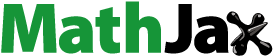
Abstract
Purpose
Intravitreal injections of anti-vascular endothelial growth factors (anti-VEGF) are the current standard of care for patients with choroidal neovascularization (CNV) secondary to age-related macular degeneration (AMD). There is a growing subset of patients that does not respond to anti-VEGF monotherapy treatment. Some patients, however, do respond to combination therapy of corticosteroids and anti-VEGF. This treatment requires monthly/bimonthly injections of anti-VEGF and semi-annual injections of corticosteroid. A drug delivery system (DDS) that simultaneously releases multiple drugs could benefit these patients by reducing the number of injections. The purpose of this study was to characterize the simultaneous release of aflibercept and dexamethasone from a biodegradable microparticle- and nanoparticle-hydrogel DDS.
Methods
Dexamethasone-loaded nanoparticles and aflibercept-loaded microparticles were created using modified single- and double-emulsion techniques, respectively. Then, microparticles and nanoparticles were embedded into a thermoresponsive, biodegradable poly(ethylene glycol)-co-(L-lactic acid) diacrylate (PEG-PLLA-DA)-N-isopropylacrylamide (NIPAAm) hydrogel DDS. Drug release studies and characterization of DDS were conducted with varying doses of microparticles and nanoparticles.
Results
The combination aflibercept-loaded microparticle- and dexamethasone-loaded nanoparticle- hydrogel (Combo-DDS) achieved a total release time of 224 days. Small decreases were seen in swelling ratio and equilibrium water content for Combo-DDS compared to monotherapy aflibercept-loaded microparticle-hydrogel DDS (AFL-DDS) and monotherapy dexamethasone-loaded nanoparticle-hydrogel DDS (DEX-DDS). Bioactivity of aflibercept was maintained in Combo-DDS compared to AFL-DDS.
Conclusions
The Combo-DDS was able to extend and control the release of both aflibercept and dexamethasone simultaneously from a single DDS. This may eliminate the need for separate dosing regiments of anti-VEGF and corticosteroids for wet AMD patients.
Introduction
Age-related macular degeneration (AMD) is the leading cause of vision loss in the elderly population.Citation1,Citation2 Wet AMD, which accounts for 10% of AMD cases, is characterized by abnormal blood vessel growth in the macula.Citation3 These immature blood vessels cause leakage, hemorrhage, and retinal damage leading to rapid and severe vision loss.Citation4,Citation5 Clinically, the current standard of care for patients with choroidal neovascularization (CNV) secondary to wet AMD is monthly or bimonthly intravitreal (IVT) injections of anti-vascular endothelial growth factor (VEGF).Citation6,Citation7 Anti-VEGFs used to treat wet AMD include bevacizumab (Avanstin®, off-label, Genentech, San Francisco, CA, USA), ranibizumab (Lucentis®, Genentech), and aflibercept (EYLEA®, Regeneron Pharmaceuticals, Tarrytown, NY, USA).
While this treatment has proven to be safe and effective, about 45% of wet AMD patients do not fully respond to monotherapy anti-VEGF treatment.Citation8 Some studies, however, have shown that these patients respond to combination therapy of corticosteroids and anti-VEGF.Citation9–12 Like many retinal diseases, it has been demonstrated that the progression of CNV involves both angiogenesis and inflammatory pathways.Citation13–19 The addition of corticosteroids addresses the inflammatory pathway of CNV that is not targeted by anti-VEGF. Dexamethasone, a steroid that helps reduce inflammation, has shown potential in maintaining visual acuity and reducing central retinal thickness when delivered in combination with monthly or bi-monthly IVT injections of anti-VEGF.Citation12,Citation17 Dexamethasone must be delivered, however, as an implant due to its low solubility and short vitreous half-life of 3.48 hours.Citation20 Thus, this combination treatment requires separate dosing regiments for each drug: monthly or bimonthly bolus IVT injections of anti-VEGF and semi-annual IVT injections of a dexamethasone implant such as Ozurdex® (Allergan Inc., Irvine, CA, USA).
A major limitation of anti-VEGF treatment is the need for monthly or bimonthly bolus IVT injections due to rapid clearance and short vitreous half-lives.Citation21 The known risks of complications associated with repeated IVT injections include retinal detachment, endophthalmitis, IVT hemorrhage, and cataract.Citation4,Citation5 Additionally, the frequency of treatment places a significant burden on patients and healthcare systems. Lastly, bolus injections have suboptimal pharmacokinetics by reaching peak drug concentration immediately after administration that is rapidly cleared and is then followed by subtherapeutic concentrations between doses that allow for further disease progression.Citation22
Many drug delivery systems (DDS) have been developed to extend the release of anti-VEGF to address these limitations.Citation23 Some examples of successful DDSs include polymer-based micro- and nano-particles, hydrogels, cell-based therapeutics, and composite systems.Citation24 Recently, Susvimo™ (Genentech), a port delivery system that releases ranibizumab for six months, received FDA approval for the treatment of wet AMD after its successful results in the Archway phase 3 trial.Citation25,Citation26 The port system must be surgically implanted, but then only requires refills every six months.Citation26 Additionally, our laboratory has developed a composite microparticle-hydrogel DDS to continually release anti-VEGF for six months.Citation27–32 In this system, aflibercept-loaded poly(ethylene glycol)-co-(L-lactic acid) (PLGA) microparticles are embedded into a thermoresponsive, biodegradable poly(ethylene glycol)-co-(L-lactic acid) diacrylate (PEG-PLLA-DA)-N-isopropylacrylamide (NIPAAm) hydrogel. It has shown treatment efficacy and safety in a laser-induced CNV model in rodents and non-human primates.Citation31,Citation32
By encapsulating dexamethasone into PLGA particles and then embedding into this composite system, a single DDS would administer both aflibercept and dexamethasone, therefore eliminating the need for separate dosing regiments. By simultaneously releasing aflibercept and dexamethasone in a controlled manner for six months, a more advantageous pharmacokinetic profile can be achieved. It would also result in the reduction of the total number of IVT injections, reducing the risks of complications associated with repeated IVT injections and the socioeconomic burden on patients and healthcare systems.
The purpose of this study was to characterize the extended and controlled dual release of dexamethasone-loaded PLGA nanoparticles and aflibercept-loaded PLGA microparticles from a single thermoresponsive, biodegradable poly(ethylene glycol)-co-(L-lactic acid) diacrylate-N-isopropylacrylamide hydrogel DDS in vitro. Optimal release kinetics for dexamethasone were determined by varying the loading dose of dexamethasone-loaded nanoparticles in the DDS. The effects of nanoparticle loading dose on drug release kinetics, swelling ratio, and equilibrium water content were investigated. Additionally, in vitro bioactivity of aflibercept was evaluated after microparticle fabrication and after release from the DDS.
Methods
Fabrication of DDS
Dexamethasone (Sigma-Aldrich, St. Louis, MO) was encapsulated into PLGA (75:25, MW 4–15 kDa) nanoparticles by a modified single-emulsion, solvent evaporation technique.Citation23,Citation33 Dexamethasone and PLGA were dissolved in dichloromethane (DCM) to create the oil phase, and polyvinyl alcohol (PVA) was used as the water phase. The oil-in-water emulsion (o/w) was created by sonication at 100 watts for 3.5 minutes. After the solvent was allowed to evaporate, dexamethasone loaded-nanoparticles (DEX-np) were collected by centrifugation, washed with deionized (DI) water at least three times, lyophilized, and stored at 4 °C.
Similarly, aflibercept (Eylea®, Regeneron, Tarrytown, NY) was encapsulated into PLGA (75:25) microspheres by a modified double-emulsion, solvent evaporation technique.Citation23,Citation29,Citation30 The first emulsion (w1/o) was created by vortex, then immediately added to the polyvinyl alcohol phase (w2) to create a double emulsion (w1/0/w2) by the vortex. Excipients were added to the inner aqueous phase (w1) for protein stabilization and to the oil phase (o) to act as a buffer.Citation29 After solvent evaporation, aflibercept loaded-microparticles (AFL-mp) were collected by centrifugation, washed with DI water at least three times, lyophilized, and stored at 4 °C.
Thermoresponsive, biodegradable PEG-PLLA-DA/NIPAAm hydrogels were synthesized by free radical polymerization, described elsewhere.Citation27,Citation34 NIPAAm (350 mM), N-tert-butylacrylamide (50 mM), ammonium persulfate (13 mM), and PEG-PLLA-DA (2 mM) were dissolved into 1xPBS (pH 7.4) to prepare the hydrogel precursor solution.Citation30 Varying loading doses of microparticles (0 mg/ml and 20 mg/ml) and nanoparticles (0 mg/ml, 20 mg/ml, 40 mg/ml, 60 mg/ml, and 80 mg/ml) were suspended into the solution to create the composite DDS. N,N,N’,N’-tetramethylethylenediamine (168 mM, pH 7.4) was added to the hydrogel precursor solutions to initiate polymerization. Hydrogels were placed on ice for 30 minutes while the reaction proceeded to create nanoparticle- and microparticle-hydrogel DDS. DDS was then collected, washed with DI water five times, and stored at 4 °C.
Aflibercept radiolabeling
Aflibercept was radiolabeled with iodine-125 using iodination beads (Pierce, Rockford, IL, USA) for all characterization and release kinetic studies. To remove any unbound, free iodine, radiolabeled aflibercept was dialyzed against DI water using a dialysis cassette for 48 hours. Then radiolabeled aflibercept was collected, lyophilized, and dissolved in PBS to create a 40 mg/ml stock solution. Radioactivity was measured using a gamma counter (Cobra-II, Auto-Gamma, Packard Instrument Co., Meriden, CT).
Encapsulation efficiencies and characterization
Encapsulation efficiency (E.E.) for microparticles and nanoparticles was defined as the percent of drug within the particles relative to the theoretical loading amount of the drug. Similarly, E.E. of particles into the hydrogel was defined as the percent of particles in the hydrogel relative to the theoretical loading amount of particles. The size distribution of dexamethasone-loaded nanoparticles was determined using Nanoparticle Tracking Analysis (NanoTracking v 3.0, NanoSight LM10, Malvern, UK). For dexamethasone, E.E. into nanoparticles was determined by using reverse-phase HPLC as previously reported.Citation35 Nanoparticle encapsulation into the hydrogel could not be directly measured due to experimental limitations.
For aflibercept-loaded microparticles, particle size was determined by capturing images with a light microscope and using ImageJ Software (National Institutes of Health, Bethesda, MD). Radioactivity before and after fabrication of microparticles and hydrogel DDS was used to determine the E.E.’s for aflibercept.
In vitro drug release studies
In vitro release profiles of both dexamethasone and aflibercept from composite DDS were conducted using a separation method described elsewhere.Citation36 Three preparations of composite DDS were made for release studies: (1) monotherapy aflibercept-loaded microparticle-hydrogel DDS (AFL-DDS), (2) monotherapy dexamethasone-loaded nanoparticle-hydrogel DDS (DEX-DDS) and (3) combination aflibercept-loaded microparticle- and dexamethasone-loaded nanoparticle- hydrogel DDS (Combo-DDS). Each DDS was prepared in 1 ml volumes and incubated at 37 °C in 1.5 ml of PBS (1x, pH 7.4). At predetermined time points, 1 ml of supernatant was removed for analysis and replaced with 1 ml of fresh PBS.
Dexamethasone release studies were conducted using a NanoDrop™ OneC (Thermo-Fischer Scientific Inc., MA, USA), a microvolume UV-spectrophotometer. To determine optimal release kinetics of dexamethasone, release studies for DEX-DDS consisted of 20, 40, 60 or 80 mg/ml dexamethasone-loaded nanoparticles (DEX-np). Similarly, release studies for Combo-DDS consisted of 20 mg/ml DEX-np with 20 mg/ml of aflibercept-loaded microparticles (AFL-mp).
For aflibercept release profiles, radiolabeled aflibercept was used to conduct release studies using a gamma counter. The AFL-DDS previously developed by our laboratory showed treatment efficacy with a microparticle loading dose of 20 mg/ml.Citation31 Thus, AFL-DDS and Combo-DDS consisted of 20 mg/ml AFL-mp, and 20 mg/ml AFL-mp and 20 mg/ml of DEX-np, respectively.
Temperature dependent swelling ratios and equilibrium water content
Due to the thermoresponsive nature of the hydrogel, swelling ratios and equilibrium water content (EWC) at varying temperatures were measured. After polymerization, hydrogels were incubated at 22 °C overnight to allow for equilibration. Wet weight (Wwet) of hydrogels was measured from 22 °C to 46 °C after 30 minutes of incubation at each temperature. Hydrogels were then lyophilized to determine the dry weight (Wdry). The swelling ratio (Q) was defined using the following equation:
(1)
(1)
Similarly, the equilibrium water content (EWC) was defined as:
(2)
(2)
EWC for each DDS was determined by averaging the values at each temperature.
Bioactivity
First, the bioactivity of aflibercept after microparticle formulation was evaluated. The primary emulsification (w1/o) was simulated to determine the effects of the organic phase (DCM) on aflibercept stability. The inner water phase (w1) was created by dissolving 100 µl of aflibercept stock solution (40 mg/ml) in PBS with 12% BSA (w/v%). Then it was incorporated into the organic phase at a 1:5 v/v water-to-organic phase ratio. The emulsification was created by stirring at 3200 rpm for 90 s to evaluate the water/organic solvent interface effect. In order to extract aflibercept from the organic phase, 4 ml of PBS was added, and then the mixture was stirred for 2 min. Then the mixture was centrifuged at 5000 rpm for 10 min to accelerate phase separation (total o/w ratio was 1:8.2). The aqueous phase was collected and used to evaluate the recovered amount and stability of aflibercept by enzyme-linked immunosorbent assay (ELISA). As a control, PBS was used in place of the organic phase and the same procedure was conducted.
Next, the bioactivity of aflibercept released from DDS was determined. Aflibercept-loaded DDS showed significant inhibition of VEGF-induced human umbilical vascular endothelial cell (HUEVC) proliferation compared to blank DDS at all timepoints.Citation28 This indicates that the bioactivity of aflibercept remains throughout the entire release time. To determine the bioactivity of aflibercept from Combo-DDS, HUVECs were cultured, trypsinized, and seeded into 96-well plates at 5000 cells per well in a growth medium (Lonza Inc., Allendale, NJ, USA). After the cells had grown to ∼80% confluence (∼48 hours), cell growth was arrested by washing the plates twice with PBS (1x, pH 7.4) and then adding 50 µL of basal growth media (Lonza Inc., Allendale, NJ, USA) to each well. To achieve VEGF-induced proliferation, 25 µL of 10 ng/mL exogenous VEGF (Lonza) was added to each well. Then, 25 µL of a release sample of anti-VEGF (aflibercept) was added.
A solution containing a tetrazolium compound (3-(4,5-dimethylthiazol-2-yl)-5-(3-carboxymethoxyphenyl)-2-(4-sulfophenyl)-2H-tetrazolium, inner salt; MTS) and an electron coupling reagent (phenazine ethosulfate) (MTS assay, CellTiter 96® Aqueous One Solution Reagent; Promega, Madison, WI, USA) was used according to the manufacturer’s protocol to determine cell proliferation and cytotoxicity after exposure to the release samples for two days. According to the MTS assay protocol, cell proliferation is proportional to the optical density (OD) of a sample when read using a spectrophotometer at 490 nm 1–4 hours after adding the MTS reagent. Cell proliferation was normalized relative to wells receiving only exogenous 10 ng/mL VEGF (negative control). Positive controls consisted of 25 µL of a clinical dose of the drug (40 mg/mL aflibercept) added to wells containing 25 µL 10 ng/mL VEGF.
Statistical analysis
All values are reported as mean ± standard error and in all graphs, error bars represent standard error. All statistical analysis between experimental groups was performed using Student’s t-test and any p value less than 0.05 was considered to be significant.
Results
Characterization of particles
AFL-mp and DEX-np were successfully fabricated. AFL-mp had an average diameter of 6.9 ± 0.4 µm and encapsulation efficiency of the drug into microparticle of 66.6 ± 1.4% (n = 3), which agrees with previously reported values.Citation27,Citation30 Dexamethasone nanoparticles had an average diameter of 138.9 ± 6.2 nm and encapsulation efficiency of a drug into nanoparticles of 91.6 ± 4.2% (n = 10).
In vitro release studies
Release profiles of dexamethasone
Cumulative releases of dexamethasone from DEX-DDS can be seen in . All loading doses of DEX-np were successfully embedded into DDS and maintained drug release for at least 200 days. Loading doses of 20, 40, 60 and 80 mg/ml had similar release rates of 16.0, 21.1, 19.4, and 19.6 ug/day, respectively, within the first 100 days (). While the 20 mg/ml continued at a similar release rate of 18.4 ug/day, the higher loading doses of DEX-np had a drastic increase in release rate after day 100. The release rates after 100 days increased to 32.2, 27.0, and 64.3 ug/day for 40, 60 and 80 mg/ml, respectively.
Figure 1. In vitro release of dexamethasone from DEX-DDS with 20, 40, 60, and 80 mg/ml loading dose of dexamethasone-loaded nanoparticles. Error bars represent standard error (n = 3).
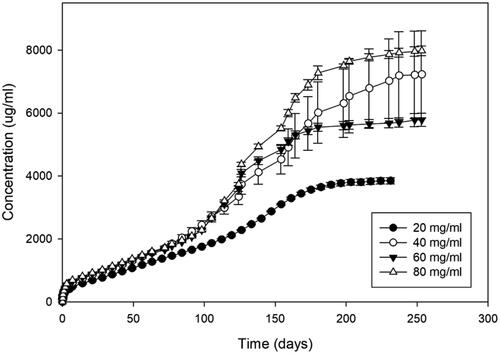
Table 1. Release characteristics of dexamethasone from DEX-DDS.
The 20 mg/ml loading dose had the most consistency and control of release and therefore was used for DEX-np dose in the Combo-DDS for all other experiments. As seen in , the addition of AFL-mp into the DDS did not significantly change the release kinetic or characterization of dexamethasone release. DEX-DDS had an initial burst, a release rate after 7 days, release time and total drug release of 244.5 ± 17.0 ug, 17.6 ug/day (R2 = 0.98), 224 days, and 3850.8 ± 99.8 ug, respectively. Similarly, Combo-DDS had an initial burst, release rate after 7 days, release time and total drug release of 217.8 ± 2.5 ug, 22.9 ug/day (R2 = 0.97), 252 days, and 5764.8 ± 3.2 ug, respectively (). No significant differences were seen in an initial burst, release rate, or total drug released for DEX-DDS and Combo-DDS. However, Combo-DDS was able to further reduce the initial burst and increase the total release time and amount of dexamethasone released.
Figure 2. Cumulative releases of dexamethasone from DEX-DDS and Combo-DDS. Error bars represent standard error (n = 3).
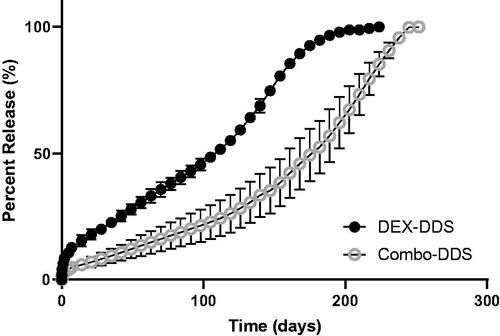
Table 2. Dexamethasone release characteristics from DDS.
Release profiles of aflibercept
shows the cumulative releases of aflibercept microparticles (20 mg/ml) from both AFL-DDS and Combo-DDS. The release of aflibercept from AFL-DDS had similar characteristics as previously reported.Citation30 shows the release characteristics of aflibercept from AFL-DDS and Combo-DDS. AFL-DDS achieved a total aflibercept drug load of 277.9 ± 8.4 ug and an initial burst of 66.7 ± 11.2 ug. Similarly, Combo-DDS achieved a total aflibercept drug load of 267.4 ± 2.2 ug and an initial burst of 54.2 ± 7.7 ug. There were no significant differences between AFL-DSS and Combo-DDS for initial burst or total aflibercept drug load (p > 0.2). After 7 days, AFL-DDS and Combo-DDS achieved release rates of 0.37 and 0.58 ug/day, respectively. Combo-DDS was able to achieve a longer release time of 224 days compared to the AFL-DDS release time of 203 days. Similarly, Combo-DDS achieved a higher final percent of aflibercept released (89.7 ± 5.1) compared to AFL-DDS (69.4 ± 6.8).
Figure 3. Cumulative releases of aflibercept from AFL-DDS and Combo-DDS. Error bars represent standard error (n = 3).
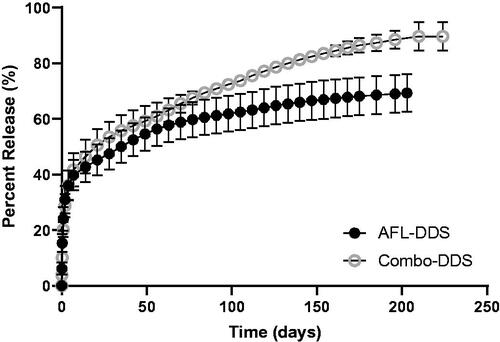
Table 3. Aflibercept release characteristics from DDS.
Temperature-Dependent swelling ratios and equilibrium water content
shows the swelling ratios and equilibrium water content (EWC) for DEX-DDS, AFL-DDS, and Combo-DDS. At room temperature (21.8 °C), swelling ratios for DEX-DDS, AFL-DDS, and Combo- DDS was 18.3 ± 0.3, 16.5 ± 0.5, and 13.3 ± 0.1, respectively. Similar decreasing trends were seen for all DDSs from 22 to 46 °C. Above physiological temperature (42 °C), swelling ratios for DEX-DDS, AFL-DDS, and Combo-DDS were 7.1 ± 0.2, 6.9 ± 0.3, and 5.7 ± 0.2, respectively. Statistically significant differences were found between Combo-DDS and AFL-DDS (p < 0.05), in addition to Combo-DDS and DEX-DDS (p < 0.05), at each temperature. EWC for DEX-DDS, AFL-DDS, and Combo-DDS hydrogels were 93.2 ± 2.6, 93.6 ± 2.6, and 91.7 ± 2.9, see . A statistically significant difference was found between the EWC of Combo-DDS and AFL-DDS (p = 0.035). No other significant differences were seen in EWC.
Figure 4. Swelling ratios (a) and equilibrium water content (b) for dexamethasone, aflibercept and combination DDSs at varying temperatures. “DEX-DDS” had 20 mg/ml of dexamethasone nanoparticles, “AFL-DDS” had 20 mg/ml of aflibercept microparticles, and “Combo-DDS” had 20 mg/ml of dexamethasone nanoparticles and 20 mg/ml of aflibercept microparticles. Error bars represent standard error (n = 3). A statistically significant difference (p < 0.05) between DEX-DDS and Combo-DDS is indicated by * and between AFL-DDS and Combo-DDS is indicated by #.
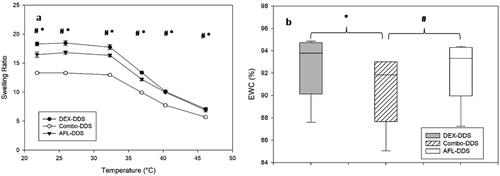
Bioactivity of aflibercept
In order to evaluate aflibercept bioactivity after exposure to organic solvent (DCM), the primary emulsification was simulated, and ELISA was conducted with the recovered aflibercept. When compared to the control (PBS), aflibercept maintained 91.0 ± 3.2% of its bioactivity (n = 3). No significant differences were seen with exposure to DCM compared to exposure to PBS (p = 0.38). Additionally, the bioactivity of aflibercept released from DDS was evaluated. Proportional increases in HUVEC proliferation were observed with varying amounts of VEGF (positive controls) compared to a negative control (BGM) (). The addition of a clinical dose of aflibercept (40 mg/ml) showed a significant reduction of VEGF-induced proliferation compared to positive controls (p < 0.05). The addition of aflibercept did not show any significant difference compared to a negative control (p > 0.62). Significant inhibition of VEGF-induced proliferation was observed at each time point throughout the release period of AFL-DDS and Combo-DDS (). This is shown by the reduction in normalized OD for all drug-treated HUVECs compared to the controls. No significant differences were seen between AFL-DDS and Combo-DDS.
Discussion
In this study, dexamethasone-loaded nanoparticles and aflibercept-loaded microparticles were successfully embedded and released from a biodegradable, thermoresponsive hydrogel drug delivery system for ∼200 days. DEX-np were created using a modified single-emulsion technique, resulting in PLGA nanoparticles averaging 138.9 ± 6.2 nm in diameter with a drug encapsulation efficiency of 91.6 ± 4.2%. Similarly, AFL-mp were created using a modified double-emulsion technique, which resulted in PLGA microparticles averaging 6.9 ± 0.4 µm in diameter and a drug encapsulation efficiency of 66.6 ± 1.4%. Long-term release of dexamethasone was achieved by the degradable nanoparticle-hydrogel DDS. Additionally, combining DEX-np and AFL-mp did not significantly change drug release kinetics compared to their single releases. The Combo-DDS in this study has the potential to replace the standard treatment of monthly/bimonthly IVT injections of anti-VEGF and semi-annual IVT injections of dexamethasone implant for non-responsive patients.
While some optimization of dexamethasone encapsulation into PLGA particles has been done, consistency in DEX-np fabrication remains a challenge due to the poor water solubility (0.16 mg/ml)Citation37 and crystallization of drug.Citation33 Variability in drug encapsulation leads to differences in the final drug-to-polymer ratio, which may explain why there is not a direct relationship between an increase in nanoparticle load and an increase in total drug release. Meaning, different loading doses of DEX-np could result in similar amounts of total drug released since the loading dose is based on the weight of particles not the weight of the drug. Further optimization of dexamethasone encapsulation into PLGA nanoparticles could achieve more consistent drug release.
All loading doses of DEX-np (20, 40, 60 and 80 mg/ml) were investigated to determine release kinetics and characterization from 1 ml of DDS. Similar release rates were observed within the first 100 days of all releases. After 100 days, 20 mg/ml loading dose maintained its release rate, while the higher loading doses had more drastic increases in release rate (). We speculate that as the hydrogel degrades and loses its integrity, release rates increase since the hydrogel acts as less of a barrier, which would be compounded at higher doses. When scaled to a clinical injection volume of 50 µl, all loading doses of DEX-np would maintain drug levels that have been shown to suppress inflammation intravitreally (0.15–4.00 µg/ml),Citation38–43 and therefore would all be viable options. There are some associated risks, however, with long-term dexamethasone use. In the GENEVA study, there was a significant occurrence of cataract progression in retinal vein occlusion (RVO) patients after only 12 months of receiving a DEX implant.Citation44 Similarly, increases in intraocular pressure (IOP) were found in RVO patients after two injections of DEX.Citation44 To minimize any risk of continuous DEX treatment, the lowest loading dose of DEX-np (20 mg/ml) was used for Combo-DDS. When embedded in combination, no significant changes in characterization or the release kinetics of dexamethasone or aflibercept were observed compared to their individual single releases ( and ).
The thermal transition behavior of PEG-PLLA-DA/NIPAAm hydrogels with micro- and nanoparticles was evaluated from 22 to 46 °C. DEX-DDS, AFL-DDS and Combo-DDS had an inverse relationship between temperature and swelling ratio (), which is similar to previously reported trends.Citation29 Since hydrogel mesh size is proportional to the swelling ratio, these results indicate that the hydrogel mesh size would decrease at physiological temperature and allow for a more controlled drug release in vivo. As for EWC, all DDSs had relatively high values (), which is advantageous since hydrogels with high water content are generally more biocompatible in medical applications.Citation45 Differences in swelling ratio and EWC were seen when comparing Combo-DDS to both DEX-DDS and AFL-DDS (p < 0.05), which may be due to the increase in total dry weight compared to AFL-DDS and DEX-DDS. The Combo-DDS has double the total weight of microparticles and nanoparticles than the monotherapy DDSs, which in turn would yield a higher dry weight. This would account for the decreases in swelling ratio and EWC seen in the Combo-DDS since both are directly calculated using dry weight.
For protein encapsulation using a double emulsion technique, the organic solvent has the greatest influence on bioactivity due to denaturation and aggregation of protein at the interface.Citation46–48 Therefore, simulation of the primary emulsion (w1/o) was used to evaluate changes in bioactivity due to the microparticle formulation process. No change in aflibercept bioactivity was seen with the presence of BSA (12% w/v). Previous reports have shown that albumin is the most protective additive in the primary emulsion.Citation48–50 It is thought that albumin occupies the solvent/water interface, which prevents therapeutic proteins from contacting the interface.Citation49–52
Dexamethasone loaded-nanoparticles can be incorporated into the aflibercept-loaded microparticle-hydrogel DDS without significantly altering the bioactivity of released aflibercept. No significant differences were seen in the bioactivity of aflibercept from AFL-DDS or Combo-DDS (). Previous studies have shown that dexamethasone does not inhibit VEGF-induced proliferation in vitro.Citation9,Citation53 and therefore, no differences in aflibercept bioactivity would be expected between AFL-DDS and Combo-DDS. The similarities in the bioactivity of AFL indicate that there are no antagonistic drug-drug interactions from Combo-DDS, thus allowing for combination therapy DDS rather than two monotherapies.
By modifying the particle-hydrogel DDS, this system can effectively control and extend drug release for a range of drugs, from hydrophobic steroids to hydrophilic proteins. This study shows that simultaneous release of drugs can be achieved without significant changes to release kinetics or characterization. The nanoparticle- and microparticle-hydrogel DDS has proven to be an effective method for simultaneously releasing dexamethasone and aflibercept for six months. Not only would this DDS eliminate the need for separate dosing regiments of anti-VEGF and corticosteroids for wet AMD patients, but it would also reduce the annual number of IVT injections, lower the socioeconomic burden on patients and healthcare systems, and allow for more favorable release kinetics.
Disclosure statement
Jennifer J. Kang-Mieler has US Patent, and the rest of the authors declare they have no conflict of interest.
Data availability statement
The data that support the findings of this study are available from the corresponding author, JJKM, upon reasonable request.
Additional information
Funding
References
- Bourne RR, Jonas JB, Flaxman SR, Keeffe J, Leasher J, Naidoo K, Parodi MB, Pesudovs K, Price H, White RA, et al. Prevalence and causes of vision loss in high-income countries and in Eastern and Central Europe: 1990–2010. Br J Ophthalmol. 2014;98(5):629–638. doi:10.1136/bjophthalmol-2013-304033.
- Wong WL, Su X, Li X, Cheung CM, Klein R, Cheng CY, Wong TY. Global prevalence of age-related macular degeneration and disease burden projection for 2020 and 2040: a systematic review and meta-analysis. Lancet Glob. Health. 2014;2(2):e106–e116. doi:10.1016/S2214-109X(13)70145-1.
- Foundation AMD 2017. Dry vs wet age-related macular degeneration. [accessed 2021 November 8]. http://www.macular.org/dry-vs-wet-macular-degeneration.
- Ghasemi Falavarjani K, Nguyen QD. Adverse events and complications associated with intravitreal injection of anti-VEGF agents: a review of literature. Eye. 2013;27(7):787–794. doi:10.1038/eye.2013.107.
- Peyman GA, Lad EM, Moshfeghi DM. Intravitreal injection of therapeutic agents. Retina. 2009;29(7):875–912. doi:10.1097/IAE.0b013e3181a94f01.
- Park YG, Rhu HW, Kang S, Roh YJ. New approach of anti-VEGF agents for age-related macular degeneration. J Ophthalmol. 2012;2012:637316. doi:10.1155/2012/637316.
- Kovach JL, Schwartz SG, Flynn HW, Scott IU. Anti-VEGF treatment strategies for wet AMD. J Ophthalmol. 2012;2012:786870. doi:10.1155/2012/786870.
- Lux A, Llacer H, Heussen FM, Joussen AM. Non-responders to bevacizumab (Avastin) therapy of choroidal neovascular lesions. Br J Ophthalmol. 2007;91(10):1318–1322. doi:10.1136/bjo.2006.113902.
- Wang Q, Jiang J, Chen W, Jiang H, Zhang Z, Sun X. Targeted delivery of low-dose dexamethasone using PCL-PEG micelles for effective treatment of rheumatoid arthritis. J Control Release. 2016;230:64–72. doi:10.1016/j.jconrel.2016.03.035.
- Al-Khersan H, Hussain RM, Ciulla TA, Dugel PU. Innovative therapies for neovascular age-related macular degeneration. Expert Opin Pharmacother. 2019;20(15):1879–1891. doi:10.1080/14656566.2019.1636031.
- Yang S, Zhao J, Sun X. Resistance to anti-VEGF therapy in neovascular age-related macular degeneration: a comprehensive review. Drug Design Dev Ther. 2016;10:1857.
- Kaya C, Zandi S, Pfister IB, Gerhardt C, Garweg JG. Adding a corticosteroid or switching to another anti-VEGF in insufficiently responsive wet age-related macular degeneration. Clin Ophthalmol. 2019;13:2403–2409. doi:10.2147/OPTH.S224456.
- Donoso LA, Kim D, Frost A, Callahan A, Hageman G. The role of inflammation in the pathogenesis of age-related macular degeneration. Surv Ophthalmol. 2006;51(2):137–152. doi:10.1016/j.survophthal.2005.12.001.
- Campochiaro PA, Soloway P, Ryan SJ, Miller JW. The pathogenesis of choroidal neovascularization in patients with age-related macular degeneration. Mol Vis. 1999;5(11):34–38.
- London NJ, Chiang A, Haller JA. The dexamethasone drug delivery system: indications and evidence. Adv Ther. 2011;28(5):351–366. doi:10.1007/s12325-011-0019-z.
- Chaudhary V, Barbosa J, Lam W-C, Mak M, Mavrikakis E, Mohaghegh P SM. Ozurdex in age-related macular degeneration as adjunct to ranibizumab (The OARA Study). Can J Ophthalmol. 2016;51(4):302–305. doi:10.1016/j.jcjo.2016.04.020.
- Todorich B, Thanos A, Yonekawa Y, Mane G, Hasbrook M, Thomas BJ, Woodward MA, Williams GA, Capone A, Wolfe JD, et al. Simultaneous dexamethasone intravitreal implant and anti-VEGF therapy for neovascular age-related macular degeneration resistant to anti-VEGF monotherapy. J Vitreoretin Dis. 2017;1(1):65–74. doi:10.1177/2474126416683299.
- Tuo J, Grob S, Zhang K, Chan C-C. Chan CC Genetics of immunological and inflammatory components in age-related macular degeneration. Ocul Immunol Inflamm. 2012;20(1):27–36. doi:10.3109/09273948.2011.628432.
- Nozaki M, Raisler BJ, Sakurai E, Sarma JV, Barnum SR, Lambris JD, Chen Y, Zhang K, Ambati BK, Baffi JZ, et al. Drusen complement components C3a and C5a promote choroidal neovascularization. Proc Natl Acad Sci USA. 2006;103(7):2328–2333. doi:10.1073/pnas.0408835103.
- Kwak HW, D'Amico DJ. Evaluation of the retinal toxicity and pharmacokinetics of dexamethasone after intravitreal injection. Arch Ophthalmol. 1992;110(2):259–266. doi:10.1001/archopht.1992.01080140115038.
- Kim LA, D'Amore PA. A brief history of anti-VEGF for the treatment of ocular angiogenesis. Am J Pathol. 2012;181(2):376–379. doi:10.1016/j.ajpath.2012.06.006.
- El Sanharawi M, Kowalczuk L, Touchard E, Omri S, De Kozak Y, Behar-Cohen F. Protein delivery for retinal diseases: from basic considerations to clinical applications. Prog Retin Eye Res. 2010;29(6):443–465. doi:10.1016/j.preteyeres.2010.04.001.
- Herrero-Vanrell R, Bravo-Osuna I, Andrés-Guerrero V, Vicario-de-la-Torre M, Molina-Martínez IT. The potential of using biodegradable microspheres in retinal diseases and other intraocular pathologies. Prog Retin Eye Res. 2014;42:27–43. doi:10.1016/j.preteyeres.2014.04.002.
- Kang-Mieler JJ, Dosmar E, Liu W, Mieler WF. Extended ocular drug delivery systems for the anterior and posterior segments: biomaterial options and applications. Expert Opin Drug Deliv. 2017;14(5):611–620. doi:10.1080/17425247.2016.1227785.
- Holekamp NM, Campochiaro PA, Chang MA, Miller D, Pieramici D, Adamis AP, Brittain C, Evans E, Kaufman D, Maass KF, et al. Archway randomized phase 3 trial of the port delivery system with ranibizumab for neovascular age-related macular degeneration. Macular Degener Ophthalmol. 2021;36(3):488–489.
- Sharma A, Parachuri N, Kumar N, Kuppermann BD, Bandello F. The port delivery system with ranibizumab—journey of mitigating vitreous hemorrhage. Eye. 2022;36(3):488–482. doi:10.1038/s41433-021-01830-5.
- Osswald CR, Kang-Mieler JJ. Controlled and extended release of a model protein from a microsphere-hydrogel drug delivery system. Ann Biomed Eng. 2015;43(11):2609–2617. doi:10.1007/s10439-015-1314-7.
- Osswald CR, Kang-Mieler JJ. Controlled and extended in vitro release of bioactive anti-vascular endothelial growth factors from a microsphere-hydrogel drug delivery system. Curr Eye Res. 2016;41(9):1216–1222. doi:10.3109/02713683.2015.1101140.
- Liu W, Borrell MA, Venerus DC, Mieler WF, Kang-Mieler JJ. Characterization of biodegradable microsphere-hydrogel ocular drug delivery system for controlled and extended release of ranibizumab. Transl Vis Sci Technol. 2019;8(1):12. doi:10.1167/tvst.8.1.12.
- Liu W, Lee BS, Mieler WF, Kang-Mieler JJ. Biodegradable microsphere-hydrogel ocular drug delivery system for controlled and extended release of bioactive aflibercept in vitro. Curr Eye Res. 2019;44(3):264–274. doi:10.1080/02713683.2018.1533983.
- Liu W, Tawakol AP, Rudeen KM, Mieler WF, Kang-Mieler JJ. Treatment efficacy and biocompatibility of a biodegradable aflibercept-loaded microsphere-hydrogel drug delivery system. Transl Vis Sci Technol. 2020;9(11):13. doi:10.1167/tvst.9.11.13.
- Kim S, Kang-Mieler JJ, Liu W, Wang Z, Yiu G, Teixeira LB, Mieler WF, Thomasy SM. Safety and biocompatibility of aflibercept-loaded microsphere thermo-responsive hydrogel drug delivery system in a nonhuman primate model. Transl Vis Sci Technol. 2020;9(3):30. doi:10.1167/tvst.9.3.30.
- Gómez-Gaete C, Tsapis N, Besnard M, Bochot A, Fattal E. Encapsulation of dexamethasone into biodegradable polymeric nanoparticles. Int J Pharm. 2007;331(2):153–159. doi:10.1016/j.ijpharm.2006.11.028.
- Drapala PW, Jiang B, Chiu YC, Mieler WF, Brey EM, Kang-Mieler JJ, Pérez-Luna VH. The effect of glutathione as chain transfer agent in PNIPAAm-based thermo-responsive hydrogels for controlled release of proteins. Pharm Res. 2014;31(3):742–753. doi:10.1007/s11095-013-1195-0.
- Hickey T, Kreutzer D, Burgess DJ, Moussy F. Dexamethasone/PLGA microspheres for continuous delivery of an anti-inflammatory drug for implantable medical devices. Biomaterials. 2002;23(7):1649–1656. doi:10.1016/s0142-9612(01)00291-5.
- Giteau A, Venier-Julienne MC, Aubert-Pouëssel A, Benoit JP. How to achieve sustained and complete protein release from PLGA-based microparticles? Int J Pharm. 2008;350(1-2):14–26. doi:10.1016/j.ijpharm.2007.11.012.
- Cao J, Naeem M, Noh JK, Lee EH, Yoo JW. Dexamethasone phosphate-loaded folate-conjugated polymeric nanoparticles for selective delivery to activated macrophages and suppression of inflammatory responses. Macromol Res. 2015;23(5):485–492. doi:10.1007/s13233-015-3065-6.
- Arya SK, Wong-Staal F, Gallo RC. Dexamethasone-mediated inhibition of human T cell growth factor and gamma-interferon messenger RNA. J Immunol. 1984;133(1):273–276.
- Culpepper JA, Lee F. Regulation of IL 3 expression by glucocorticoids in cloned murine T lymphocytes. J Immunol. 1985;135(5):3191–3197.
- Lewis GD, Campbell WB, Johnson AR. Inhibition of prostaglandin synthesis by glucocorticoids in human endothelial cells. Endocrinology. 1986;119(1):62–69. doi:10.1210/endo-119-1-62.
- Grabstein K, Dower S, Gillis S, Urdal D, Larsen A. Expression of interleukin 2, interferon-gamma, and the IL 2 receptor by human peripheral blood lymphocytes. J Immunol. 1986;136(12):4503–4508.
- Knudsen PJ, Dinarello CA, Strom TB. Glucocorticoids inhibit transcriptional and post-transcriptional expression of interleukin 1 in U937 cells. J Immunol. 1987;139(12):4129–4134.
- Lee SW, Tsou AP, Chan H, Thomas J, Petrie K, Eugui EM, Allison AC. Glucocorticoids selectively inhibit the transcription of the interleukin 1 beta gene and decrease the stability of interleukin 1 beta mRNA. Proc Natl Acad Sci USA. 1988;85(4):1204–1208. doi:10.1073/pnas.85.4.1204.
- Haller JA, Bandello F, Belfort R, Jr Blumenkranz MS, Gillies M, Heier J, Loewenstein A, Yoon YH, Jiao J, Li XY, et al. Dexamethasone intravitreal implant in patients with macular edema related to branch or central retinal vein occlusion: twelve-month study results. Ophthalmol. 2011;118(12):2453–2460. doi:10.1016/j.ophtha.2011.05.014.
- Katime I, Novoa R, Zuluaga F. Swelling kinetics and release studies of theophylline and aminophylline from acrylic acid/n-alkyl methacrylate hydrogels. Euro Polym J. 2001;37(7):1465–1471. doi:10.1016/S0014-3057(01)00004-0.
- Sah H. Protein instability toward organic solvent/water emulsification: implications for protein microencapsulation into microspheres. PDA J Pharm Sci Tech. 1999;53(1):3–10.
- Li X, Zhang Y, Yan R, Jia W, Yuan M, Deng X, Huang Z. Influence of process parameters on the protein stability encapsulated in poly-DL-lactide–poly (ethylene glycol) microspheres. J Control Release. 2000;68(1):41–52. doi:10.1016/S0168-3659(00)00235-2.
- Varshochian R, Jeddi-Tehrani M, Mahmoudi AR, Khoshayand MR, Atyabi F, Sabzevari A, Esfahani MR, Dinarvand R. The protective effect of albumin on bevacizumab activity and stability in PLGA nanoparticles intended for retinal and choroidal neovascularization treatments. Eur J Pharm Sci. 2013;50(3–4):341–352. doi:10.1016/j.ejps.2013.07.014.
- He J, Feng M, Zhou X, Ma S, Jiang Y, Wang Y, Zhang H. Stabilization and encapsulation of recombinant human erythropoietin into PLGA microspheres using human serum albumin as a stabilizer. Int J Pharm. 2011;416(1):69–76. doi:10.1016/j.ijpharm.2011.06.008.
- Bilati U, Allémann E, Doelker E. Strategic approaches for overcoming peptide and protein instability within biodegradable nano- and microparticles. Eur J Pharm Biopharm. 2005;59(3):375–388. doi:10.1016/j.ejpb.2004.10.006.
- van de Weert M, Hoechstetter J, Hennink WE, Crommelin DJ. The effect of a water/organic solvent interface on the structural stability of lysozyme. J Control Release. 2000;68(3):351–359. doi:10.1016/S0168-3659(00)00277-7.
- van de Weert M, Hennink WE, Jiskoot W. Protein instability in poly (lactic-co-glycolic acid) microparticles. Pharm Res. 2000;17(10):1159–1167.
- Won JY, Kim J, Gao G, Kim J, Jang J, Park YH, Cho DW. 3D printing of drug-loaded multi-shell rods for local delivery of bevacizumab and dexamethasone: a synergetic therapy for retinal vascular diseases. Acta Biomater. 2020;116:174–185. doi:10.1016/j.actbio.2020.09.015.