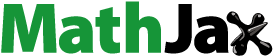
Abstract
For a better understanding of the metabolic adaptations to sulfonamide (SA) stress in submerged macrophytes, the alterations of nutrient element uptake and secondary metabolism were studied in the leaves of Vallisneria natans (Lour.) Hara exposed to 0 and 50 mg/L of SA for 15 d. The results showed that, under a treatment of 50 mg/L SA, the malondialdehyde and superoxide anion (O2•−) contents in V. natans leaves significantly increased, 1.77–3.32 times higher than the control group, respectively, while the chlorophyll concentration showed a 36.99% reduction at the end of experiment. Simultaneously, V. natans showed a removal effect on SA and SA dramatically affected the nutrient removal efficiency by V. natans. Our results suggest that high SA levels can damage V. natans tissue and in turn that results in a stimulation of the antioxidant response. V. natans significantly improved the removal efficiency of SA, meanwhile SA resulted in less removal of TN and TP from the water column.
Introduction
Sulfonamide antibiotics (SA) are used in human and veterinary medicine to treat and prevent infectious bacterial diseases (Yang et al. Citation2011). SA consumption has increased worldwide, and their residues are frequently reported in aquatic environment research (Bartelt-Hunt et al. Citation2011; Garcia-Galan et al. Citation2011; Gonzalez et al. Citation2013). SA concentrations are very low in the water environment, but long-term persistence and continual exposure can induce antibiotic-resistant bacteria or genes that increase potential health and ecological risks (Baran et al. Citation2011; Gonzalez et al. Citation2013; Zhang et al. Citation2015; Qiu et al. Citation2016). Submerged macrophytes play an important role in aquatic ecosystems through accumulation and decomposition of contaminants and they are commonly used for river and lake restoration (Zhou et al. Citation2012). Compared to free-floating macrophytes, submerged macrophytes can assimilate nutrients though the whole plant (Li et al. Citation2018), release oxygen by photosynthesis, and supply nutrients and micro-niches for biofilms through their leaves (Michael et al. Citation2008). Biofilms consists of a complex consortium of bacteria, algae, fungi, as well as other metazoans (Wu et al. Citation2012) and they have a significant ecological function in modulating nutrient cycles and improving water quality (Gong et al. Citation2018).
Vallisneria natans is a fully submerged, rooted plant that is widely distributed in freshwater worldwide. Compared to other submerged macrophytes, V. natans has been proven to have superior survival and growth potential in eutrophic lakes (Qiu et al. Citation2001). It has been suggested that this genus can provide positive feedback for aquatic ecosystem restoration by affecting the benthic metabolism and enhancing the ecosystem capacity to control nitrogen contamination (Pinardi et al. Citation2009).
Previous studies have mainly focused on the purification capacity of nutrient salts and heavy metals (Hijosa et al. Citation2011; Sim et al. Citation2011; Wang et al. Citation2012). Consequently, there is a general lack of knowledge regarding how aquatic macrophytes are involved in the uptake of antibiotics.
The aim of this research is to investigate the potential effect of SA on the physiology of V. natans, as well as the nitrogen and phosphorus removal capacity of V. natans under SA treatment. Our research results will be able to positively improve the understanding of antibiotic interactions with submerged macrophytes in a wastewater treatment system.
Materials and methods
Cultivation of V. natans
The submerged macrophyte V. natans, grown in a concrete pool (length 10 m, width 5 m and depth 1.5 m) in Shanghai Ocean University, China (originally collected from Lake Dian), was selected for the experiment. Individuals of similar size were maintained in a container with 10% Hoagland nutrient solution for 10 d indoors to acclimate the samples before the experiments. The plants were then cleaned with deionized water to remove biofilm. One hundred and sixty plants approximately 10 cm in height with an individual weight of 9–10 g were selected for the experiment. The plants were randomly assigned to each mesocosm.
Experimental design
Experiments in the laboratory were divided into three treatments (): (1) V. natans grown in Hoagland nutrient solution diluted 1:10 (CK1), (2) V. natans grown in Hoagland nutrient solution diluted 1:10 and treated with 50 mg/L SA (SD-T), and (3) Hoagland nutrient solution diluted 1:10 and treated with 50 mg/L SAs but without V. natans (CK2). Twenty plants each for the CK1 and SD-T treatments (four replicates each) with a plant height of 9.58 ± 0.21 cm and a fresh weight of 10.12 ± 0.32 g were cultivated in a 5-L Plexiglas container with 100 mm of sediment (6 mm clear glass beads) and 3 L of modified medium. Plants were incubated at 26.0 ± 1 °C under a continuous light of 80 μmol quanta m−2 s−1 (PPFD) using a 12:12 h dark:light cycle in a container. They were replenished with ultrapure water every day due to evaporation. Plant samples were collected on Day 15 of the culture. Total nitrogen (TN), total phosphorus (TP) of CK1, and SD-T group were determined on Day 1, Day 3, Day 6, Day 12, and Day 15, while the SA content of CK2 and SD-T were determined each day. After the experimental treatments, plants were harvested and rinsed three times with ultrapure water. These plants’ leaves were then selected to measure the parameters of chlorophyll content, malondialdehyde (MDA), and superoxide radical. The fresh samples were frozen in liquid nitrogen and then used to determine the chlorophyll content and antioxidant enzymatic activities.
Table 1. Concentrations (mgL−1) of SAs and number of V. natans.
Measurement of TN, TP and sulfonamide in water
TN and TP were analyzed using standard methods (American Public Health Association (APHA) Citation2005). High performance liquid chromatography (Agilent 1100 Series, USA) was used to analyze the water sample antibiotics. Approximately 1 mL water samples were filtered through a 0.22-μm membrane filter (Hydrophilic) and used for determination of the residual sulfonamide concentrations in the aqueous medium. Sulfonamide was monitored using high-pressure liquid chromatography equipped with a Waters 2487 UV-vis detector. A 10-μL sample was injected onto a C18 column (250 × 4.6 mm, 5-μm pore size) while maintaining a column temperature of 40 °C. Acetonitrile and phosphoric acid (60: 40 v/v) were used as the mobile phase at a flow rate of 1 mL·min−1. The column effluent was monitored at 259 nm to detect the sulfonamide. Calibration curves of six points showed good linearity for the analyte (R2 = 0.99; p < 0.0001) in the region of the expected sample concentration.
The total removal (Pt) of sulfonamide via different treatments was calculated using the following equation:
where Co is the initial sulfonamide concentration at time zero and Ct is the sulfonamide concentration at time t.
Measurement of MDA and superoxide radical (O2•−)
Leaf samples weighing 0.5 g were homogenized in 5 mL of 10% (w/v) trichloroacetic acid (TCA) to determine MDA. The homogenate was centrifuged at 15,000 × g for 10 min, and 4 mL of 20% TCA containing 0.65% (w/v) 2-thiobarbituric acid (TBA) was added to 1 mL of supernatant. Samples were heated at 90 °C for 25 min; thereafter, the reaction was stopped in an ice bath. Centrifugation was performed at 10,000 × g for 5 min at 4 °C, and the absorbance of the supernatant was measured at 440, 532 and 600 nm. MDA equivalents were calculated following the methods of Hodges et al. (Hodges et al. Citation1999; Jiang et al. Citation2019).
O2– production was measured using a modified method according to Elstner and Heupel (Citation1976). Fresh leaves weighing 1.0 g were ground with 3 mL of 50 mM potassium phosphate buffer (pH = 7.8) and centrifuged at 12,000 × g for 20 min. The addition of 1 mL of 4-aminobenzenesulfonic acid (17 mM) and 1 mL of naphthylamine (7 mM) for 20 min at 25 °C altered the color, and the specific absorption at 530 nm was determined (Zhang et al. Citation2018).
Measurement of total chlorophyll
To determine total chlorophyll (a + b), approximately 0.2 g of fresh leaf samples was collected and then ground in liquid nitrogen. Following extraction in 90% hot ethanol, total chlorophyll was spectrophotometrically measured at 649 and 665 nm and then calculated according to Wang et al. (Citation2008) and Jiang et al. (Citation2019).
Statistical analysis
One-way analysis of variance (ANOVA) was used to test the difference between the experimental group (SD-T) and the control groups (CK1, CK2), and differences were considered statistically signification at p < 0.05. Graphical work was conducted using Origin 9.0 (OriginLab Corporation, Northampton, MA, USA).
Results and discussion
Physiological parameters and photosynthesis of V. natans leaves
Oxidative damage of V. natans leaves
The extent of oxidative damage of V. natans leaves was examined by the MDA concentration and the content of O2−. The MDA content increased during the cultivation time, both in the CK1 and SD-T groups, but the concentration in the CK1 group was obviously lower than that of the SD-T group, showing that the SA altered the lipid and protein structures of the leaf cell. As shown in , O2-production increased with increasing levels of SAs, and there was a significant difference between the control and the SA treatment groups (p < 0.01). The O2-production under treatment SD-T was three times that of the CK group.
Table 2. Effect of SAs on the MDA and O2•− in V. natans leaves.
MDA is the final product of membrane lipid peroxidation, and its content can reflect the degree of stress on plants (Wang et al. Citation2008; Jose et al. Citation2012; Paul et al. Citation2012). The result in this study showed that SA toxicity induced an increase in MDA in the submerged plant V. natans. The increase in the MDA content demonstrated that SAs induce oxidative stress in V. natans.
O2− is one type of Reactive Oxygen Species (ROS) causing oxidative stress and the greater the accumulation, the more the tissue protective ability decreases. It is the first oxygen radical generated in living organisms and plays an important role in signal transduction, oxygen induction, and inflammatory reactions when it is at a nonequilibrium concentration. It can also cause tissue damage. In this study, SA induced a significant increase in the level of O2•− in the plant cells, providing direct evidence for SA antibiotic-induced plant cell production of reactive oxygen species. The increased MDA content in the SA treatment group means that O2•− and other reactive oxygen species do attack the plant cells and trigger lipid peroxidation (Bazivamakenga et al. Citation1995; Dayan et al. Citation1999; Galindo et al. Citation1999).
Chlorophyll of V. natans leaves
Variations in chlorophyll content can be used as an indicator of the growth of V. natans. shows that the chlorophyll concentration significantly decreased (p < 0.05) with the increase in the SA concentration, while an obvious increase is observed in CK1.The chlorophyll content in the SD-T group was 44.28% of the CK1 group on the 15th day. Compared to the beginning of the test, the chlorophyll concentration in the V. natans leaves decreased by 36.99% at the end of the experiment.
Figure 1. Effects of SA on the total chlorophyll in leaves of V. natans. Data are shown as mean ± SD (n = 4); different letters represent significant difference at p < 0.05.
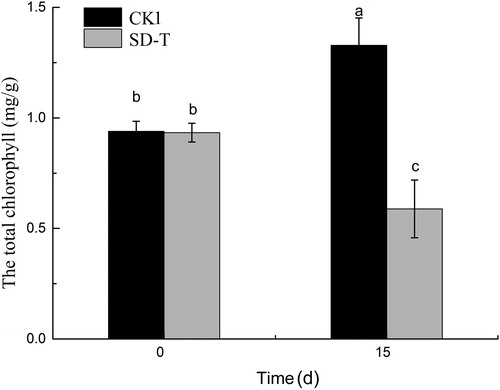
Chlorophyll plays an important role in the absorption of light energy during photosynthesis (Li et al. Citation2018). There are two types of chlorophyll, designated as chlorophyll a and b (chl a and chl b), which differ slightly in chemical makeup and in color. When plants are externally stressed, the leaves change their own morphology and structure to adapt to the environment. Chloroplasts are the most direct and important organelles under environmental stress. Under environment stress, the chlorosis of plant leaves is mainly because of the decrease in chlorophyll content. The change in chlorophyll content can reflect the degree of phytotoxicity of plants to some extent. Liu reported that the chlorophyll content of reeds decreased under oxytetracycline and sulfamethazine stress (Liu et al. Citation2013). In this study, SAs induced a significant reduction in the chlorophyll content. The chlorophyll content decreased 36.99% within 15 d. It is suggested that the SAs could inhibit the synthesis of chl a and chl b and cause deleterious effects on plant photosynthesis. The synthesis of chl a and chl b requires a variety of enzymes (Suzuki et al. Citation1997).
Effects of sulfonamide on nutrient removal of V. natans
As shown in and , the TN and TP removal rates were significantly different between control group 1 and the experimental group. After 3 d, the TN and TP concentrations decreased under each treatment; as the cultivation time increased, the TN and TP concentrations were always lower in the control than in those pre-treated with 50 mg/L SAs (p < 0.05). After 15 d, the TN removal rate reached 43.1% and 29.2% under CK1 and SD-T, while the TP removal rate reached 93.5% and 70.1%, respectively. SA significantly affected the nutrient removal efficiency of V. natans, and the TN and TP concentrations significantly differed between CK1 and SD-T (15 d, p < 0.01).
Antibiotics affect V. natans growth and interfere with nutrient removal. Phosphate can be removed from water via sedimentation, absorption or biological uptake (Song et al. Citation2007). In the present study, the phosphate removal rate in the SD-T group was 75.1% of the CK1 group after 15 d, probably because the high concentration of SA affected the purification ability of V. natans system in water. On the one hand, the plant growth was likely inhibited by SA, on the other hand the biofilm growth on the plant leaves may affect the SA as well. The surface biofilm of submerged macrophyte hosts a large number of microorganisms, which play an important role in nutrient removal.
As shown in , the concentration in both the CK2 and SD-T groups was stable during the first 5 d and then decreased after the 6th day. The SA content in the SD-T group more rapidly decreased compared to that of the CK2 group. The difference between them was not significant on the 10th day but was significant on the 15th day (p < 0.05).
It was found that SA removal may be different from other “common” pollutants such as TN,TP and metals. Photolysis appears to be the main degradation process of SAs in natural waters (Andreozzi et al. Citation2003), whereas direct photolysis, indirect photolysis and self-sensitized photolysis of antibiotic pollutants are common in surface waters exposed to sunlight (λ > 290 nm) (Li et al. Citation2015; Lian et al. Citation2015). Forni et al. (Citation2001) found that the fern plant Azolla has the ability to remove antibiotic sulfamonomethoxine in aqueous solution, and the degradation rate reached 55.7% after 5 weeks. The degradation was mainly caused by biodegradation, and the residue in plants was only 3%. Similar to our study, SA rapidly decreased after 5 d for both groups, and the removal rate reached 78.9% and 92.5% on the 15th day, respectively, suggesting that V. natans system may promote the removal of SA. SA was significantly reduced in both the experimental and control groups, indicating that SA removal was also associated with other factors such as photolysis.
Conclusion
Our study showed that high SA levels had toxic effects on the growth of V. natans and that results in stimulating the antioxidant response. V. natans significantly improved the removal efficiency of SA, meanwhile, SA resulted in less removal of TN and TP in the water column. However, the removal mechanism of SA by V. natans needs to be studied in the future.
Notes on contributors
Houtao Xu is a postdoctoral at School of Agriculture and Biology, Shanghai Jiao Tong University, who is now working on freshwater ecology.
Liming Zhu is a doctor candidate at Shanghai Ocean University, who is now working on Aquatic Biology.
Liqing Wang is a professor at Shanghai Ocean University, where she has contributed to Aquatic Biology and wetland ecology.
Xiaoyan Zheng is a Research and Development Manager at Shanghai Aquatic Environmental Engineering Co., Ltd, who is working on Freshwater Ecology.
Linkui Cao is a professor at School of Agriculture and Biology, Shanghai Jiao Tong University, the research filed is mainly focus on non-point source pollution control and ecological agriculture.
Disclosure statement
No potential conflict of interest was reported by the authors.
Additional information
Funding
References
- Andreozzi R, Raffaele M, Nicklas P. 2003. Pharmaceuticals in STP effluents and their solar photodegradation in aquatic environment. Chemosphere. 50(10):1319–1330.
- American Public Health Association (APHA). 2005. Standards methods for the examination of water and wastewater. Washington, DC: American Public Health Association (APHA).
- Baran W, Adamek E, Ziemiańska J, Sobczak A. 2011. Effects of the presence of sulfonamides in the environment and their influence on human health. J Hazard Mater. 196:1–15.
- Bartelt-Hunt S, Snow DD, Damon-Powell T, Miesbach D. 2011. Occurrence of steroid hormones and antibiotics in shallow groundwater impacted by livestock waste control facilities. J Contam Hydrol. 123(3–4):94–103.
- Bazivamakenga RR, Leroux GD, Simard RR. 1995. Effects of benzonic and cinnamic on membrane permeability of soybean roots. J Chem Ecol. 21(9):1271–1285.
- Dayan FE, Watson SB, Galindo JCG, Hernández A, Dou J, McChesney JD, Duke SO. 1999. Phytotoxicity of quassinoids: physiological responses and structural requirements. Pesticide Biochem Physiol. 65(1):15–24.
- Elstner EF, Heupel A. 1976. Inhibition of nitrite formation from hydroxylammonium chloride: a simple assay for superoxide dismutase. Anal Biochem. 70(2):616–620.
- Forni C, Cascone A, S. Cozzolino, Migliore L. 2001. Drugs uptake and degradation by aquatic plants as a bioremediation technique. Minerva Biotecnol. 13(2):151–152.
- Galindo JCG, Hernández A, Dayan FE, Tellez MR, Macı́as FA, Paul RN, Duke SO. 1999. Dehydrozaluzanin C, a natural sesquiterpenolide, causes rapid plasma membrane leakage. Phytochemistry. 52(5):805–813.
- Garcia-Galan M, Diaz-Cruz M, Barcelo D. 2011. Occurrence of sulfonamide residues along the Ebro river basin. Removal in wastewater treatment plants and environmental impact assessment. Environ Int. 37:462–473.
- Gong L, Zhang S, Chen D, Liu K, Lu J. 2018. Response of biofilms-leaves of two submerged macrophytes to high ammonium. Chemosphere. 192:152–160.
- Gonzalez PM, Gonzalo S, Rodea PI. 2013. Toxicity of five antibiotics and their mixtures towards photosynthetic aquatic organisms: implications for environmental risk assessment. Water Res. 47(6):2050–2064.
- Hijosa VM, Fink G, Schlusener MP, Sidrach-Cardona R, Martín-Villacorta J, Ternes T, Bécares E. 2011. Removal of antibiotics from urban wastewater by constructed wetland optimization. Chemosphere. 83(5):713–719.
- Hodges DM, DeLong JM, Forney CF, Prange RK. 1999. Improving the thiobarbituric acid-reactive-substance assay for estimating lipid peroxidation in plant tissues containing anthocyanin and other interfering compounds. Planta. 207(4):604–611.
- Jiang M, Zhou Y, Ji X, Li H, Zheng Z, Zhang J. 2019. Responses of leaf-associated biofilms on the submerged macrophyte Vallisneria natans during harmful algal blooms. Environ Pollut. 246:819–826. EnvironmentPollution
- Jiang M, Zhou Y, Wang N, Xu L, Zheng Z, Zhang J. 2019. Allelopathic effects of harmful algal extracts and exudates on biofilms on leaves of Vallisneria natans. Sci Total Environ. 655:823–830.
- Jose OB, Arsalan DD, Vernon BG, Paul B. 2012. Reactive oxygen species and their role in plant defence and cell wall metabolism. Planta. 236(3):765–779.
- Li B, Gu B, Yang Z, Zhang T. 2018. The role of submerged macrophytes in phytoremediation of arsenic from contaminated water: a case study on Vallisneria natans (Lour.) Hara. Ecotoxicol Environ Saf. 165:224–231.
- Li YJ, Wei XX, Chen JW, Xie H, Zhang YN. 2015. Photodegradation mechanism of sulfonamides with excited triplet state dissolved organic matter: a case of sulfadiazine with 4-carboxybenzophenone as a proxy. J Hazardous Mater. 147:305–310.
- Lian J, Qiang Z, Li M, Bolton JR, Qu J. 2015. UV photolysis kinetics of sulfonamides in aqueous solution based on optimized fluence quantification. Water Res. 75:43–50.
- Liu L, Liu Y-h, Liu C-X, Wang Z, Dong J, Zhu G-F, Huang X. 2013. Potential effect and accumulation of veterinary antibiotics in Phragmites australis under hydroponic condition. Ecol Eng. 53:138–143.
- Michael TS, Shin HW, Hanna R, Spafford DC. 2008. A review of epiphyte community development: surface interactions and settlement on seagrass. J Environ Biol. 29(4):629.
- Paul DR, Huang BW, Tsuji Y. 2012. Reactive oxygen species (ROS) homeostasis and redox regulation in cellular signaling. Cell Signal. 2012;24(5):981–90.
- Pinardi M, Bartoli M, Longhi D, Marzocchi U, Laini A, Ribaudo C, Viaroli P. 2009. Benthic metabolism and denitrification in a river reach: a comparison between vegetated and bare sediments. J Limnol. 68(1):133–145.
- Qiu D, Wu Z, Liu B, Deng J, Fu G, He F. 2001. The restoration of aquanticmacrohytes for improving water quality in hypertrophic shallow lake in Hubei Province. China Ecol Eng. 18(2):147–156.
- Qiu JR, Zhao T, Liu QY, He JH, He DC, Wu GY, Li YT, Jiang CG, Xu ZC. 2016. Residual veterinary antibiotics in pig excreta after oral administration of sulfonamides. Environ Geochem Health. 38(2): 549–556.
- Sim W-J, Lee J-W, Lee E-S, Shin S-K, Hwang S-R, Oh J-E. 2011. Occurrence and distribution of pharmaceuticals in wastewater from households, livestock farms, hospitals and pharmaceutical manufactures. Chemosphere. 82(2):179–186.
- Song KY, Zoh KD, Kang H. 2007. Release of phosphate in a wetland by changes in hydrological regime. Sci Total Environ. 380(1–3):13–18.
- Suzuki JY, BollivaR DW, Bauer CE. 1997. Genetic analysis of chlorophyll biosynthesis. Annu Rev Genet. 31(1):61–89.
- Wang C, Zhang S, Wang P, Hou J, Li W, Zhang W. 2008. Metabolic adaptations to ammonia-induced oxidative stress in leaves of the submerged macrophyte Vallisneria natans (Lour.) Hara. Aquat Toxicol. 87(2):88.
- Wang C, Zhang SH, Wang PF, Hou J, Li W, Zhang WJ. 2008. Metabolic adaptations to ammonia-induced oxidative stress in leaves of the submerged macrophyte Vallisneria natans (Lour.) Hara. Aquat Toxicol. 87(2):88–98.
- Wang P, Zhang S, Wang C, Lu J. 2012. Effect of Pb on the oxidative stress and antioxidant response in a Pb bioaccumulator plant Vallisneria natans. Ecotoxicol Environ Safety. 78:28–34.
- Wu Y, Li T, Yang L. 2012. Mechanisms of removing pollutants from aqueous solutions by microorganisms and their aggregates: a review. Bioresour Technol. 53(1):55–62.
- Yang W, Zheng F, Xue X, Lu Y. 2011. Investigation into adsorption mechanisms of sulfonamides onto porous adsorbents. J Colloid Interface Sci. 362(2):503–504.
- Zhang QQ, Yang GG, Pan CG, et al. 2015. Comprehensive evaluation of antibiotics emission and fate in the river basins of China: source analysis, multimedia modeling, and linkage to bacterial resistance. Environ Sci Technol. 49(11):6772–6782.
- Zhang X, Liu Y, Liu Q, Zong B, Yuan X, Sun H, Wang J, Zang L, Ma Z, Liu H, et al. 2018. Nitric oxide is involved in abscisic acid-induced photosynthesis and antioxidant system of tall fescue seedlings response to low-light stress. Environ Exp Botany. 155:226–238.
- Zhou L-J, Ying G-G, Liu S, Zhao J-L, Chen F, Zhang R-Q, Peng F-Q, Zhang Q-Q. 2012. Simultaneous determination of human and veterinary antibiotics in various environmental matrices by rapid resolution liquid chromatography electrospray ionization tandem mass spectrometry. J Chromatogr A. 1244:123–138.