ABSTRACT
Purpose: Retinopathy of prematurity (ROP) is a condition of abnormal retinal vascularization with reduced levels of vascular endothelial growth factor (VEGF) causing vaso-obliteration (Phase I), followed by abnormal neovascularization from increased VEGF (Phase II). We hypothesized that intravitreal pro-angiogenic VEGF-A in microparticle form would promote earlier retinal revascularization in an oxygen-induced ischemic retinopathy (OIR) mouse model.
Materials and Methods: Wildtype mice (39) were exposed to 77% oxygen from postnatal day 7 (P7) to P12. VEGF-A165-loaded poly(lactic-co-glycolic acid) (PLGA) (n = 15) or empty PLGA (n = 14) microparticles were fabricated using a water-in-oil-in-water double emulsion method, and injected intravitreally at P13 into mice right eyes (RE). Left eyes (LE) were untreated. At P20, after retinal fluorescein angiography, vascular parameters were quantified. Retinal VEGF levels at P13 and flatmounts at P20 were performed separately.
Results: VEGF-A165-loaded microparticles had a mean diameter of 4.2 μm. with a loading level of 8.6 weight.%. Retinal avascular area was reduced in VEGF-treated RE (39.5 ± 9.0%) compared to untreated LE (52.6 ± 6.1%, p < 0.0001) or empty microparticle-treated RE (p < 0.001) and untreated LEs (p = 0.001). Retinal arteries in VEGF-treated RE were less tortuous than untreated LE (1.08 ± 0.05 vs. 1.18 ± 0.08, p < 0.001) or empty-microparticles-treated RE (p = 0.02). Retinal arterial tortuosity was similar in the LE of VEGF and empty microparticle-treated mice (P > 0.05). Retinal vein width was similar in VEGF-treated and empty microparticle-treated RE (P > 0.9), which were each less dilated than their contralateral LE (p < 0.01). VEGF levels were higher in P13 OIR mice than RA mice (p < 0.0001). Retinal flatmounts showed vaso-obliteration and neovascularization.
Conclusions: Endogenous retinal VEGF is suppressed in OIR mice. Exogenous intravitreal VEGF-A165-loaded microparticles in OIR mice reduced retinal vaso-obliteration and accelerated recovery from vein dilation and arterial tortuosity. This may be beneficial in preventing Phase II ROP without systemic effects.
Introduction
Retinopathy of prematurity (ROP), a disease of abnormal retinal vascularization in preterm infants, impairs vision and remains the leading cause of childhood blindness in industrialized nations.Citation1 Due to recent advances in neonatal and obstetric care, infants of lower gestational ages at the limit of viability are surviving, thus leading to an increasing incidence of ROP.
Figure 1. Size and in vitro release profile of VEGF-A165-loaded microparticles.
(a) Size distribution of VEGF-A165-loaded microparticles analyzed by DLS. (b) SEM image of VEGF-loaded microparticles. DLS represents dynamic light scattering. The diameter of the VEGF-loaded microparticles was ~4.2 µm. 1000 nm = 1 µm.
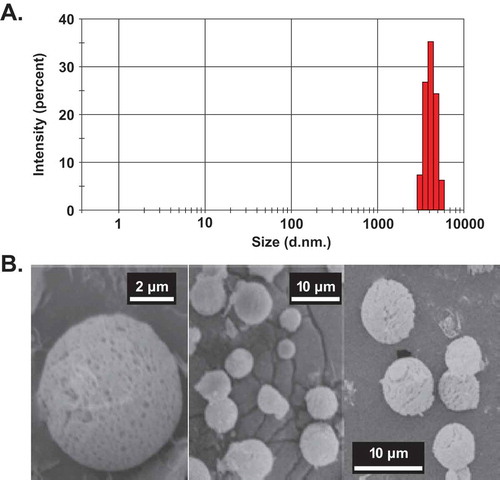
Figure 2. Representative FA images at the phases of retinal vascular development.
RVD represents Retinal Vascular Development. Summary of the major features of representative FA images in the Phases of RVD are shown: Early (P16-20), Mid (P23-27), Late (P30-34), and Mature (>P35).Citation25
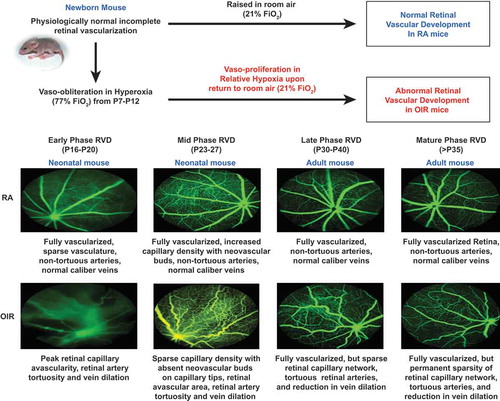
Figure 3. Method of assessment of retinal vascular features.
(a) Fluorescein angiogram (FA) of OIR Mouse. (b) Retinal avascular area – calculated from the binary image generated from the FA image as the area of black space as a percentage of total retinal image. (c) Retinal artery tortuousity (RAT) – calculated by selecting branch points along the most tortuous artery using a cursor, from the base of the optic nerve up to a validated distance of 275 ± 25 μm for OIR mice. A linear projection was automatically projected by the software program to connect the first and last points. RAT index is calculated as the ratio of actual vessel length to the projected linear length. RAT of 1 is a non-tortuous vessel, while an RAT index more than 1 denotes degree of arterial tortuosity. (d) Retinal vein width (RVW) – calculated using semi-automated calibrations between two points aligned horizontally at either edge of the largest vein in the FA image starting from the optic nerve base to a pre-validated distance of 275 ± 25 μm. The customized MATLAB program is available at www.quantbv.com.
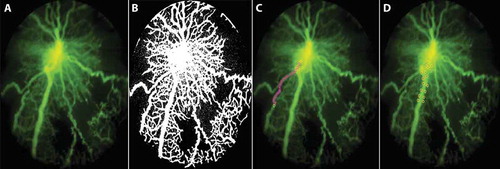
Figure 4. Quantification of in vivo fluorescein angiography in VEGF-loaded and empty microparticles.
Quantification of retinal vascular parameters. A shows FA images, B shows decreased retinal avascular area, C shows reduced vein dilation, and D shows reduced arterial tortuosity.Note: RAA: retinal avascular area (%); RVW: retinal vein width (µm); RAT: retinal artery tortuosity index. All values are presented as mean ± SD.Empty represents empty microparticle injection; VEGF represents VEGF-loaded microparticle injection; RE: right eye; LE: left eye. NS denotes not statistically significant. *p < 0.05 **p < 0.01, ***p < 0.001.
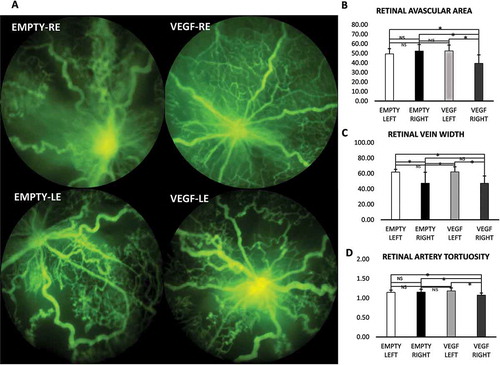
Figure 5. Retinal flatmount and quantification of retinal VEGF levels by ELISA.
Assessment of retinal vaso-obliteration, neovascularization, and VEGF levels during OIR. A. Retinal flatmount of a room air mouse. B. Retinal flat mount showing vaso-oblietration and neovascularization. C. Quantification of Vaso-obliteration or non-perfused retinal area relative to total retinal area. D. Quantification of retinal neovascularization relative to total retinal area. E. Graph shows values for VEGF ELISA at P13 in RA and OIR mice (****p < 0.0001).Note: RA: room air; OIR: oxygen-induced ischemic retinopathy.
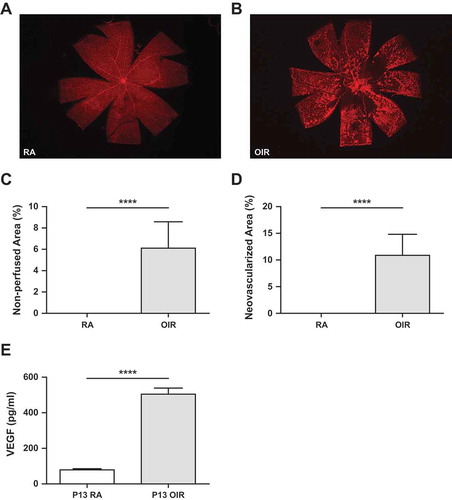
Retinal vascular development (RVD) begins from the fourth month of gestation,Citation2 and ends at term, such that prematurely born infants have incompletely vascularized peripheral retinas. The pathogenesis of ROP involves two phases. Phase 1 (vaso-obliteration) begins at preterm birth with the delay or disruption of retinal vascular growth upon exposure to the relatively hyperoxic ex utero environment.Citation3 With developmental maturation at 4–6 weeks postnatally, the retinal environment, being incompletely vascularized, becomes relatively hypoxic and upregulates production of growth factors, predominantly vascular endothelial growth factor (VEGF). Thereby VEGF leads to abnormal new blood vessel growth (i.e., the Phase 2 ROP (vaso-proliferation)).Citation3 Clinically, Phase 1 ROP occurs from 22 to 30 weeks postmenstrual age (PMA), while Phase 2 ROP occurs from 31 to 44 weeks PMA.
VEGF is a diffusible, homodimeric glycoprotein produced by both normal and cancerous cells in the body,Citation4 known to be a potent angiogenic factor in the pathogenesis of ROP.Citation5 VEGF has several isoforms, with VEGF-A, predominant in the eye, exhibiting both pro-angiogenic (VEGF-A165) and antiangiogenic (VEGF-A165b) splice variants.Citation6–Citation8 Therapies directly or indirectly target excess VEGF reduction in Phase II ROP. Intravitreal injection of the vector-based HIF-1α -targeted shRNA expression system reduced both HIF-expression and retinal neovascularization in vivo.Citation9 Bevacizumab is a humanized nonselective anti-VEGF monoclonal antibody approved by the Food and Drug Administration (FDA) for intravenous use in metastatic colon cancer.Citation4 Bevacizumab has been adapted in the past decade, as an off-label treatment for neovascular ocular diseases like diabetic retinopathy, age-related macular degeneration, and in Phase 2 ROP, either for monotherapy or in conjunction with laser therapy,Citation10 and remains the only current clinical pharmacologic therapy for ROP. Potential risks of intravitreal anti-VEGF therapy in ROP relate to systemic absorption and blocking of all VEGF isoforms, which could be detrimental for developing organs. VEGF is required for vascular growth in developing lungs, brain, liver, and kidneys. Furthermore, there is a risk of increased intraocular pressure following anti-VEGF therapy.Citation11 Different therapies, such as, retinoic acid Citation12 and the inhibition of prolyl hydroxylase,Citation13 have been used in Phase I ROP to reduce vaso-obliteration and thereby reduce the ischemia-mediated retinal neovascularization in Phase II ROP. Challenges in treating vitreoretinal diseases with topical and systemic pharmacological methods are due to the fluid-dynamic properties of the anterior segment structures and drainage, as well as the anterior flow of the aqueous humor, blood-aqueous barrier, and blood-retinal barrier that limit drug diffusion and penetration into the retina.Citation14 Hence, there is a need for drug delivery options that limit systemic absorption when treating retinal diseases such as ROP. Use of pro-angiogenic agents that could indirectly stimulate VEGF, like erythropoietin,Citation15,Citation16 were reported to be unsuccessful in preventing ROP and may have increased the risk of ROP, likely because of timing during the vaso-proliferative phase. Targeted drug delivery specifically during vaso-obliteration prior to the neovascular phase would avoid excess VEGF stimulation.
Advanced drug delivery systems can provide sustained release at target sites, potentially reduce the need for repeat dosing, and avoid undesired systemic effects.Citation14 Different polymer-based nano- and micro-particles have been explored for intraocular drug delivery to achieve sustained drug release.Citation17–Citation19 Poly (lactic-co-glycolic acid) (PLGA) is a biocompatible and biodegradable polymer approved by the FDA for human clinical applications. It breaks down into lactic acid and glycolic acid through hydrolytic degradation. PLGA microparticles have been used to deliver pro-angiogenic VEGF-A165 to stimulate vasculogenesis, angiogenesis, and tissue remodeling in an acute myocardial ischemia-reperfusion rat model.Citation20 Judiciously designed PLGA microparticles (e.g., via control of molecular weight, ratio of lactic to glycolic acid, and fabrication process) can achieve the controlled release of proteins ranging from several days to months.Citation21,Citation22
Oxygen-induced ischemic retinopathy (OIR) models are optimal for ROP studies because newborn mice and rats have incomplete RVD at birth similar to preterm infants. Mouse OIR involves exposing newborn mice to hyperoxia shortly after birth from postnatal day (P) 7 to P12, mimicking the vaso-obliterative phase of ROP. Upon return to room air, relative hypoxia occurs triggering neovascularization, mimicking the vaso-proliferative phase of ROP. Ex vivo models of OIR are informative for comparing areas of vaso-obliteration and vaso-proliferation at about P17.Citation23,Citation24 In our recently described innovative in vivo method of assessing OIR vascular outcomes using fluorescein angiography, unique retinal vascular features (retinal vascular area (RVA), retinal vein width (RVW), and retinal artery tortuosity (RAT)) were shown to be reliable biomarkers of disease progression.Citation25 Retinal VEGF levels decrease during hyperoxia exposure, with a steady increase upon return to room air from P12 onwards. At P13, VEGF mRNA levels in OIR mice had a 4-fold increase compared to age-matched room air mice, then peaked within 12 h in hypoxia and remained elevated for about 14 days; meanwhile, VEGF remained elevated during the development of neovascularization.Citation26 It appears that despite the high circulating endogenous P13 VEGF level in the retina, there is a functional delay or inhibition of its angiogenic actions on retinal vessels, since avascularity persists in ex vivo flat mounts at P13 to P20. This led us to propose that administration of endogenous pro-angiogenic VEGF at P13 could rescue or potentiate the activity of endogenous VEGF in OIR mice. Human VEGF protein, VEGF165, is one residue longer than the murine homologue VEGF164. Studies have shown that VEGF164(165) have similar mechanisms of action and both bind avidly to the VEGFR2 receptor.Citation27,Citation28 Both pro-angiogenic VEGF165 and its endogenous inhibitory isoform, VEGF165b, bind VEGFR-2 with the same affinity, but do not activate it or stimulate their separate downstream signalling pathways.Citation29 While human recombinant VEGF165 has been used in OIR,Citation30 our incorporation of VEGF in microparticle form for intravitreal injection is novel.
We demonstrated in this study that a retinal-specific, pro-angiogenic form of VEGF-A (VEGF-A165), if given early in OIR mice during the vaso-obliterative phase of ROP, would promote earlier revascularization of the retina.
Materials and methods
Materials
PLGA (50:50, inherent viscosity 0.15–0.25/dL/g, molecular weight (MW): 10,000 g/mol) was obtained from Durect Corporation (Pelham, AL, USA). Dichloromethane (DCM) and dimethyl sulfoxide (DMSO) were obtained from Alfa Aesar (Tewksbury, MA, USA). Recombinant human vascular endothelial growth factor-A165 (VEGF- A165) was obtained from R&D Systems (Minneapolis, MN, USA).
VEGF-A165-loaded microparticle fabrication
VEGF-A165-loaded PLGA microparticles were fabricated using a water-in-oil-in-water (w/o/w) double emulsion method.Citation31,Citation32 Briefly, VEGF-A165 (10 μg) was dissolved in 10 μL of phosphate buffer saline (PBS, 10 mM) with 4% polyvinyl alcohol (PVA), and PLGA (100 μg) was dissolved in 100 μL of DCM. The as-prepared VEGF-A165 solution (1 μg/μL in PBS) and PLGA solution (1 μg/μL in DCM) were emulsified using a bath sonicator for 10 s. The resulting emulsion (w/o) was mixed with 0.5 mL PBS with 1% PVA and again emulsified via vortex for 30 s to prepare the secondary emulsion (w/o/w). The double emulsion was poured into 5 mL PBS and mixed thoroughly using a magnetic stirrer at 800 rpm for 6 h to evaporate the organic solvent. The hardened microparticles were centrifuged (3000 × g, 15 min) and then washed with de-ionized (DI) water three times to remove the free PVA. The rinsed microparticles were lyophilized for 3 days and then stored at –20°C.
Microparticle characterization
The hydrodynamic size and size distribution of the VEGF-A165-loaded PLGA microparticles were characterized by a dynamic light scattering (DLS) spectrometer (Malvern Zetasizer Nano ZS, MA, USA) at a 90° detection angle (). The concentration of the microparticles used for the DLS measurement was 0.1 μg/μL. The morphology of the dried microparticles was studied by scanning electron microscope (SEM) ().
In vitro drug release study
The loading content of the VEGF-A165-loaded PLGA microparticles was studied by dissolving the microparticles (50 μg) in 10 μL of dimethyl sulfoxide, followed by the addition of 10 μL of DI water. The VEGF-A165 concentration in the solution was determined by a NanoDrop Microvolume Spectrophotometer (Thermo Fisher Scientific, MA, USA). To study the release profile of the microparticles, an accurately weighed amount of VEGF-loaded PLGA microparticles (100 μg) were dispersed in 50 μL of PBS. The microparticle suspension was incubated at 37°C under constant shaking. At several timepoints (24 h, 48 h, 72 h, 96 h, 120 h, and 144 h), the microparticle suspension was centrifuged (5,000 rpm, 15 min). A quantity of 30 μl of supernatant was removed and 30 μl of fresh PBS was added to resuspend the microparticles. The VEGF content in the supernatant was determined by bicinchoninic acid (BCA) protein assay.
Animals and OIR
Animals used for this study were C57BL/6J mice (Jackson Laboratory, Bar Harbor, ME, USA) obtained from breeding colonies maintained according to approved protocols by the Institutional Animal Care and Use Committee of the University of Wisconsin School of Medicine and Public Health, and were in compliance with the Association for Research in Vision and Ophthalmology Statement for the Use of Animals in Ophthalmic and Vision Research. Neonatal mice (n = 39) were exposed to 77 ± 2% oxygen from P7 to P12 (OIR mice) in an oxygen chamber (Biospherix ProOX 110; Apex lab, Redwing, MN, USA) with their nursing mothers, before returning to room air to induce relative hypoxia, as in our published modificationCitation25,Citation33 of an existing mouse model of OIR.Citation34 The slightly higher range of FiO2 (77 ± 2%) generates more visible vascular features of OIR during in vivo imaging (unpublished observation). Pups from five different litters were used in this study to avoid inter-litter variability. Each litter had different paternal and material mice. At P13, intravitreal injections were performed. At P20, representing the early phase of RVD, see , mice underwent in vivo retinal fluorescein angiography (FA). In a separate experiment, two cages of 6 mice each were subjected to OIR. Mice from one cage underwent retinal extraction for VEGF enzyme-linked immunosorbent assay (ELISA) (n = 6) and the second cage was used for retinal wholemount staining to compare neovascularization and vaso-obliteration.
In vivo drug delivery and imaging
Intravitreal injection
Following OIR, as described above, in a random fashion, the mice received intravitreal injections of VEGF-A165-loaded (n = 15) or empty (n = 14) PLGA microparticles to their right eyes (RE) on P13 (vaso-obliteration stage). The left eyes (LE) of each mouse were controls. In brief, a mouse was anesthetized through intraperitoneal (IP) injection of ketamine and xylazine and placed on a heated pad (37°C). The RE was visualized under a dissecting microscope and the skin around the eye was cleansed with an ethanol soaked cotton pad. An aperture of 1 mm, posterior to the superotemporal limbus, was made with a 30-gauge needle lancet. Then, a 33-gauge Hamilton needle was inserted through the incision, and the needle advanced parallel to the outer eye wall at the limbal margin of the cornea and the sclera, directed towards the vitreous. The prepared microparticle injection (0.5 μL of 1 ng of VEGF protein per eye) was given over 30 s to allow for diffusion, taking care to avoid damaging the lens. A 5-second pause followed the injection before slowly removing the needle. Topical bacitracin antibiotic ointment was administered to the eye after injection, and continued daily for 4 days. Out of 39 mice, 29 VEGF-A165-loaded (n = 15) or empty (n = 14) PLGA microparticle-injected mice were used for analysis. Ten mice developed cataracts during prolonged imaging that affected the quality of the FA images obtained, and were excluded from analysis. Two of the ten mice were observed during imaging to have significant hematomas, likely inadvertently from the intravitreal injection, that obscured fundus views, and were excluded in the analysis.
In vivo FA
At P20, a micron IV retinal imaging system (Phoenix Research lab, California) was used to perform FA according to the manufacturer’s image acquisition software. The pupils were dilated with 1% tropicamide (Bausch + Lomb, Inc., Tampa, FL) and the mouse placed on a heated stage (37°C) where 10% sodium fluorescein (50 mg/kg) (AK-FLUOR, Akron, Decatur, IL) was injected intraperitoneally. Fluorescent images were obtained in central, superior, nasal, and temporal retinal quadrants. RE images were obtained first in some mice, followed by LE, while an alternating equal number of the opposite order was used in others to ensure an even image quality for both eyes since fluorescein pooling and blurring can occur with delayed imaging of the contralateral eye. VEGF-RE is the RE that was treated with the VEGF-loaded PLGA microparticles. VEGF-LE is the untreated contralateral LE in the same mouse, whose RE is VEGF-RE. Empty-RE is the RE that was treated with the empty PLGA microparticles. Empty-LE is the untreated contralateral LE in the same mouse, whose RE is empty-RE.
Quantification of retinal vascular parameters
RVA, RVW, and retinal arterial tortuosity (RAT) were quantified from selected fluorescent angiograms, and automated and semi-automated customized software protocols, which were developed using MATLAB (Mathworks, Natick, MA), and are publicly available at www.quantbv.com.Citation25,Citation35 RVA quantifies the retinal area covered with vessels (major vessels and capillaries). To quantify capillary density during retinal revascularization after hyperoxia, the percent RVA or RAA was used, which is the reverse of the percent RVA (mathematically (100 – RVA)), see . In brief, a retinal FA image with a centrally located optic nerve was uploaded into the customized program and was automatically converted to a gray-scale image and then to a binary image. The degree of avascularity (empty spaces or black areas not occupied by vessels) in the retinal images represents the RAA as a percentage (%) of the total retinal area in the circular FA image (percent RAA). A separate FA image with a peripherally located optic nerve was used to determine both RVW and RAT as previously described.Citation25 RAT quantifies the degree of tortuosity in OIR mice, which has been shown to decrease with increasing age but persist in adult mice. RVW quantifies the degree of dilation of the veins, which is transient in neonatal mice but returns to normal width in adult mice.
VEGF level measurements
The VEGF levels were evaluated in retina extracts prepared from P13 mice under room air or OIR conditions using the Mouse VEGF Immunoassay Kit as recommended by the supplier (R&D Systems, Minneapolis, MN). Briefly, retinas from each mouse were dissected and snap frozen in 0.5 mL of PBS and stored at −80°C prior to analysis. At the time of the assay, the samples were removed from −80°C and subjected to two cycles of freeze-thaw followed by a brief sonication. The lysates were then centrifuged for 15 min at 4°C; the supernatants were transferred to a clean tube and used in the assay.
Preparation, visualization, and analysis of retinal wholemounts
Mice were sacrificed at indicated time points during OIR. The mice eyes were enucleated, fixed in 4% paraformaldehyde briefly (3–5 min), transferred to 70% methanol, and fixed for at least 24 h at −20ºC. Retinas were dissected and washed with phosphate buffered saline (PBS) three times, 10 min each. Dissected retinas were then incubated in blocking buffer (50% fetal calf serum (FBS) and 20% normal goat serum (NGS) in PBS) for 2 h at room temperature (RT). Retinas were incubated with the primary antibody, rabbit anti-mouse collagen IV (Millipore, AB756P) diluted 1:500 in PBS containing 20% FCS, 20% NGS at 4ºC overnight. Retinas were then washed three times with PBS, 10 min each; incubated with appropriate secondary antibody, Alexa 594 goat-anti-rabbit (A-11037; ThermoFisher) diluted 1:500 in PBS containing 20% FCS, 20% NGS for 2 h at RT. Retinas were then washed four times with PBS, 10 min each and mounted on the slide with PBS/glycerol (1:1 vol). Retinas were viewed by fluorescence microscopy and images were captured using EVOS imaging system (AMG, ThermoFisher) or Zeiss microscope (AxioPhot, Carl Zeiss, Chester, VA). The quantification of retinal and vitreous neovascularization during OIR was performed as described previously.Citation36 For image analysis, after wholemount staining and imaging, retinal neovascularization was assessed by a semi-automated quantification method (SWIFT_NV) installed on ImageJ software (NIH, Maryland, USA) as developed and described by Stalh et.al.Citation36 During the preparation of digital captured images for these macros, avascular areas were also measured and reported as percentage of vaso-obliterated area relative to the total retina area.
Statistical analysis
Results are presented as the mean ± standard deviation (SD). Two-way analysis of variance (ANOVA) with post-hoc analysis using Tukey’s multiple comparisons for pair-wise comparisons were used to determine the difference between RAA, RVW, and RAT at P20 between the RE (injected eye) and the LE (control eye) in each mouse, and between VEGF-loaded and empty microparticles at P20. Statistical significance was set at p < 0.05. Statistical analyses were conducted using SAS 9.4.
Results
Properties of PLGA microparticles
Particle size distribution
The hydrodynamic diameters of the microparticles averaged 4.2 μm, as measured by DLS (). The size and morphology of the microparticles were studied by SEM (Figure 1b). The microparticles were spherical in structure, with a diameter ranging from 3 to 8 μm.
In vitro drug release
VEGF-loaded microparticles were used for the VEGF loading and in vitro release studies (). The VEGF-A165 loading content of microparticles was 8.6 wt%, with a high loading efficiency of 95%. An in vitro protein release profile was studied by a BCA assay. A burst release within the first 24 h was observed. At 12 h and 24 h, 35% and 48% of protein was released from the microparticles, respectively. By 72 h, 74% of the VEGF-loaded microparticles were released. From this point, the release curve reached a plateau in which only 2% of the protein was released from 72 h to 144 h.
Microparticle effect on retinal vascularization
RVA was more reduced in VEGF-treated eyes compared to all controls
The mice with RE treated with VEGF-loaded microparticles (VEGF-RE) exhibited less RAA than VEGF-LE (39.50 ± 9.0% vs. 52.60 ± 6.1%, p < 0.0001) of the same mouse. VEGF-RE mice had less avascular area or were more vascularized than age-matched mice injected with empty microparticles (empty-RE) in the same cage (39.50 ± 9.0% vs. 52.43 ± 6.8%, p < 0.001). VEGF-RE mice also had less avascular area than the empty microparticle untreated LE (empty-LE) (39.50 ± 9.0% vs 49.50 ± 5.4%, p = 0.001). Empty-RE mice did not differ from empty-LE (52.43 ± 6.8% vs. 49.50 ± 5.4%, p = 0.37). Retinas in VEGF-RE were also more vascularized than empty-LE (p = 0.0001). RAA in empty-LE and VEGF-LE did not differ (p = 0.25) (Figure 4a,b).
VEGF-treated eyes and empty microparticle-injected eyes had a reduction in RVW
Retinal veins were less dilated in VEGF-RE compared to the contralateral LE of the same mouse (VEGF-LE) (47.29 ± 9.5 µm vs. 62.12 ± 6.7 µm, p < 0.0001). VEGF-RE retinal veins were much smaller in width than the retinal veins of the empty-LE (47.29 ± 9.5 µm vs. 61.9 ± 3.6 µm, p < 0.001). RVW did not differ between VEGF-RE and empty-RE (47.29 ± 9.5 µm vs. 47.5 ± 14.2 µm P > 0.97). RVW in empty-RE was smaller than VEGF-LE (p < 0.001). Empty-RE-injected eyes also had retinal veins smaller than untreated empty-LE (p < 0.001). However, RVW did not differ between VEGF-LE and empty-LE (p = 0.95) ().
The retinal arteries in VEGF-treated eyes were less tortuous than all controls
There was a significant difference in the retinal tortuosity index in the VEGF-RE-treated eyes compared to the contralateral untreated LE of the same mouse (VEGF-LE), (1.08 ± 0.05 vs. 1.18 ± 0.08, p < 0.001). VEGF-loaded microparticle-treated RE had less tortuous retinal arteries than empty microparticle-injected RE (empty-RE) in comparison mice (1.08 ± 0.05 vs. 1.15 ± 0.07, p = 0.02). The RAT index was reduced in VEGF-RE compared to empty-LE (1.08 ± 0.05 vs. 1.15 ± 0.06, p = 0.02). The RAT index did not differ between empty-RE and empty-LE (p = 0.8). The retinal arteries of both VEGF-LE and empty-RE did not differ in tortuousity (p = 0.8) There was no difference between empty-LE and VEGF-LE (p = 0.15) ().
Retinal wholemounts showed vaso-obliteration and neovascularization
At P20, the retinal flat mount showed normal retinal vascularization in the room air mice. The OIR mice showed central to mid-peripheral vaso-obliteration and peripheral areas of neovascularization. When quantified, the area of vaso-obliteration (26,801 ± 4041, n = 8 vs. 0.1667 ± 0.1667, n = 6, p < 0.0001) and neovascularization (47,504 ± 6088, n = 8 vs 0.1667 ± 0.1667, n = 6, p < 0.0001 was increased in OIR compared to RA mice, ().
VEGF levels were increased in OIR mice post-hyperoxia
VEGF Levels were higher in pooled retina (n = 6) from OIR mice at P13. They had significantly higher VEGF levels than pooled retina from P13 RA mice (n = 6), (502.5 ± 36 pg/ml vs 79.8 ± 4.0 pg/ml (p < 0.0001; )
Discussion
This study is the first demonstration of the use of intravitreal pro-angiogenic VEGF-A165 encapsulated in PLGA microparticles for sustained release in order to promote earlier revascularization of the retina for treatment of Phase I ROP. Clinically, no known drug therapies are available to treat the vaso-obliterative Phase I ROP in at-risk premature infants. The available therapy for treatment of proliferative Phase II ROP is a universal anti-VEGF monoclonal antibody prompting concerns for systemic toxicity to the eye and other developing organs. Intravitreally administrated VEGF-A165 microparticles, used in this study, were also shown to treat other aspects of the ROP vascular phenotype, arterial tortuosity and venous dilation (akin to human plus disease). Our novel application of VEGF-loaded microparticles as a treatment for early Phase 1 ROP has the potential to limit or prevent systemic toxicity while being beneficial for accelerated revascularization of retinal vessels.
The P17 is the commonly accepted time point for ex vivo OIR assessments, as it demonstrates both vaso-obliteration and “neovascularization” prior to the onset of natural regression of OIR around P20.Citation24 However, in vivo assessments show no significant difference in the degree of vaso-obliteration and “neovascularization” or revascularization following recovery from hyperoxia exposure during the Early Phase of RVD (P16-20).Citation25 A central hyper-fluorescence is noted around the optic nerve at P16,Citation25 likely from increased vascular permeability and abnormal barrier function post-hyperoxia that decreased in prominence with increasing developmental age from P17 to P20. Therefore, in order to avoid overestimating RVA quantitation from fluorescein pooling, P20 was chosen in this study as the optimal time point for in vivo FA assessments in the early phase. Our finding of 45–54% RVA in OIR mice at P20 is consistent with prior in vivo studies.Citation25 There was a 75% increase in the area of retinal revascularization following VEGF microparticle treatment. While ex vivo OIR studies note that retinal neovascularization is well established at P15,Citation37 with maximal pathological neovascularization at P17,Citation24 full peripheral revascularization from P19 to P25, and spontaneous regression of neovascularization between P17 and P25,Citation24 in vivo FA imaging shows maximal neovascularization at P18 to P19, full peripheral revascularization between P30 to P34, and significant arterial tortuosity and reduced capillary density in OIR compared to RA mice persisting beyond sexual maturity of the mice (>P35).Citation25 The “neovascularization” or clusters of neovascular tufts observed during ex vivo wholemounts are not at all seen during FA imaging in live mice, and could be in part due to the fluorescein pooling from leaky vessels observed in late angiography. Rather, the “neovessel tufts” seen in flatmounts appear to be actual points of peripheral arborization, revascularization, or an increase in density of the retinal capillary network, more aptly quantified by RVA, using the customized MATLAB program. Our ex vivo retinal flatmounts showed the presence of neovascularization and vaso-obliteration consistent with other OIR studies.Citation24,Citation36 Therefore, the administration of pro-angiogenic VEGF in this study is effective in promoting or accelerating revascularization in the OIR mice, not necessarily reducing retinal neovascularization. While the VEGF-A165-loaded microparticle-treated eyes showed increased revascularization, the capillary networks in-between major vessels were still sparse and less dense than age-matched room-air mice. Retina from room-air mice at P20, from our previous studies,Citation25 have a relatively densely packed intricate network of capillaries in-between major arteries and veins, with the presence of hyperfluorescent neovascular buds at the capillary tips, likely evidence of physiologic developmental angiogenesis. Longitudinal studies of VEGF-A165-loaded microparticle treatment at later time points beyond P35 (age of sexual maturity of mice) will be helpful to determine whether full regeneration of capillary networks occurs after P20.
Our study shows that VEGF level is higher in P13 OIR mice than RA mice. This is consistent with Shen et al.’s finding of increased VEGF levels in mice retinas following OIR.Citation38 It appears that endogenous VEGF levels are increased following hyperoxia, despite the marked phenotype of retinal avascularity, indicating that most likely the functional activty of endogenous VEGF is reduced or suppressed compared to the functional activity of endogenous VEGF in RA mice. Pharmacologic agents have been employed previously, both in vitro and in vivo, to promote retinal revascularization. Using tumor necrosis factor (TNF-α) knockout mice, Gardiner et al. showed that the absence of TNF-α from P12 to P17 promoted revascularization of ischemic areas in OIR mice, with increased angiogenesis and reduced pathological pre-retinal neovascularization.Citation39 A combined study using in vitro and in vivo low-dose simvastatin in retinal microvascular endothelial cells and OIR mice, showed decreased avascular area, thereby preventing pathological neovascularization.Citation40 Dimethyloxalylglycine, an inhibitor of prolyl hydroxylase, was injected systemically at P6 and P8 to stabilize HIF-1, and resulted in reduced capillary dropout, decreased vascular tortuosity, but did not have a protective effect on OIR pathology when given at P11 and P13.Citation13 Trolox C, a vitamin E analog with antioxidant activity, when given to OIR rats, reduced RVAs by 43%, decreased vaso-obliteration, and increased capillary density, compared to controls,Citation41 like our study. Unlike our intravitreal microparticle VEGF injections, these agents were delivered systemically. Intravitreal application of pharmaceutical agents in OIR mice have been previously employed, mainly using antiangiogenic agents for Phase II OIR. Intravitreal administration of bevacizumab in OIR mice significantly reduced neovascularization in quantitative endothelial nuclei counting of the nerve fiber layer and ganglion cell layer.Citation42 The higher the degree of retinal vaso-obliteration in Phase I ROP the greater the severity of retinal hypoxia in Phase II ROPCitation12; therefore, Phase I therapies have been studied. Wang et al. increased VEGF levels during hyperoxia exposure by giving retinoic acid intraperitoneally from P6 to P9, thus promoting retinal revascularization and preventing the subsequent hypoxia-induced retinal neovascularization caused by excess VEGF.Citation12 Konopatskya et al. injected a single antiangiogenic human recombinant human VEGF-A165b intravitreally at P13, like our study, showing a reduction in preretinal neovascularization without inhibition of physiological intraretinal angiogenesis.Citation30 Intravitreal injection of VEGF prior to hyperoxia exposure in an adult or neonatal OIR rat model prevented vessel regression by accelerating pericyte maturation,Citation43 but the VEGF isoform given was not specified, and is presumed to be nonselective for pro- or antiangiogenic isoforms. While our use of exogenous pro-angiogenic VEGF-A165 at P13 right after the hyperoxic phase, may increase the retinal levels of VEGF following its down-regulation in the hyperoxic phase (P7-12), it serves primarily to enhance the functional angiogenic activity of VEGF, given that avascularity persists between P13 and P17, despite increasing levels of endogenous VEGF in relative hypoxia. The mechanism by which exogenous VEGF stimulates angiogenesis in OIR mice despite a delay in activity of the excess endogenous VEGF was beyond the scope of this study, and requires further exploration.
Microparticles have previously been used to deliver pharmacologic agents intraocularly.Citation19,Citation44,Citation45 Since proteins typically are relatively large and unstable in vitro and in vivo, the design of microparticles for a controlled protein delivery system is critical.Citation46,Citation47 PLGA microparticles are used for protein delivery vehicles Citation31,Citation48 for the following reasons: (1) PLGA polymers are biocompatible and biodegradable and FDA approved for human clinical use, (2) the PLGA microparticle morphology can be controlled by modifying the fabrication process and chemical structure, and thus (3) release profiles of proteins in PLGA microparticles can be controlled from days to months. PLGA has been used as a vehicle for the intraocular delivery of drugs to treat retinal degenerationCitation49 and ocular surface disease.Citation21 VEGF-loaded PLGA microparticles previously promoted revascularization in rat models of myocardialCitation20 and brain ischemia.Citation50 In this study, we controlled the microparticle size to 3–8 μm, and achieved a moderate protein loading content of 8.6 wt.% with a high loading efficiency of 95%. The release profile of VEGF from PLGA microparticles is a burst release in the first 24 h, followed by a sustained release until 72 h when three-fourths of our VEGF was reached, coinciding with peak neovascularization in the OIR model at P15. Prolonged delivery of VEGF could exacerbate pathologic neovascularization in Phase II ROP, so achieving release of most of the VEGF microparticles by 72 h in Phase I ROP is optimal.
Our delivery of proangiogenic VEGF-A165 microparticles into the vitreous demonstrated a limited systemic effect. This can only be inferred from the observation that the untreated contralateral LE of the same mouse with a VEGF- A165-loaded microparticle-treated RE had similar RVAs to control LE in mice that received empty microparticles to their RE, since our study did not include measurements of systemic levels of VEGF-A165. Bevacizumab, being a nonselective anti-VEGF antibody inhibits the binding of all isoforms of VEGF (A, B, C, D, E) to their receptors present in other developing organs, raising concerns of systemic adverse effects in premature infants. Selective pro-angiogenic VEGF-A isoforms have been used to induce angiogenesis in clinical and experimental studies of myocardialCitation51,Citation52 and brain ischemiaCitation53; however, VEGF-A165-loaded microparticles have not been used in OIR experimentally or in clinical ROP therapy. Our specific use of pro-angiogenic isoform of VEGF-A165, rather than the antiangiogenic isoform VEGF-A165b not only limits the risk of unwanted effects on other organs, but also ensures only pro-angiogenic actions on the retinal vasculature.
Our study indicates that early VEGF-A165 treatment in at-risk premature infants may promote physiologic revascularization and potentially prevent severe or proliferative ROP. We showed that VEGF-A165-loaded PLGA microparticle treatment not only accelerated retinal vascular regeneration in OIR mice, but also reduced venous dilation and arterial tortuosity. Retinal arterial tortuosity and venous dilation could be potential objective markers of ROP progression and recovery. RAT is a more reliable marker than RVW, because while RVW is variable in OIR mice and transiently dilated in the early phase RVD, the arteries are consistently more tortuous, decreasing in tortuosity with increasing developmental age and persisting in adult OIR mice despite full retinal vascularization (Figure 2). Our study’s incidental findings of reduced RVW in the empty-PLGA-injected eyes compared to the LE controls in the same animals and the VEGF-LE could be that the PLGA component of the microparticles may have a positive effect on retinal veins, independent of VEGF. Empty microparticles did not otherwise impact revascularization or RAT. The degradation of the PLGA microparticle released free lactate, which has been shown to promote vascular regeneration in tissues and wound healing through hypoxia-inducible factor-activation and stimulation of pro-angiogenic growth factor release from endothelial cells,Citation54–Citation56 and may cause release of macrophage VEGF. Further exploration of the selective effects of PLGA on retinal veins would be helpful in understanding its microparticle retinal effects, but was beyond the scope of our current study.
Future studies to optimize the pharmacokinetics of VEGF-A165 microparticle delivery, protein loading content, and loading efficiency to achieve peak release within 24 h of injection may potentiate its vascular effects, and promote earlier revascularization or normalization of the retinal capillary density and arterial tortuosity. A limitation of our study was the absence of comparative data at the time of injection at P13. This is because the mice eyes are mostly fused at P13, thus in vivo imaging is not feasible at this early developmental age. In a potential clinical translation of our study, a newborn mouse is equivalent in gestational maturity to a 24-week-old infant and P12 – P13 mice are comparable to a term newborn infant,Citation57 so technically administering pro-angiogenic VEGF165a at this time may potentially exacerbate exisiting ROP. Therefore, VEGF-A165 microparticles would ideally be administered during Phase I ROP in the human for maximal revascularization effect prior to established proliferative ROP disease. Our use of pro-angiogenic VEGF at P13 shows that it can be administered early in Phase II ROP (from 31 weeks) up to 37–38 weeks (term) postconception to promote retinal revascularization without exacerbating proliferative retinopathy. Thus, the ideal clinical timing for administering VEGF-A165 would be prior to the first ROP exams, which normally occurs at 31 weeks postconception or 4–6 weeks after birth. This would avoid treating already fragile premature infants right after birth, since only a limited number would progress to developing severe ROP, thereby reserving treatment to the most at-risk infants with early signs of proliferative disease. Nonetheless, exploring the impact of intravitreal VEGF-A microparticle injections during peak vaso-obliteration and exploring the associated molecular mechanisms and histological effects needs further exploration. In conclusion, the use of VEGF-loaded microparticles, in a sustained release form shortly after hyperoxia exposure accelerated retinal vascular remodelling with the potential for limited systemic absorption. This method could be useful in the prevention of and earlier treatment of ROP before pathological neovascularization occurs or promote recovery from plus disease in humans. Further studies on the mechanism of action of exogenous pro-angiogenic VEGF-A165 would be paramount to advancing ROP treatment strategies.
Financial Support
University of Wisconsin Department of Pediatrics and School of Graduate Studies (to Mezu-Ndubuisi); NIH 1K25CA166178 and R01 HL129785 (to Gong). The work in NS lab is supported by an unrestricted award from Research to Prevent Blindness to the Department of Ophthalmology and Visual Sciences, Retina Research Foundation, P30 EY016665, EPA 83573701, and EY026078. NS is a recipient of RPB Stein Innovation Award.
Acknowledgments
The authors thank Ellen R. Wald, MD (Department of Pediatrics, University of Wisconsin) and Pam Kling, MD (Department of Pediatrics, University of Wisconsin) for their review of the manuscript. The authors also thank Nasim Jamali, PhD and Andrew Suscha for their assistance with retinal ELISA and flatmount studies.
Disclosure statement
No potential conflict of interest was reported by the authors.
Additional information
Funding
References
- Gilbert C. Retinopathy of prematurity: A global perspective of the epidemics, population of babies at risk and implications for control. Early Hum Dev. 2008;84:77–82. doi:10.1016/j.earlhumdev.2007.11.009.
- Roth AM. Retinal vascular development in premature infants. Am J Ophthalmol. 1977;84:636–40.
- Smith LEH. Pathogenesis of retinopathy of prematurity. Semin Neonatal. 2003Dec 1;8:469–73. doi:10.1016/S1084-2756(03)00119-2.
- Iqbal S, Lenz HJ. Integration of novel agents in the treatment of colorectal cancer. Cancer Chemother Pharmacol. 2004 Sep;54(Suppl 1):S32–9. doi:10.1007/s00280-004-0884-0.
- Smith LE, Shen W, Perruzzi C, Soker S, Kinose F, Xu X, Robinson G, Driver S, Bischoff J, Zhang B, et al. Regulation of vascular endothelial growth factor-dependent retinal neovascularization by insulin-like growth factor-1 receptor. Nat Med. 1999 Dec;5:1390–95. doi:10.1038/70963.
- Zhao M, Shi X, Liang J, Miao Y, Xie W, Zhang Y, Li X. Expression of pro- and anti-angiogenic isoforms of VEGF in the mouse model of oxygen-induced retinopathy. Exp Eye Res. 2011;93:921–26. doi:10.1016/j.exer.2011.10.013.
- Neufeld G, Cohen T, Gengrinovitch S, Poltorak Z. Vascular endothelial growth factor (VEGF) and its receptors. FASEB J. 1999 Jan 1;13(1):9–22. doi:10.1096/fasebj.13.1.9.
- Bates DO, Cui TG, Doughty JM, Winkler M, Sugiono M, Shields JD, Peat D, Gillatt D, Harper SJ. VEGF165b, an inhibitory splice variant of vascular endothelial growth factor, is down-regulated in renal cell carcinoma. Cancer Res. 2002 Jul 15;62:4123–31.
- Xia XB, Xiong SQ, Xu HZ, Jiang J, Li Y. Suppression of retinal neovascularization by shRNA targeting HIF-1alpha. Curr Eye Res. 2008 Oct;33:892–902. doi:10.1080/02713680802416670.
- Mintz-Hittner HA, Kennedy KA, Chuang AZ. Efficacy of intravitreal bevacizumab for stage 3+ retinopathy of prematurity. New England J Med. 2011;364:603–15. doi:10.1056/NEJMoa1007374.
- Bracha P, Moore NA, Ciulla TA, WuDunn D, Cantor LB. The acute and chronic effects of intravitreal anti-vascular endothelial growth factor injections on intraocular pressure: A review. Surv Ophthalmol. 2018 May–Jun;63(3):281–295. doi:10.1016/j.survophthal.2017.08.008. Epub 2017 Sep 5.
- Wang L, Shi P, Xu Z, Li J, Xie Y, Mitton K, Drenser K, Yan Q. Up-regulation of VEGF by retinoic acid during hyperoxia prevents retinal neovascularization and retinopathy. Invest Ophthalmol Vis Sci. 2014 Jul;55:4276–87. doi:10.1167/iovs.14-14170.
- Sears JE, Hoppe G, Ebrahem Q, Anand-Apte B. Prolyl hydroxylase inhibition during hyperoxia prevents oxygen-induced retinopathy. Proc Natl Acad Sci U S A. 2008 Dec 16;105:19898–903. doi:10.1073/pnas.0805817105.
- Yasukawa T, Tabata Y, Kimura H, Ogura Y. Recent advances in intraocular drug delivery systems. Recent Pat Drug Deliv Formul. 2011 Jan;5:1–10.
- Figueras-Aloy J, Alvarez-Dominguez E, Morales-Ballus M, Salvia-Roiges MD, Moretones-Sunol G. [Early administration of erythropoietin in the extreme premature, a risk factor for retinopathy of prematurity?]. An Pediatr (Barc). 2010 Dec;73:327–33. doi:10.1016/j.anpedi.2010.09.001.
- Romagnoli C, Tesfagabir MG, Giannantonio C, Papacci P. Erythropoietin and retinopathy of prematurity. Early Hum Dev. 2011 Mar;87(Suppl 1):S39–42. doi:10.1016/j.earlhumdev.2011.01.027.
- Ayalasomayajula SP, Kompella UB. Subconjunctivally administered celecoxib-PLGA microparticles sustain retinal drug levels and alleviate diabetes-induced oxidative stress in a rat model. Eur J Pharmacol. 2005 Mar 28;511:191–98. doi:10.1016/j.ejphar.2005.02.019.
- Chen G, Shi X, Wang B, Xie R, Guo LW, Gong S, Kent KC. Unimolecular micelle-based hybrid system for perivascular drug delivery produces long-term efficacy for neointima attenuation in rats. Biomacromolecules. 2017 Jul 10;18:2205–13. doi:10.1021/acs.biomac.7b00617.
- Amrite AC, Ayalasomayajula SP, Cheruvu NP, Kompella UB. Single periocular injection of celecoxib-PLGA microparticles inhibits diabetes-induced elevations in retinal PGE2, VEGF, and vascular leakage. Invest Ophthalmol Vis Sci. 2006 Mar;47:1149–60. doi:10.1167/iovs.05-0531.
- Formiga FR, Pelacho B, Garbayo E, Abizanda G, Gavira JJ, Simon-Yarza T, Mazo M, Tamayo E, Jauquicoa C, Ortiz-de-Solorzano C, et al. Sustained release of VEGF through PLGA microparticles improves vasculogenesis and tissue remodeling in an acute myocardial ischemia-reperfusion model. J Control Release. 2010 Oct 1;147:30–37. doi:10.1016/j.jconrel.2010.07.097.
- Chang E, McClellan AJ, Farley WJ, Li DQ, Pflugfelder SC, de Paiva CS. Biodegradable PLGA-based drug delivery systems for modulating ocular surface disease under experimental murine dry eye. J Clin Exp Ophthalmol. 2011 Nov 1;2. doi:10.4172/2155-9570.1000191.
- Shive MS, Anderson JM. Biodegradation and biocompatibility of PLA and PLGA microspheres. Adv Drug Deliv Rev. 1997 Oct 13;28:5–24.
- Saito Y, Geisen P, Uppal A, Hartnett ME. Inhibition of NAD(P)H oxidase reduces apoptosis and avascular retina in an animal model of retinopathy of prematurity. Mol Vis. 2007;13:840–53.
- Connor KM, Krah NM, Dennison RJ, Aderman CM, Chen J, Guerin KI, Sapieha P, Stahl A, Willett KL, Smith LE. Quantification of oxygen-induced retinopathy in the mouse: a model of vessel loss, vessel regrowth and pathological angiogenesis. Nat Protoc. 2009;4:1565–73. doi:10.1038/nprot.2009.187.
- Mezu-Ndubuisi OJ. In vivo angiography quantifies oxygen-induced retinopathy vascular recovery. Optom Vis Sci. 2016;93:1268. doi:10.1097/OPX.0000000000000941.
- Pierce EA, Avery RL, Foley ED, Aiello LP, Smith LE. Vascular endothelial growth factor/vascular permeability factor expression in a mouse model of retinal neovascularization. Proc Natl Acad Sci U S A. 1995 Jan 31;92:905–09.
- Usui T, Ishida S, Yamashiro K, Kaji Y, Poulaki V, Moore J, Moore T, Amano S, Horikawa Y, Dartt D, et al. VEGF164(165) as the pathological isoform: differential leukocyte and endothelial responses through VEGFR1 and VEGFR2. Invest Ophthalmol Vis Sci. 2004 Feb;45:368–74.
- Saint-Geniez M, Maldonado AE, D’Amore PA. VEGF expression and receptor activation in the choroid during development and in the adult. Invest Ophthalmol Vis Sci. 2006 Jul;47:3135–42. doi:10.1167/iovs.05-1229.
- Woolard J, Wang WY, Bevan HS, Qiu Y, Morbidelli L, Pritchard-Jones RO, Cui TG, Sugiono M, Waine E, Perrin R, et al. VEGF165b, an inhibitory vascular endothelial growth factor splice variant: mechanism of action, in vivo effect on angiogenesis and endogenous protein expression. Cancer Res. 2004 Nov 1;64:7822–35. doi:10.1158/0008-5472.CAN-04-0934.
- Konopatskaya O, Churchill AJ, Harper SJ, Bates DO, Gardiner TA. VEGF165b, an endogenous C-terminal splice variant of VEGF, inhibits retinal neovascularization in mice. Mol Vis. 2006 May 26;12:626–32.
- Panyam J, Dali MM, Sahoo SK, Ma W, Chakravarthi SS, Amidon GL, Levy RJ, Labhasetwar V. Polymer degradation and in vitro release of a model protein from poly (D, L-lactide-co-glycolide) nano-and microparticles. J Control Release. 2003;92:173–87.
- Sandor M, Enscore D, Weston P, Mathiowitz E. Effect of protein molecular weight on release from micron-sized PLGA microspheres. J Control Release. 2001 Oct 19;76:297–311.
- Mezu-Ndubuisi OJ, Teng PY, Wanek J, Blair NP, Chau FY, Reddy NM, Raj JU, Reddy SP, Shahidi M. In vivo retinal vascular oxygen tension imaging and fluorescein angiography in the mouse model of oxygen-induced retinopathy. Invest Ophthalmol Vis Sci. 2013;54:6968–72. doi:10.1167/iovs.13-12126.
- Smith LE, Wesolowski E, McLellan A, Kostyk SK, D’Amato R, Sullivan R, D’Amore PA. Oxygen-induced retinopathy in the mouse. Invest Ophthalmol Vis Sci. 1994 Jan;35:101–11.
- Mezu-Ndubuisi OJ, Taylor LK, Schoephoerster JA. simultaneous fluorescein angiography and spectral domain optical coherence tomography correlate retinal thickness changes to vascular abnormalities in an in vivo mouse model of retinopathy of prematurity. J Ophthalmol. 2017;2017:1–9. doi:10.1155/2017/9620876.
- Stahl A, Connor KM, Sapieha P, Willett KL, Krah NM, Dennison RJ, Chen J, Guerin KI, Smith LEH. Computer-aided quantification of retinal neovascularization. Angiogenesis. 2009;12:297–301. doi:10.1007/s10456-009-9155-3.
- Zhang JS, Da Wang J, An Y, Xiong Y, Li J, Jonas J, Xu L, Zhang W, Wan XH. Cedilanid inhibits retinal neovascularization in a mouse model of oxygen-induced retinopathy. Mol Vis. 2017;23:346–55.
- Shen J, Samul R, Silva RL, Akiyama H, Liu H, Saishin Y, Hackett SF, Zinnen S, Kossen K, Fosnaugh K, et al. Suppression of ocular neovascularization with siRNA targeting VEGF receptor 1. Gene Ther. 2006 Feb;13:225–34. doi:10.1038/sj.gt.3302641.
- Gardiner TA, Gibson DS, de Gooyer TE, de la Cruz VF, McDonald DM, Stitt AW. Inhibition of tumor necrosis factor-alpha improves physiological angiogenesis and reduces pathological neovascularization in ischemic retinopathy. Am J Pathol. 2005 Feb;166:637–44.
- Medina RJ, O’Neill CL, Devine AB, Gardiner TA, Stitt AW. The pleiotropic effects of simvastatin on retinal microvascular endothelium has important implications for ischaemic retinopathies. PLoS One. 2008 Jul 9;3:e2584. doi:10.1371/journal.pone.0002584.
- Penn JS, Tolman BL, Bullard LE. Effect of a water-soluble vitamin E analog, trolox C, on retinal vascular development in an animal model of retinopathy of prematurity. Free Radic Biol Med. 1997;22:977–84.
- Zhang Q, Zhang J, Guan Y, Zhang S, Zhu C, Xu GT, Wang L. Suppression of retinal neovascularization by the iNOS inhibitor aminoguanidine in mice of oxygen-induced retinopathy. Graefes Arch Clin Exp Ophthalmol. 2009 Jul;247:919–27. doi:10.1007/s00417-009-1066-x.
- Benjamin LE, Hemo I, Keshet E. A plasticity window for blood vessel remodelling is defined by pericyte coverage of the preformed endothelial network and is regulated by PDGF-B and VEGF. Development. 1998 May;125:1591–98.
- Shelke NB, Kadam R, Tyagi P, Rao VR, Kompella UB. Intravitreal Poly(L-lactide) microparticles sustain retinal and choroidal delivery of TG-0054, a hydrophilic drug intended for neovascular diseases. Drug Deliv Transl Res. 2011 Feb;1:76–90. doi:10.1007/s13346-010-0009-8.
- Gaddipati S, Lu Q, Kasetti RB, Miller MC, Lu Q, Trent JO, Kaplan HJ, Li Q. IKK2 inhibition using TPCA-1-loaded PLGA microparticles attenuates laser-induced choroidal neovascularization and macrophage recruitment. PLoS One. 2015;10:e0121185. doi:10.1371/journal.pone.0121185.
- Sinha VR, Trehan A. Biodegradable microspheres for protein delivery. J Control Release. 2003;90:261–80.
- Torchilin VP, Lukyanov AN. Peptide and protein drug delivery to and into tumors: challenges and solutions. Drug Discov Today. 2003;8:259–66.
- White LJ, Kirby GTS, Cox HC, Qodratnama R, Qutachi O, Rose FRAJ, Shakesheff KM. Accelerating protein release from microparticles for regenerative medicine applications. Mater Sci Eng C. 2013;33:2578–83. doi:10.1016/j.msec.2013.02.020.
- Andrieu-Soler C, Aubert-Pouessel A, Doat M, Picaud S, Halhal M, Simonutti M, Venier-Julienne MC, Benoit JP, Behar-Cohen F. Intravitreous injection of PLGA microspheres encapsulating GDNF promotes the survival of photoreceptors in the rd1/rd1 mouse. Mol Vis. 2005 Nov 17;11:1002–11.
- Bible E, Qutachi O, Chau DY, Alexander MR, Shakesheff KM, Modo M. Neo-vascularization of the stroke cavity by implantation of human neural stem cells on VEGF-releasing PLGA microparticles. Biomaterials. 2012 Oct;33:7435–46. doi:10.1016/j.biomaterials.2012.06.085.
- Visconti RP, Richardson CD, Sato TN. Orchestration of angiogenesis and arteriovenous contribution by angiopoietins and vascular endothelial growth factor (VEGF). Proc Natl Acad Sci U S A. 2002 Jun 11;99:8219–24. doi:10.1073/pnas.122109599.
- Hao X, Silva EA, Mansson-Broberg A, Grinnemo KH, Siddiqui AJ, Dellgren G, Wardell E, Brodin LA, Mooney DJ, Sylven C. Angiogenic effects of sequential release of VEGF-A165 and PDGF-BB with alginate hydrogels after myocardial infarction. Cardiovasc Res. 2007 Jul 1;75:178–85. doi:10.1016/j.cardiores.2007.03.028.
- Yang JP, Liu HJ, Wang ZL, Cheng SM, Cheng X, Xu GL, Liu XF. The dose-effectiveness of intranasal VEGF in treatment of experimental stroke. Neurosci Lett. 2009 Sep 25;461:212–16. doi:10.1016/j.neulet.2009.06.060.
- Beckert S, Farrahi F, Aslam RS, Scheuenstuhl H, Konigsrainer A, Hussain MZ, Hunt TK. Lactate stimulates endothelial cell migration. Wound Repair Regen. 2006 May–Jun;14:321–24. doi:10.1111/j.1743-6109.2006.00127.x.
- Porporato PE, Payen VL, de Saedeleer CJ, Preat V, Thissen JP, Feron O, Sonveaux P. Lactate stimulates angiogenesis and accelerates the healing of superficial and ischemic wounds in mice. Angiogenesis. 2012 Dec;15:581–92. doi:10.1007/s10456-012-9282-0.
- De Saedeleer CJ, Copetti T, Porporato PE, Verrax J, Feron O, Sonveaux P. Lactate activates HIF-1 in oxidative but not in Warburg-phenotype human tumor cells. PLoS One. 2012;7:e46571. doi:10.1371/journal.pone.0046571.
- Romijn HJ, Hofman MA, Gramsbergen A. At what age is the developing cerebral cortex of the rat comparable to that of the full-term newborn human baby? Early Hum Dev. 1991 Jul;26:61–67.