearson method; P < 0.01 indicated extremely significant correlation, and P < 0.05 indicated significant correlation.
Results and analysis
Light intensity is one of the major factors that affect the growth of submerged macrophytes, but when it is lower than the light compensation point of the plant, then the net production of the plant is negative, and the plant cannot survive. The transmission of light in water can be approximated as an exponential decay function:
Iz and I0 are the light intensities at water depth z and the surface, respectively. The attenuation coefficient E in the vertical direction indicates how fast the light decays in the water body. The distribution of light in the test water can be fitted as:
The attenuation coefficient of light in water was E = 0.0906, and the light intensity at the water depth of 150 cm was 1.117 klx. The light intensity was significantly negatively correlated with the water depth, .
The change trends of Chla, Chlb, Chl(a + b) and Car are shown in , and the chlorophyll content of V. natans leaves showed different trends with the increase of the water depth gradient. As for the influence of the depth gradients on the V. natans leaf, the effect on Chla content was significant (P < 0.01). Under the influence of the water depth gradient, the Chla content had a trend that first increased and then decreased. The Chla content was increased from 0.66 mg·g−1 (60 cm) to 0.99 mg·g−1 (110 cm), an increase of 50.0%. The variation trend of Chlb was consistent with that of Chla, but the variation range of Chlb was relatively small, and the maximum amplitude of differences between adjacent treatment groups was 0.06 mg·g−1. Chla, Chlb and Chl(a + b) contents decreased clearly with increased water depth; under 110 cm, the minimum values were 0.51, 0.19 and 0.70 mg·g−1, respectively, and all appeared in the 150 cm treatment group. This result showed that the photosynthesis of the plants was obviously affected by the increase in water depth, but the change in Car was not obvious (P > 0.01). Thus, we knew that the depth gradient had no obvious effect on the content of Car, but its effect on Chla, Chlb and Chl(a + b) was significant (P < 0.01).
After 30 days, SOD activity in the different depth treatments showed little difference, but the activity at the depth of 150 cm had declined obviously; it was decreased by 54.9% compared with 140 cm group, while the 60–140 cm groups all fluctuated around approximately 65.47 U·mg−1. The variation trend of SOD activity was more obvious after 60 days. With the increase in water depth, enzyme activity decreased continuously, and the attenuation coefficient was 0.04.
The results of the double factorial analysis of variance showed that the SOD of the V. natans leaf was significantly affected by different water depth gradients after 60 days (P < 0.05). SOD decreased with increased water depth. At 1 month into the experiment, leaves had already begun to respond to the depth stress. SOD decreased significantly between the 140 and 150 cm treatment groups, indicating that leaves in the 150 cm group suffered the highest water stress.
As shown in , the POD of V. natans leaf responded significantly (P < 0.05) after 30 days, and it generally showed a downward trend over the water depth range of 60–150 cm. The attenuation coefficient was 0.20, and the fitting equation was y = 146.89e(−0.2024x). At 60 cm depth, POD was 101.81 U·(g·min)−1, which was much higher than that in the other treatments, possibly due to the shallow water at the beginning of the experiment, so the response to supersaturated light intensity was obvious. Light stress led to increased POD, removed the active oxygen that was produced by the membrane system, and increased plant stress resistance, which is a response mechanism to water depth. After 60 days, POD still tended to decrease, but at 140 and 150 cm, it was higher than that at 130 cm depth, probably due to the increase in water depth and the decrease in light intensity. POD increased to cope with the stress, but the long-term deep water and low-light conditions caused yellowing on the top of the leaves, and eventually the old leaves of the plant slowly declined.Depth gradients have different effects on V. natans CAT activity (). CAT decreased drastically in the depth range of 60–80 cm, with a maximum decrease of 50%. At deeper points on the depth gradient, the 80–100 cm group had a slow rise trend, but the increase was less than the drop in the previous range. However, within the 100–130 cm depth range, CAT showed a significant downward trend, dropping to the lowest point of 0.01 U/mg (130 cm), followed by an upward trend.
The content of MDA in leaves decreased gradually within the depth range of 60–110 cm, and the maximum decrease between adjacent treatments was 28.4%. However, it had a slight increase (3.34 nmol·g−1) at the depth of 120 cm, and the overall trend still decreased, which was consistent with the trend of POD enzyme activity.
From , the soluble protein content in the leaves of V. natans had a trend which dropped first, then rose, and then dropped with the increase of water depth. Soluble protein content decreased from 60 to 110 cm, but at 120 cm, it increased significantly and then decreased. In the shallow water, the soluble protein decreased overall as a response to environmental stress, with the lowest drop to 4.59 mg/g (150 cm), and the relative maximum value of 9.00 mg/g (60 cm) decreased by nearly 50%. This result indicates that environmental stress may have surpassed the limit of the plant's tolerance, triggering protein hydrolysis, a decrease in soluble protein content, and damage to the plant cells. The V. natans leaves that were planted at a 130–150 cm depth were relatively yellowish, and the plant morphology index was lower than for other treatment groups.
Due to cell division, the root is in the apical part, so this part is generally taken for measurement. In the depth range of 60–110 cm, the root activity showed a decreasing trend, but then it increased–decreased–increased, and the overall coefficient of decline was 0.0038. In the range of 60–120 cm depth, the growth of V. natans was obvious, and there were more ramets; the plants at 130–150 cm were mainly in the slow growth stage, the roots were mainly old plants, the new ramets were fewer in number, and the root activity was relatively weak, but individual plants at 150 cm grew slightly better, and the root activity was increased to 5.12 mg/(g·h)−1. Root activity is an indicator of plant growth status, and the more active the root system is, the more active the underground part is. Therefore, the root could continue to absorb mineral elements from the soil to supply the growth of the aboveground parts. Root activity can sometimes be used as the standard for transplanting plants. The root activity determination is very important for understanding the growth status of plant. We generally use the triphenyl-tetrazolium chloride method, referred to as the TTC method ()
Pearson correlation analysis can be used to further study the relationship between the indexes under the water depth gradient (). Correlation analysis showed that water depth was significantly negatively correlated with light intensity at P < 0.01. Root activity was significantly negatively correlated with water depth at P < 0.05. Plant growth mainly relied on the absorption of nutrients by the roots to supplement the growth of aboveground biomass. A decrease in root activity indicated that the water depth gradient exerted a stress on plant growth. At the same time, SOD, POD and MDA were significantly negatively correlated with water depth at P < 0.01. However, there was no significant correlation between CAT and water depth or other indexes in plant leaves, which meant that water depth had no obvious effect on all enzyme defense systems. There was no significant correlation between the root activity of the underground part and the aboveground biomass of the plant.
Table 2. The correlation between biological characteristic values after 60 days.
Discussion
In a stress-inducing environment, V. natans undergoes changes in plant height, leaf length, leaf number, root length, biomass distribution and other morphological traits to adapt to different water depth conditions (Xiao et al. Citation2007). The depth distribution of V. natans is related to water transparency and depth. When light is sufficient, the number of plants per unit area can increase by clonal reproduction (Fu et al. Citation2012; Dai et al. Citation2014; Wen et al. Citation2014). Heavy metal copper stress could also inhibit the physiological indexes of V. natans. In the early stage of Cu stress induced by Cu2O and Cu(OH)2, the antioxidant enzyme activity increased because of the peroxidative stress response. Lipid peroxidation appeared to reflect a certain degree of peroxidative damage, and the chlorophyll content decreased, but with longer growth times, V. natans appeared adaptable to copper stress, as peroxide damage was eventually alleviated and the chlorophyll content rose again (Hu et al. Citation2011). The change in biomass was closely related to the CO2 concentration in the water; under a high CO2 content, most of the biomass accumulation was in the root and ramet of V. natans, and leaf growth was not obvious (Cao and Ruan Citation2015). Under salt stress, the active oxygen can destroy the functional molecules of the plant (Liu et al. Citation2011). In response to this situation, the antioxidant defense system produced MDA while the peroxidase enzyme acted. The antioxidant content would increase to resist the adverse environment, but it would decrease when the plant endured a long-term adverse environment, which would lead to the slow senescence of the plant.
Chlorophyll content is an important physiological index that reflects and directly affects the photosynthesis of plant leaves and can also be used to reflect the senescence of the plant tissues and organs under adversity (Huang et al. Citation2009). The chlorophyll content of the submerged macrophyte leaf is much greater than in other plants, which makes it easy for macrophytes to adapt to, use, and fully absorb the weak light during their growth. When the received light intensity is large, V. natans will increase their chlorophyll content through a series of physiological functions to increase the ability to obtain light quanta. The decrease in the later stage of the stress response indicated that the light intensity gradually decreased and the photosynthesis of the plant was affected. This result can also be confirmed by studies of the effects of water depth, light and other factors on the physiological characteristics of V. natans (Li et al. Citation2008; Yang et al. Citation2014).
When plants are in a stressful environment, it will lead to the production and accumulation of superoxide anion free radicals, hydrogen peroxide, hydroxyl radical, etc., and cause damage to cell lipids, proteins and sugars (Liu et al. Citation2012). Antioxidant enzymes such as SOD, POD and CAT provide a protective mechanism through a series of antioxidants to reduce or eliminate this kind of damage. SOD is the first antioxidant enzyme that works in an aerobic biologically active oxygen scavenging system. It can catalyze a rapid disproportionation that changes the superoxide anion free radical O2− to molecular oxygen and H2O2, to reduce the peroxidative damage to the plant (Ma and Zhu Citation2003). POD is a common oxidoreductase in plants that can catalyze the oxidative decomposition of toxic substances. Membrane lipid peroxidation often occurs when plant organs are aged or suffer from stress. MDA is a product of this process in plants and is often used to measure the degree of membrane lipid peroxidation. In this experiment, when V. natans was under waterlogging stress, its antioxidant enzyme defense system accelerated the oxidative decomposition of toxic substances or reduced plant peroxidation damage to increase plant stress resistance. SOD, POD and MDA showed a decreasing trend, which signifies that the response of the reaction system to stress can be used as an indicator of plant growth. The activity of antioxidant enzymes and MDA decreased continuously with the increase in depth. This finding indicated that after the first 30 days, the defense mechanism represented by MDA that was triggered by the stress-induced damage of plant tissue due to long-term exposure to the stressful environment weakened gradually, as did the physiological metabolism of the plant. The above results demonstrated that the plant's antioxidant enzyme defense system can protect the plant within a certain range of stress, but the defense system will lose its ability to function if it exceeds this stress range. This finding is consistent with other studies on the reaction of the antioxidant enzyme system when V. natans is under other stresses (Song et al. Citation2011; Wang et al. Citation2012).
Soluble protein is an important regulatory substance and nutrient that plays a protective role in the organismal material and biofilm. Soluble protein had a decrease–increase–decrease trend with the increase in water depth, and decreased to 4.59 mg/g (at 150 cm), which indicated that the water stress at this depth may exceed the tolerance limit of the leaves, causing the soluble protein content to decrease and the plant cells to be damaged. The plant root system is an active absorptive and synthetic organ. The growth status and activity level of the roots directly affect the nutrition status and yield level of the aboveground parts. Root activity is a value that characterizes the root system of the plant. The root system is the basis of plant growth; the protein hydrolysis and root activity decreased with the increase of water depth; and the decrease coefficient was 0.0038. However, correlation analysis with water depth and soluble protein content showed that there was no significant correlation between the root activity of the underground part and the leaf enzyme activity of the aboveground part, and the specific relationship remains to be further studied.
Conclusion
In this experiment, the plant grew well at depths of 120 cm or less and showed clear signs of aging or death beyond this gradient. However, with the increase in the water depth gradient, which means a decrease in light intensity, the stress exceeds the tolerance of V. natans, damaging the antioxidant enzyme defense system and accelerating the aging of plants, eventually leading the plant to death. This is also a problem that we need to consider in the process of restoring and reconstructing the lake water ecological environment.
Disclosure statement
No potential conflict of interest was reported by the authors.
Funding
This research was supported by the Natural Scientific Foundation of China (41361017, 41661102), the Natural Scientific Foundation of Jiangxi Province (20181BAB203021), the Youth Natural Scientific Foundation of China (41603077).
Additional information
Notes on contributors
Yun Cao
Cao Yun received his PhD from Nanjing Normal University in 2007. His research interests are in plant ecology and ecological restoration in wetlands. He is currently working on a research project about the ecological amplitude of beach-typical vegetation in Poyang Lake under a water level gradient.
Dan Chen
Chen Dan is a graduate student at Jiangxi Normal University. Her research interest is in submerged vegetation restoration.
Xin-Sheng Ji
Ji Xin-Sheng is a graduate student at Jiangxi Normal University. Her research interest is in submerged vegetation restoration. She is currently working on understanding the influence of environmental factors on the growth of submersed macrophytes.
Su-Juan Zhang
Zhang Su-Juan received her master's degree from Jiangxi Normal University in 2016. Her research interest is in submerged plants of Poyang Lake. She is currently working on the spatial-temporal changes of the submerged plant V. natans driven by hydrological systems in Poyang Lake.
Qi Huang
Huang Qi received his PhD form Nanjing Institute of Geography and Limnology, Chinese Academy of Sciences. His current research explores the ecologically relevant water quality criteria for shallow dish lakes in Poyang Lake wetlands.
Wen-Lin Wang
Wang Wen-Lin received his PhD from Nanjing Normal University in 2014. His research interests are in wetland ecology and ecological restoration in wetlands. He is currently working on a research project about the characteristics and regulatory mechanisms in the oxygen-rich of the urban black-odor river sediment microinterface.
References
- Bai X, Chen K, Ren KW,Chen XM,Yang H. 2011. Influence of Myriophyllum spicatum’s growth on nitrogen and phosphorus in sediment in different water depth. Ecol Environ Sci. 20(6/7):1086–1091.
- Cao J, Ruan H. 2015. Responses of the submerged macrophyte V. natans to elevated CO2 and temperature. Aquat Biol. 23(2):119–127.
- Cao Y, Zhang SJ, Liu YY, Guo ZC,Chen BX. 2014. Effects of water gradient on seedlings growth and biomass of V. natans. Ecol Environ Sci. 23(8):1332–1337.
- Dai QS, Zhang CY, Wang YJ, Wang LY, Cai H, Wang TX, Wang N. 2014. Study on purification effect of autumn submerged plants on artificial lake. J Wetland Sci Manag. 10(2):38–41.
- Egertson CJ, Kopaska JA, Downing JA. 2004. A century of change in macrophyte abundance and composition in response to agricultural eutrophication. Hydrobiologia 524(1):145–156.
- Fu H, Zhong J, Yuan G, Xie P, Guo L, Zhang X, Xu J, Li Z, Li W, Zhang M, et al. 2014. Trait-based community assembly of aquatic macrophytes along a water depth gradient in a freshwater lake. Freshw Biol. 59(12):2462–2471.
- Fu H, Yuan GX, Cao T, Ni LY,Zhang XL. 2012. Clonal growth and foraging behavior of a submerged macrophyte V. natans in response to water depth gradient. J Lake Sci. 24(5):705–711.
- Geest GJV, Wolters H, Roozen FCJM, Coops H, Roijackers RMM, Buijse AD, Scheffer M. 2005. Water-level fluctuations affect macrophyte richness in floodplain lakes. Hydrobiologia. 539(1):239–248.
- Hu F, Pu HQ, Chen LY,Cheng SP. 2011. Effects of cuprous oxide (Cu2O) and cupic hydroxide[Cu(OH)2]on activity of antioxidase and content of chlorophyll in V. natans L. J Ecol Rural Environ. 20(7):72–78.
- Huang YJ, Ynag H F, Yang J H, Wang X L, ZHou S B. 2009. Effects of Cu stress on Alternanthera Philoxeroides growth and reactive oxygen species metabolism. Chin J Ecol. 28(6):1112–1116.
- Ji HT, Xie D, Zhou HJ,An SQ. 2015. Effects of submerged macrophyte on the growth of three common algae species. Acta Ecologica Sinica. 34(2):445–453.
- Jian MF, Li LY, Yu HP, Xiong JQ, Yu GJ. 2015. Effects of heavy metal pollution on submerged macrophyte in the water body and sediments of Poyang Lake Wetland. Acta Ecologica Sinica. 24(1):96–105.
- Li C-h, Wang B, Ye C, Ba Y-X. 2014. The release of nitrogen and phosphorus during the decomposition process of submerged macrophyte (Hydrilla Verticillata Royle) with different biomass levels. Ecol Eng. 70:268–274.
- Li H-L, Wang Y-Y, Zhang Q, Wang P, Zhang M-X, Yu F-H. 2015. Vegetative propagule pressure and water depth affect biomass and evenness of submerged macrophyte communities. Plos One. 10(11):e0142586.
- Lee HS. 2000. Principles and techniques of plant physiological and biochemical experiments. Higher Education Press: Beijing, China.
- Li HJ, Ni LY, Cao T, Zhu LX. 2008. Responses of V. natans to reduced light availability and nutrient enrichment. Acta Hydr Sin. 32(2):225–230.
- Li Q, Xie Y. 2013. Influence of low light on the growth and development of V. natans seedlings. AMM. 295-298:62–68.
- Liu X, Zhang Y, Shi K, Lin J, Zhou Y, Qin B. 2016. Determining critical light and hydrologic conditions for macrophyte presence in a large shallow lake: The ratio of euphotic depth to water depth. Ecol Indic. 71:317–326.
- Liu XP, Zhang YJ, Li Y, Dong Y,Wen XF, Yi M, He P M, LiI JY. 2012. Effects of cycocel on the dwarfing characteristics and physiological indices of V. natans. Chin J Ecol. 31(10):2561–2567.
- Liu XP, Zhang YJ, Wang C, Ma HF, Li Y, Dong Y, Yi M, Wen XF. 2011. Experimental study on the effects of salt stress on physiological indexes of Vallisneria/The Eighth Conference of Chinese Algae Society and the 16th Symposium.
- Ma XJ, Zhu DH. 2003. Studies on superoxide dismutase (SOD) in plants. Yi Chuan. 25(2):225–231.
- Novak PA, Chambers JM. 2014. Investigation of nutrient thresholds to guide restoration and management of two impounded rivers in south-western Australia. Ecol Eng. 68(7):116–123.
- Orth RJ, Williams MR, Marion SR, Wilcox DJ, Carruthers TJB, Moore KA, Kemp WM, Dennison WC, Rybicki NB, Bergstrom P, Batiuk RA. 2010. Long-term trends in submersed aquatic vegetation (SAV) in Chesapeake Bay, USA, related to water quality. Estuaries Coasts. 33(5):1144–1163.
- Shang YY, Guan BH, Zheng JW, Kang YH. 2015. Effects of Cyanobacteria accumulation on aquatic environment and growth of submerged macrophyte. Chin Agric Sci Bull. 31(5):195–198.
- Song R, Jiang J, Geng J, Gao S-X. 2011. Physiological effect of V. natans L. under different concentrations of ammonia. China Environ Sci. 31(3):448–453.
- Toporowska M, Pawlik-Skowrońska B, Wojtal A. 2008. Epiphytic algae on Stratiotes aloides L. Potamogeton lucens L. Ceratophyllum demersum L. and Chara Spp. in a macrophyte-dominated lake. Oceanol Hydrobiol Stud. 37(2):51–63.
- Verhofstad MJJM, Núñez MMA, Reichman EP, van Donk E, Lamers LPM, Bakker ES. 2017. Mass development of monospecific submerged macrophyte vegetation after the restoration of shallow lakes: roles of light, sediment nutrient levels, and propagule density. Aquatic Bot. 141:29–38.
- Wang P, Zhang S, Wang C, Lu J. 2012. Effects of Pb on the oxidative stress and antioxidant response in a Pb bioaccumulator plant V. natans. Ecotoxicol Environ Saf. 78(2):28–34. (
- Water and wastewater monitoring and analysis methods of the state environmental protection bureau 2002. Water and Wastewater Monitoring and Analysis Methods – edition 4. China Environmental Science Press.
- Wen W, Shao L, Wu JY, Yu K, Hu Z, He P. 2014. Distribution properties and effects of light intensity on V. natans in Litang river. J Shanghai Ocean Univ. 23(6):897–903.
- Xiao C, Wang X, Xia J, Liu G. 2010. The effect of temperature, water level and burial depth on seed germination of Myriophyllumspicatum and Potamogeton malaianus. Aquatic Bot. 92(1):28–32.
- Xiao K, Yu D, Wu Z. 2007. Differential effects of water depth and sediment type on clonal growth of the submersed macrophyte V. natans. Hydrobiologia 589(1):265–272.
- Xie YC, Li Q, Wang GX. 2012. Effects of long-term low light on the growth and development of V. natans seedlings. Chin J Ecol. 31(8):1954–1960.
- Yang X, Sun SY, Bai X, Zhang QC, Chen KN. 2014. Influences of water depth gradient on photosynthetic fluorescence characteristics of V. natans. J Lakes Sci. 26(6):879–886.
- Yang X, Zhang Q-C, Sun S-Y, Chen K-N. 2014. Effects of water depth on the growth of V. natans and photosynthetic systemII. Photochemical characteristics of the leaves. Ying Yong Sheng Tai Xue Bao. 25(6):1623–1631.
- Yang ZQ, Han D, Wang XL,Jin ZF. 2016. Changes in photosynthetic characteristics and activities of protective enzymes in four tea cultivars during cold wave process and varietal differences. Acta Ecol Sin. 36(3):629–641.
- Ye B, Chu Z, Wu A, Hou Z, Wang S. 2018. Optimum water depth ranges of dominant submersed macrophytes in a natural freshwater lake. Plos One. 13(3):e0193176.
- Yin Y, Sun Y-Y, Guo H-Y, Wang X-R. 2007. Biotoxic effects of earthworms on Vallisneriae. Ying Yong Sheng Tai Xue Bao. 18(7):1528–1533.
- Zhu G, Li W, Zhang M, Ni L, Wang S. 2012. Adaptation of submerged macrophyte to both water depth and flood intensity as revealed bytheir mechanical resistance. Hydrobiologia 696(1):77–93.