Abstract
Purpose
Loss of corneal transparency is one of the major causes of visual loss, generating a considerable health and economic burden globally. Corneal transplantation is the leading treatment procedure, where the diseased cornea is replaced by donated corneal tissue. Despite the rise of cornea donations in the past decade, there is still a huge gap between cornea supply and demand worldwide. 3D bioprinting is an emerging technology that can be used to fabricate tissue equivalents that resemble the native tissue, which holds great potential for corneal tissue engineering application. This study evaluates the manufacturability of 3D bioprinted acellular corneal grafts using low-cost equipment and software, not necessarily designed for bioprinting applications. This approach allows access to 3D printed structures where commercial 3D bioprinters are cost prohibitive and not readily accessible to researchers and clinicians.
Methods
Two extrusion-based methods were used to 3D print acellular corneal stromal scaffolds with collagen, alginate, and alginate-gelatin composite bioinks from a digital corneal model. Compression testing was used to determine moduli.
Results
The printed model was visually transparent with tunable mechanical properties. The model had central radius of curvature of 7.4 mm, diameter of 13.2 mm, and central thickness of 0.4 mm. The compressive secant modulus of the material was 23.7 ± 1.7 kPa at 20% strain. 3D printing into a concave mold had reliability advantages over printing into a convex mold.
Conclusions
The printed corneal models exhibited visible transparency and a dome shape, demonstrating the potential of this process for the preparation of acellular partial thickness corneal replacements. The modified printing process presented a low-cost option for corneal bioprinting.
Introduction
Corneal disease is one of the leading causes of vision loss worldwide.Citation1–4 Transplantation of human donor corneas remains the gold standard, with over 180,000 transplants performed worldwide annually.Citation5 Despite the prevalence of corneal disease, there is an enormous discrepancy between the number of corneas donated and the number of people who need a cornea transplant, and graft rejection can occur.Citation6 Globally, it is estimated that 12.7 million people require a corneal transplant, with only 1 cornea available for every 70 needed.Citation5 This substantial gap between cornea supply and demand, combined with advancements in tissue engineering, has accelerated the need for development of artificial cornea substitutes.
Advancements have been made in the field of 3D bioprinting that present a potential solution to this problem. 3D bioprinting is the process in which established 3D printing techniques are adapted for printing of biomaterials.Citation7 The desired product is generated layer by layer with a selected biomaterial that is precisely extruded in space using a 3D positioning system.
There are many advantages of 3D bioprinting when compared to other biofabrication techniques that have been used to create corneal substitutes.Citation7–10 First, 3D bioprinting provides precise control over the architecture of the printed tissue construct, both through computer-aided position control and advanced material dispensing techniques. The material properties of a product are also easily controlled in 3D bioprinting, as seen with ability to print several biomaterials in addition to modifications of extrusion rate, printing speed and infill percentages. Further, 3D models can be generated based on diagnostic imaging using a variety of technologies, making it feasible to create patient-specific implants that fit a patient’s unique anatomical features, which would be especially beneficial for the curved cornea.Citation7
Many bioprinted corneas are being prepared using expensive commercial bioprinters, or customized industrial robots. The high cost of some of these systems (> $50,000 USD) can present a huge roadblock for potential wide adoption by both the research and clinical communities. Additionally, current research employing bioprinting strategies has difficulties achieving the desired optical properties for a corneal substitute,Citation11 since other parameters (mechanical properties, tissue compatibility, cytotoxicity) must also be considered.Citation12 The final corneal substitute should show transparency properties that are comparable to the human cornea. For the human cornea, transparency increases from almost 0% at 300 nm and shorter wavelengths to 80% at 380 nm and levels at greater than 90% between 500 nm and 1300 nm to decrease sharply at wavelengths greater than 1300 nm.Citation13 To create a corneal substitute, one has to consider multiple areas which have been addressed in the work of various research groups. This involves the evaluation of the various printing technologies, i.e. extrusion, LASER, DLP, Inkjet,Citation14 along with biomaterials research which involves crosslinking technologyCitation15–17 and the stability and cytotoxic effects on cellular material in the printing process.Citation18,Citation19
The objective of the current study was to investigate 3D bioprinting of corneal stroma scaffolds using low-cost desktop technology and open-source software. The specific target is partial thickness corneal transplant applications rather than full corneal substitution. For example, in a deep anterior lamellar transplant, an approximately 8 mm diameter corneal button is replaced with a partial thickness donor graft. This procedure is used when the pathology is in the anterior layers and the endothelium is intact. Native cells populate the graft in the post-operative period, which means a 3D printed partial thickness corneal construct would not require cells to be embedded during the printing process, but rather require acceptable transparency and customizable mechanical properties. These design criteria are essential to provide clear vision in terms of refractive power with minimal scatter, as well as structural support of the pressurized globe.
The current study looks at the components within the manufacturing process to demonstrate that while utilizing materials with known low toxicity and established processes from the literature, that with low cost of the components, equipment, and software, it is possible to produce an acceptable acellular corneal graft. With that in mind, we considered synthetic extrudable hydrogels which have been researched over the past 10–15 years for biological applications.Citation20 Therefore, the ultimate purpose of this study was to investigate the feasibility of 3D printing biopolymers with a low-cost desktop 3D printer and open-source software with potential for future application in corneal repair.
Materials and methods
Preparation of digital corneal model
The datasets defining the anterior and posterior surfaces were taken from the literature to generate the digital spherical model of the cornea used for 3D bioprinting.Citation21 The target printing parameters were based on an average range of values for an adult human cornea: 7.10–8.75 mm for radius of curvature, 10–12 mm for overall diameter, and 0.48–0.62 mm for central thickness.Citation22,Citation23 A CAD model of the cornea was created in SolidWorks (Waltham, MA), with the point data imported directly to ensure the appropriate dimensions and thickness profile of the model. The CAD model was then converted into an STL file and uploaded to Slic3r, an open-source 3D printing software capable of generating G-code by digitally slicing STL models into thin layers.
Creation of the support molds
From the CAD corneal model, concave and convex 3D molds were created in SolidWorks. These molds were used to support the cornea’s shape during printing and curing. The central curvature of the molds was calculated using the data for anterior (for the concave mold) or posterior (for the convex mold) surfaces from the digital corneal model. The CAD model of the molds was exported as an STL file and was 3D printed on a Formlabs 3D printer (Form 2, Formlabs, Somerville, MA).
Printer modification
A modified printer configuration was used to extrude the bioink. A relatively low cost LulzBot Mini 2 Desktop 3D printer (Aleph Objects Inc., Loveland, CO) was modified for use with a syringe-based extruder following the protocol described by Pusch et al.Citation24 A 2.5 mL glass syringe (Hamilton Company, Reno, NV) was used with a 21-gauge needle (Jensen Global, Santa Barbara, CA) to extrude the bioink. Two different printing methods were evaluated in this study: 1) extrusion based bioprinting using a modified Freeform Reversible Embedding of Suspended Hydrogels (FRESH) support method including a cornea shaped support mold for the extruded bioink, and 2) extrusion based bioprinting without the FRESH support but with the corneal shaped support mold.
Bioink preparation
The first method of printing evaluated, modified FRESH, utilized both a collagen-based and alginate-based bioink. For our modified FRESH printing, the FRESH starter kit and the support slurry were prepared following the product manual (Advanced Biomatrix, Carlsbad, CA). To prepare the collagen-based bioink, 5.4 mL LifeInk200 type I collagen (Advanced Biomatrix) was dissolved in 2.7 mL 0.24 M acetic acid, yielding a final concentration of 23 mg/mL. To ensure crosslinking of collagen into a gel after extrusion, the FRESH support bath was supplemented with 10 mM HEPES to maintain a pH of ∼7.4 and to neutralize the acetic acid. To prepare the alginate-based bioink, 2% (w/v) sodium alginate (Sigma) was mixed with 2% (w/v) CaCO3 to create a homogeneous mixture. D-Glucono-delta-lactone (GDL) at 2% (w/v) was added to the FRESH support bath to facilitate alginate crosslinking. After printing, samples were incubated at 37 °C for 30 min to remove the FRESH support material. Samples were then washed with PBS and stored in Petri dishes with the samples submerged in PBS.
For the second method of bioprinting, a bioink was prepared using gelatin and alginate. 10% (w/v) type A gelatin from porcine skin (Millipore Sigma, St. Louis, MO) was combined with 1% (w/v) sodium alginate (Millipore Sigma). The mixture was maintained at 65 °C on a stirring hot plate until addition to the bioprinter. Additional crosslinking was performed by adding calcium chloride (CaCl2) with a dropper after the completion of printing.
3D Printing
An established patient-specific digital corneal model was used for 3D printing.Citation21 The model was constructed using a rotating Scheimpflug camera with a Placido disk and was exported as an STL file (). The diameter of the corneal model measured 13.13 mm, while its thickness measured approximately 390 μm at the center and 950 μm towards the periphery. The STL file was then processed by Slic3r software and sliced into 60–200 µm-thick layers to generate G-code for the 3D printer ( and ). The G-code sets were finally sent to the printer using Printrun, an open-source 3D printer host interface. 3D printing was performed using a LulzBot Mini 2 desktop 3D printer modified with a syringe-based extruder (), following Feinberg’s protocol.Citation24 A 2.5 mL syringe (Hamilton) and needles (Jensen Global) with gauge sizes of 30 G (collagen) and 22 G (alginate/gelatin) were used for material extrusion.
Figure 1. Schematic showing the low-cost cornea bioprinting process. A digital corneal model was derived from patient data and was used for generating CAD models of both corneal scaffolds and support molds. A modified desktop 3D printer was used to print cornea structures with the aid of the support mold. A mold holder was used in conjunction with a custom start G-code to facilitate the initial positioning.
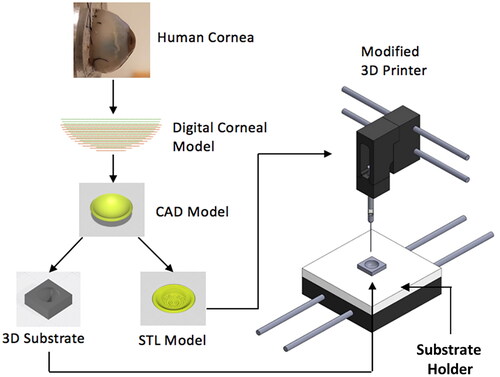
The first process applied the FRESH printing technique and was used to print collagen bioink. The bioink was extruded at 6 mm/s into the support slurry. Part infill density was set at 100% and concentric infill mode was applied. After printing, scaffolds were incubated at 37 °C for 30 min to further crosslink the collagen and melt the support bath.
The second process printed alginate/gelatin composite bioinks on a polymer mold with predefined surface geometry. The print settings were altered in Slic3r to print the desired cornea shape. The print speed was set to 4 mm/s, and an infill density of 60% in a concentric pattern was applied. The extrusion multiplier (EM) was set to 75% (EM = original EM x set wall thickness/actual wall thickness). For these initial prints, the layer thickness was set to 200 µm. The G-code generated by Slic3r was sent to Printrun to print the model. During printing, the bioink was kept at a constant temperature to maintain print consistency. To achieve this, a polyimide insulated flexible heater (Omega Engineering Inc., Norwalk, CT) was affixed to the syringe. The heater was connected to the printer’s heating unit and the printer settings were adjusted to keep the bioink at 33 °C. After printing, the corneal model was crosslinked with a 2% (w/v) CaCl2 solution for 5 min to increase scaffold integrity and prevent dissolution. The solution was then aspirated and replaced with an equal volume of PBS solution for storage prior to additional testing.
Mechanical testing and data analysis
To verify the mechanical properties of the alginate/gelatin bioink, compression tests were performed using a cylindrical material sample of 20 mm diameter and 5 mm height. Materials and printing parameters remained the same as the corneal models. All the measurements were performed in triplicate. Pseudo-static compressive tests were performed using a 100 Series Electromechanical Universal Test Machine (TestResources, Shakopee, MN) with a 0.5 N load cell. The compressive strain was increased from 0 to 40% at a constant loading rate. The compressive force was measured during the entire loading period, where the compressive stress was derived. The experimental force/distance measurements were translated into stress/strain data and curve-fitted with third order polynomials to calculate the compressive modulus at specific strain rates. The results are represented as mean ± standard deviation from three measurements and compared to values from the literature.
Results
A digital corneal model was successfully created by the schematic shown in with promising transparency properties and tunable mechanical properties. The final printed model had a central radius of curvature of 7.41 mm, an overall diameter of 13.20 mm, and a central thickness of 0.39 mm, which is comparable to the target values. The average range of these values for an adult human cornea are 7.10–8.75 mm for radius of curvature, 10–12 mm for overall diameter, and 0.48–0.62 mm for central thickness.Citation22,Citation23 The initial model prepared had a slightly larger diameter and a thinner central thickness than the targets.
We demonstrated that the central radius of curvature values could also be adapted to fit a patient’s specific anatomy (). As a proof of concept, it was determined that multiplying the curvature data from the original model by a factor of 1.05 created a spherical corneal model with a radius of curvature that matched the average anterior radius of curvature in an adult human cornea (7.79 mm)Citation23 (). The original model was also modified to create two alternative shaped corneal models. Greater radius of curvature corresponds to flatter curvature. A flatter model was made by multiplying the original radius of curvature data by a factor of 1.18 (), which matched the upper bound of the published human cornea radii of curvature (8.65 mm). On the contrary, a steeper model was created by multiplying by a factor of 0.96 (), which matched the lower bound of the range (7.10 mm).
Figure 2. Digital corneal models with (0,0) as center, x is distance from the center, and y is through-thickness depth relative to plane at apex. (A) Cloud point curves defining the meridian section of corneal models with different radii of curvature. Data were derived from the original corneal model. 3D corneal models created from the cloud point data in (A), with model configurations matching (B) human average values, (C) human lower bound values (steep), and (D) upper bound values (flat), of the human cornea radii of curvature.
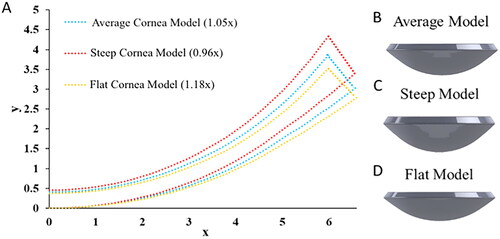
The 3D printing system used in this study was modified from a low-cost desktop 3D printer (LulzBot Mini 2, $1,500 USD MSRP) following an established protocol ().Citation24 The built-in extrusion head of the 3D printer was replaced with a syringe-based extruder to allow for the bioink to be extruded using the low-cost syringe ($102). The syringe-based extruder consisted of a core, a geared leadscrew transmission, and a plunger adapter assembled from 3D printed parts (). During printing, the syringe was secured inside the core. The built-in step motor turned the gear which pushed the plunger adapter down on the syringe plunger to extrude the bioink. The temperature was controlled by a polyimide flexible heater ($54). The total system cost was less than $2,000. shows the cost compilation of the printer used and the components used to modify the printer.
Figure 3. Low-cost cornea bioprinting setup. (A) Modified 3D printer. (B) Syringe-based extruder assembled from 3D-printed parts. (C) A 3D-printed concave substrate. (D) A 3D-printed convex substrate.
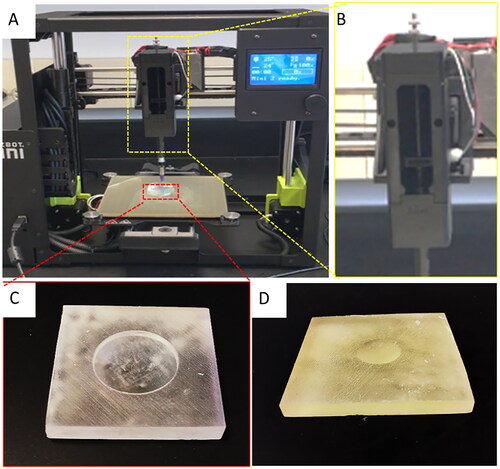
Table 1. Compilation of costs associated with graft production.
Based on previous work we selected the initial material combinations (collagen and alginate) using the preparation specifications from literature. Since the resulting optical properties of the FRESH printed corneal grafts were inadequate due to porosity, we abandoned the FRESH process and printed the composite hydrogel material (gelatin and alginate) also based on literature studies.
Another factor that affected printing consistency was the corneal geometry. Extrusion-based 3D bioprinting works by depositing a selected bioink layer by layer to create a 3D object. In this method, each new layer must be supported by the layer beneath it. The corneal model, however, has a dome-like shape where most of its surfaces are not supported by anything below, which causes print failure. To address this challenge, we designed printing molds to support the cornea’s shape during printing and curing. A concave mold was used as a print support to print the cornea from the center to the periphery (). In this way, the printed cornea had a better-defined anterior surface. The convex mold was used as a print support to print the cornea in the opposite direction, moving from the periphery to the center, that resulted in a precise posterior surface (). The concave mold demonstrated better results, as material sometimes did not fill in the center of the cornea while utilizing the convex mold. Other mold configurations can be easily made to accommodate the need for variant cornea dimensions and curvatures.
When using the mold to support cornea printing, it is important to ensure that the print starts at the right position. Otherwise, the needle may hit the mold wall in the middle of printing, causing printing failure. Manual positioning of the printhead is inefficient and results in inconsistencies. Therefore, we developed a solution by using a mold holder in conjunction with a custom start G-code. Prior to each print, the mold was placed in the holder and the holder was secured to the print bed (). The mold holder ensured that the mold was placed directly in the center of the print bed, and the custom start G-code defined the initial movement of the printhead so that the needle tip was positioned right at the bottom center of the mold (in the case of a concave mold) before the print started. This setup automated the initial positioning process which eliminated previous complications associated with manually positioning the syringe needle before each print.
The aforementioned process allowed the printing of the corneal models by two techniques with the appropriate printing technique and optimal material. To understand the underlying causes of lack of transparency in previously published reports, we first tested printing of collagen- and alginate-based bioinks into the gelatin support following a FRESH protocol (). As a result, both collagen- and alginate-based structures showed poor transparency (). Microscopic examination revealed high porosity and many air bubbles embedded within the structure (). Presumably, this is because the support slurry that had intermixed with the bioink was composed of gelatin microparticles. When they liquefied during post-incubation and were washed away, a large number of voids were left in the printed structure and were later filled with air. Consequently, the mismatch of refractive indexes between air and the polymeric bioink material led to the opacities.Citation25 In contrast, our second printing method does not involve a sacrificial material – the extruded bioink directly forms desired shapes on the mold. The enhanced material continuity across the printed structure thus guarantees a high level of transparency ().
Figure 4. Comparison of optical properties of the corneal structures printed with the modified FRESH process and the standard bioprinting method. (A) FRESH printed corneal structures showing well preserved cornea shape. (B-C) Overview and microscopic images of FRESH printed corneal structures with a collagen-based bioink. (D-E) Overview and microscopic images of FRESH printed corneal structures with an alginate-based bioink. (F) Corneal structures printed with the standard (presented) bioprinting method.
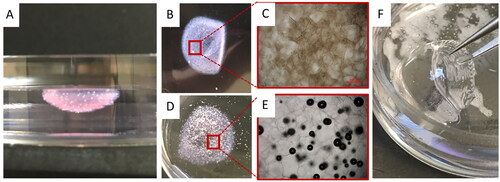
The FRESH printing method was not further pursued due to its optical properties (). As shown in , by using a standard printing method, the printed corneal structure retained its dome-like shape after printing and post-curing. The cross-section view showed a thin profile at the center region and a thicker peripheral (), which is in good qualitative agreement with the digital corneal model. The printed corneal structure also had smooth surfaces, as can be seen from the reflection of green (540 nm) and blue (480 nm) fluorescent lights at the cornea surfaces (). Moreover, the printed corneal structure exhibited qualitatively acceptable transparency, as text could be clearly viewed through the artificial corneas both soaked in PBS solution () and exposed to air ().
Figure 5. 3D structure of bioprinted corneal scaffolds and their optical properties. (A-C) Top, bottom and section view of the printed corneal structure. (D-E) The printed corneal structures were exposed to green (540 nm) and blue (480 nm) fluorescent illumination, showing overall dome shape. (F-G) Depiction of transparency of the printed corneal structures in liquid and air, respectively.
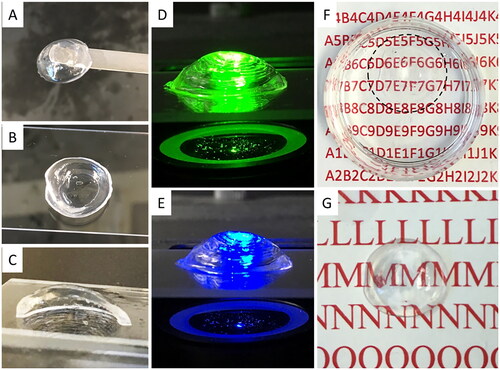
Mechanical testing was performed on a cylinder test coupon of the alginate/gelatin composite bioink to determine the bioprinted material’s mechanical properties (). As a result, the compressive secant modulus of the test coupon was calculated as 23.7 ± 1.7 kPa () at 20% strain, and the tangent modulus at 20% strain was calculated as 42.2 ± 9.0 kPa (). With our method, mechanical properties of the printed structure can be tuned by adjusting the ratio of alginate to gelatin in the bioink formulation, using an alginate with different molecular weight, or varying the crosslinking ratio.Citation26 The aforementioned stiffness, for instance, was obtained from a 1% alginate / 10% gelatin bioink. These preliminary results indicate the potential capability to customize the printed structure’s mechanical properties.
Figure 6. Mechanical properties of the printed structures. (A) Compressive stress-strain results of a 3D printed cylindrical test coupon (shown in the inset). (B) Compressive secant modulus of the test coupon. (C) Compressive tangent modulus of the test coupon. Error bars represent standard deviations for 3 experimental replicates.

Discussion
This study demonstrated the ability to create an appropriate radius of curvature within the range of physiological corneal dimensions. For these initial proof-of-concept prints, the layer thickness was set to 200 µm. Future work will focus on reducing the thickness while maintaining mechanical integrity. Additionally, the dimensional factors could be adjusted to accommodate the needs for different surgical procedures. For instance, penetrating keratoplasty (PK) requires a full-thickness corneal graft, whereas deep anterior lamellar keratoplasty (DALK) involves only partial corneal thickness above the endothelium and Descemet’s membrane.Citation27
This study additionally aimed to show that low-cost options exist to 3D print corneal scaffolds (). The major component was the 3D printer ($1,500 USD MSRP). Printer modifications included using a glass syringe ($102) and addition of a polyimide insulated flexible heater ($54) to the syringe. This heater could be powered by the printer’s built-in heating unit and controlled by the printer settings, avoiding the need for external controllers that may increase the total cost of typical bioprinters. The cost of these printer modifications was under $200. Relatively inexpensive biopolymers were selected (gelatin, $44 per 100 g, collagen $260 per 5 mL, and alginate $14 per 100 g), bringing the total system cost (printer + modification + syringe + heater + mold) to approximately $2,000. Compared to other methods that require commercial bioprinters or customized industrial robots that could cost upwards of $50,000, our proposed cornea bioprinting solution significantly reduced the cost, making it more easily accessible to the research and clinical communities.
In our preliminary studies, particularly using the FRESH method, ensuring printing consistency and optical transparency were major issues. For extrusion-based 3D printing, printing consistency is largely affected by the material viscosity. If the bioink is too viscous, it tends to clog the needle and thus causes discontinuity in extrusion. The bioink that is less viscous, on the other hand, can cause the extruded filaments to collapse under their own weight during printing. Both collagen and gelatin, major components in our bioinks, are temperature-sensitive materials whose viscosity changes dramatically as the temperature fluctuates.Citation28 As such, temperature management during the printing process was critical to achieve the desired print consistency. To this end, we used a polyimide insulated flexible heater to keep the bioink at a printable temperature (e.g. 33 °C for the 1% alginate / 10% gelation bioink) for the duration of the print.
For these studies, relatively inexpensive and commonly used biopolymers were selected for proof-of-concept test prints. Collagen has been widely used and studied as a biomaterial due to its capability to act as a scaffold for cell attachment, although collagen exhibits a low mechanical strength when used as a bioink. Gelatin, especially in the form of gelatin methacrylate (GelMA), has also been extensively studied and due to its origin also exhibits a low toxicity. It can be tuned to provide an acceptable strength in the printing process.Citation29,Citation30 Alginate has shown great potential recently for bioengineered materials for corneal tissue repair and engineering due to its adaptability, transparency, tunable crosslinking, and low immunogenicity.Citation11,Citation31 Compressive modulus was calculated at 20% strain and was found to be 23.7 ± 1.7 kPa (secant) and 42.2 ± 9.0 kPa (tangent) for a blend of 1% alginate with 10% gelatin in the bioink. The tangent modulus value is higher than that found in a previous report which evaluated a 0.5% agarose / 0.2% collagen scaffold and measured a tangent modulus of 18.1 ± 3.5 kPa,Citation32 but still below the compressive stiffness of native human corneal tissue, which is about 300 kPa.Citation33 The native corneal tissue has a compressive modulus approximately 10x higher, and this can be targeted in future studies by adjusting the ratios of alginate to gelatin in the bioink, changing alginate molecular weight, or increasing the degree or chemical nature of crosslinking. Further, chemical modifications or blending of the alginate with other functional compounds with stronger chemical crosslinking can further facilitate tuning of its mechanical properties in addition to controlling scaffold degradation rate and modulating biological activity, if desired.
Transparency is one of the most important criteria for evaluating any corneal replacements or tissue engineering products. The main disadvantage of past studies in cornea bioprinting was less than ideal optical properties of the printed constructs.Citation34,Citation35 For instance, Isaacson et al. reported bioprinting of corneal structures from a collagen-based bioink using the modified FRESH method. The FRESH method involves deposition of a selected hydrogel bioink into a thermoreversible support bath, which helps maintain the hydrogel’s 3D structure during the print process. After printing, the support can be molten and washed away. In our case, the 3D printed structure remained on the mold.Citation36 In Isaacson’s report, the printed structures showed good preservation of the corneal shape, but they only exhibited low-to-moderate degree of transparency. In the current study, we were able to increase the transparency of the printed corneal structures due to the reduced porosity in the extrusion-based printing method without support gel.
This proof-of-concept study evaluated different bioinks and demonstrated that a bioprinted scaffold could be created using open-source software and low-cost equipment. The mechanical and optical properties, particularly increased transparency, showed promising characteristics for potential application for corneal repair. This study did have several limitations, including qualitative assessment of optical transparency, only evaluating mechanical properties under compression, and only varying central radius of curvature of the printed scaffolds. The target was an acellular corneal stroma for potential use in lamellar cornea procedures, so cell incorporation was not evaluated. The materials used have been extensively evaluated in vitro with corneal cells and in vivo for other ocular applications, so cytocompatibility and toxicity results can be found readily in the literature.Citation11,Citation37–39 Further evaluation of these parameters was outside of the scope of this study.
As it has been shown in previous work, the inclusion of cellular material into the bioink requires additional considerations to maintain cellular viability during the extrusion process, since fibrillar alignment of the bioink components due to shear stress (desired) can be detrimental to cellular viability (not desired).Citation19 We therefore elected to use an acellular approach to create the scaffolds and we selected materials which have been thoroughly evaluated for cytotoxicity in the literature.Citation29,Citation31 Islam et al. have shown in clinical work that cellular migration into acellular corneal scaffolds happens over time,Citation40 demonstrating the potential for an acellular partial thickness corneal graft.
Various research directions can be observed in addressing corneal defect repair. Looking at the clinical applications, several groups have been working on suture free materials as corneal sealants.Citation16,Citation41 Other groups are investigating the incorporation of cellular material.Citation19,Citation42,Citation43 Further, others are looking at material creation to address the mechanical, optical, and biological challenges of corneal repair, i.e. the generation of orthogonally crosslinked polymer hydrogels from different base materials.Citation18,Citation41 In this study, we wanted to focus on the methods to create a 3D printed material which has the potential to be utilized as an acellular corneal graft. Recent studies have demonstrated methods of 3D printing corneal equivalent models for corneal tissue engineering.Citation32,Citation34,Citation35,Citation44,Citation45 Duarte Campos et al. presented a drop-on-demand bioprinting strategy for creating a collagen-based corneal stromal model.Citation32 Sorkio et al. introduced a laser-assisted bioprinting (LaBP) process to create corneal equivalent structures.Citation35 Isaacson et al. used the modified FRESH method to print cornea substitutes by extruding collagen-based bioinks to form corneal shapes in a thermoreversible support bath.Citation34 Kim et al. demonstrated with their recent corneal sealant development that cellular migration happens into an appropriate corneal ECM structure, further confirming the results of Islam et al.Citation16,Citation40 The current study built upon these previous experiments to produce an acellular corneal stroma for potential use in a lamellar cornea procedure.
Future work will focus on evaluating detailed mechanical, optical, and biological parameters. In particular, determining the range of mechanical properties that can be achieved while maintaining the bioprinted material’s geometric fidelity and optical properties will be beneficial for corneal applications. Mechanical testing should also focus on tensile strength, in addition to increasing the modulus, while evaluating suitability for suturing. Optical properties to be quantified for corneal application include light transmission and refractive index. Once these parameters are determined, scaffolds will be assessed for corneal and stromal cell growth, cytotoxicity, and degradation rate, prior to assessing tissue compatibility in vivo. Additionally, other promising materials will be evaluated with these printing parameters. Additional bioprinter process parameters that can be further tuned in future studies include material shear behavior, depositing smaller and more precise layers, modulating microarchitecture, and in-process photocuring to determine the influence of strand and layer deposition.
Conclusions
In this study we successfully demonstrated a low-cost method to 3D print corneal stroma scaffolds for corneal tissue engineering. The nature of low cost and open source bioprinting makes this method widely accessible to the research community. The use of alginate-gelatin composite bioinks can also provide the flexibility to allow customizing the mechanical properties of the printed corneas. Future work will focus on fine tuning these mechanical properties through crosslinking and modifying printing patterns. A standard 3D printer was successfully modified with a syringe-based extrusion system that was capable of bioprinting corneal models with good transparency and dome shape, and acceptable material properties.
Acknowledgements
The authors want to acknowledge Professor Adam Feinberg for kindly providing the printer modification instructions and the 3D printing files for the syringe extruder components.
Disclosure statement
No potential conflict of interest was reported by the author(s).
Data availability statement
All data supporting the findings of this study are available from the corresponding author, Katelyn E. Swindle-Reilly, upon request.
Additional information
Funding
References
- Resnikoff S, Pascolini D, Etya’ale D, Kocur I, Pararajasegaram R, Pokharel GP, Mariotti SP. Global data on visual impairment in the year 2002. Bull World Health Organ. 2004;82(11):844–851.
- Pascolini D, Mariotti SP. Global estimates of visual impairment: 2010. Br J Ophthalmol. 2012;96(5):614–618. doi:10.1136/bjophthalmol-2011-300539.
- Resnikoff S, Keys TU. Future trends in global blindness. Indian J Ophthalmol. 2012;60(5):387–395. doi:10.4103/0301-4738.100532.
- Oliva MS, Schottman T, Gulati M. Turning the tide of corneal blindness. Indian J Ophthalmol. 2012;60(5):423–427. doi:10.4103/0301-4738.100540.
- Gain P, Jullienne R, He Z, Aldossary M, Acquart S, Cognasse F, Thuret G. Global Survey of Corneal Transplantation and Eye Banking. JAMA Ophthalmol. 2016;134(2):167–173. doi:10.1001/jamaophthalmol.2015.4776.
- Golchet G, Carr J, Harris MG. Why don’t we have enough cornea donors? A literature review and survey. Optometry. 2000;71(5):318–328.
- Murphy SV, Atala A. 3D bioprinting of tissues and organs. Nat Biotechnol. 2014;32(8):773–785. doi:10.1038/nbt.2958.
- Karamichos D, Hutcheon AE, Zieske JD. Reversal of fibrosis by TGF-β3 in a 3D in vitro model. Exp Eye Res. 2014;124:31–36. doi:10.1016/j.exer.2014.04.020.
- Li F, Carlsson D, Lohmann C, Suuronen E, Vascotto S, Kobuch K, Sheardown H, Munger R, Nakamura M, Griffith M. Cellular and nerve regeneration within a biosynthetic extracellular matrix for corneal transplantation. Proc Natl Acad Sci U S A. 2003;100(26):15346–15351. doi:10.1073/pnas.2536767100.
- Li F, Griffith M, Li Z, Tanodekaew S, Sheardown H, Hakim M, Carlsson DJ. Recruitment of multiple cell lines by collagen-synthetic copolymer matrices in corneal regeneration. Biomaterials. 2005;26(16):3093–3104. doi:10.1016/j.biomaterials.2004.07.063.
- Tarsitano M, Cristiano MC, Fresta M, Paolino D, Rafaniello C. Alginate-based composites for corneal regeneration: the optimization of a biomaterial to overcome its limits. Gels. 2022;8(7):431. doi:10.3390/gels8070431.
- Ulag S, Uysal E, Bedir T, Sengor M, Ekren N, Ustundag CB, Midha S, Kalaskar DM, Gunduz O. Recent developments and characterization techniques in 3D printing of corneal stroma tissue. Polym Adv Technol. 2021; Aug32(8):3287–3296. doi:10.1002/pat.5340.
- Meek KM, Knupp C. Corneal structure and transparency. Prog Retin Eye Res. 2015; Nov49:1–16. doi:10.1016/j.preteyeres.2015.07.001.
- Jia S, Bu Y, Lau DSA, Lin Z, Sun T, Lu WW, Lu S, Ruan C, Chan CHJ. Advances in 3D bioprinting technology for functional corneal reconstruction and regeneration. Front Bioeng Biotechnol. 2022;10:1065460. doi:10.3389/fbioe.2022.1065460.
- Chen F, Le P, Lai K, Fernandes-Cunha GM, Myung D. Simultaneous interpenetrating polymer network of collagen and hyaluronic acid as an in situ -forming corneal defect filler. Chem Mater. 2020;32(12):5208–5216. doi:10.1021/acs.chemmater.0c01307.
- Kim H, Jang JH, Han W, Hwang HJ, Jang J, Kim JY, Cho DW. Extracellular matrix-based sticky sealants for scar-free corneal tissue reconstruction. Biomaterials. 2023;292:121941. doi:10.1016/j.biomaterials.2022.121941.
- Fernandes-Cunha GM, Chen KM, Chen F, Le P, Han JH, Mahajan LA, Lee HJ, Na KS, Myung D. In situ-forming collagen hydrogel crosslinked via multi-functional PEG as a matrix therapy for corneal defects. Sci Rep. 2020;10(1):16671. doi:10.1038/s41598-020-72978-5.
- Hull SM, Lindsay CD, Brunel LG, Shiwarski DJ, Tashman JW, Roth JG, Myung D, Feinberg AW, Heilshorn SC. 3D Bioprinting using UNIversal Orthogonal Network (UNION) Bioinks. Adv Funct Mater. 2021;31(7):2007983. doi:10.1002/adfm.202007983.
- Kim H, Jang J, Park J, Lee KP, Lee S, Lee DM, Kim KH, Kim HK, Cho DW. Shear-induced alignment of collagen fibrils using 3D cell printing for corneal stroma tissue engineering. Biofabrication. 2019;11(3):035017. doi:10.1088/1758-5090/ab1a8b.
- Khosravimelal S, Mobaraki M, Eftekhari S, Ahearne M, Seifalian AM, Gholipourmalekabadi M. Hydrogels as emerging materials for cornea wound healing. Small. 2021;17(30):e2006335. doi:10.1002/smll.202006335.
- Simonini I, Pandolfi A. Customized finite element modelling of the human cornea. PLoS One. 2015;10(6):e0130426. doi:10.1371/journal.pone.0130426.
- Iyamu E, Osuobeni E. Age, gender, corneal diameter, corneal curvature and central corneal thickness in Nigerians with normal intra ocular pressure. J Optom. 2012;5(2):87–97. doi:10.1016/j.optom.2012.02.001.
- Mashige KP. A review of corneal diameter, curvature and thickness values and influencing factors. S Afr Optom. 2013;72(4):185–194. doi:10.4102/aveh.v72i4.58.
- Pusch K, Hinton TJ, Feinberg AW. Large volume syringe pump extruder for desktop 3D printers. HardwareX. 2018;3:49–61. doi:10.1016/j.ohx.2018.02.001.
- Shiwarski DJ, Hudson AR, Tashman JW, Feinberg AW. Emergence of FRESH 3D printing as a platform for advanced tissue biofabrication. APL Bioeng. 2021;5(1):010904. doi:10.1063/5.0032777.
- Freeman FE, Kelly DJ. Tuning alginate bioink stiffness and composition for controlled growth factor delivery and to spatially direct MSC fate within bioprinted tissues. Sci Rep. 2017;7(1):17042. doi:10.1038/s41598-017-17286-1.
- Söğütlü Sari E, Kubaloğlu A, Ünal M, Piñero Llorens D, Koytak A, Ofluoglu AN, Özertürk Y. Penetrating keratoplasty versus deep anterior lamellar keratoplasty: comparison of optical and visual quality outcomes. Br J Ophthalmol. 2012;96(8):1063–1067. doi:10.1136/bjophthalmol-2011-301349.
- Barrera C, Florián-Algarin V, Acevedo A, Rinaldi C. Monitoring gelation using magnetic nanoparticles. Soft Matter. 2010;6(15):3662–3668. doi:10.1039/c003284k.
- Guo Q, Phillip JM, Majumdar S, Wu P-H, Chen J, Calderón-Colón X, Schein O, Smith BJ, Trexler MM, Wirtz D, et al. Modulation of keratocyte phenotype by collagen fibril nanoarchitecture in membranes for corneal repair. Biomaterials. 2013;34(37):9365–9372. doi:10.1016/j.biomaterials.2013.08.061.
- Osidak EO, Kozhukhov VI, Osidak MS, Domogatsky SP. Collagen as Bioink for Bioprinting: a Comprehensive Review. Int J Bioprint. 2020;6(3):270.
- Kang D, Liu Z, Qian C, Huang J, Zhou Y, Mao X, Qu Q, Liu B, Wang J, Hu Z, et al. 3D bioprinting of a gelatin-alginate hydrogel for tissue-engineered hair follicle regeneration. Acta Biomater. 2023;165:197–199. doi:10.1016/j.actbio.2022.03.011.
- Duarte Campos DF, Rohde M, Ross M, Anvari P, Blaeser A, Vogt M, Panfil C, Yam GH-F, Mehta JS, Fischer H, et al. Corneal bioprinting utilizing collagen-based bioinks and primary human keratocytes. J Biomed Mater Res A. 2019;107(9):1945–1953. doi:10.1002/jbm.a.36702.
- Garcia-Porta N, Fernandes P, Queiros A, Salgado-Borges J, Parafita-Mato M, González-Méijome JM. Corneal biomechanical properties in different ocular conditions and new measurement techniques. ISRN Ophthalmol. 2014;2014:724546. doi:10.1155/2014/724546.
- Isaacson A, Swioklo S, Connon CJ. 3D bioprinting of a corneal stroma equivalent. Exp Eye Res. 2018;173:188–193. doi:10.1016/j.exer.2018.05.010.
- Sorkio A, Koch L, Koivusalo L, Deiwick A, Miettinen S, Chichkov B, Skottman H. Human stem cell based corneal tissue mimicking structures using laser-assisted 3D bioprinting and functional bioinks. Biomaterials. 2018;171:57–71. doi:10.1016/j.biomaterials.2018.04.034.
- Hinton TJ, Jallerat Q, Palchesko RN, Park JH, Grodzicki MS, Shue HJ, Ramadan MH, Hudson AR, Feinberg AW. Three-dimensional printing of complex biological structures by freeform reversible embedding of suspended hydrogels. Sci Adv. 2015;1(9):e1500758. doi:10.1126/sciadv.1500758.
- Maxwell CJ, Soltisz AM, Rich WW, Choi A, Reilly MA, Swindle-Reilly KE. Tunable alginate hydrogels as injectable drug delivery vehicles for optic neuropathy. J Biomed Mater Res A. 2022;110(10):1621–1635. doi:10.1002/jbm.a.37412.
- Poudel BK, Robert MC, Simpson FC, Malhotra K, Jacques L, LaBarre P, Griffith M. In situ tissue regeneration in the cornea from bench to bedside. Cells Tissues Organs. 2022;211(4):506–526. doi:10.1159/000514690.
- Chen Z, You J, Liu X, Cooper S, Hodge C, Sutton G, Crook JM, Wallace GG. Biomaterials for corneal bioengineering. Biomed Mater. 2018;13(3):032002. doi:10.1088/1748-605X/aa92d2.
- Islam MM, Buznyk O, Reddy JC, Pasyechnikova N, Alarcon EI, Hayes S, Lewis P, Fagerholm P, He C, Iakymenko S, et al. Biomaterials-enabled cornea regeneration in patients at high risk for rejection of donor tissue transplantation. NPJ Regen Med. 2018;3:2. doi:10.1038/s41536-017-0038-8.
- Chen F, Le P, Fernandes-Cunha GM, Heilshorn SC, Myung D. Bio-orthogonally crosslinked hyaluronate-collagen hydrogel for suture-free corneal defect repair. Biomaterials. 2020;255:120176. doi:10.1016/j.biomaterials.2020.120176.
- Xue Q, Ma L, Hu H, Li Q, Wang W, Yang H, Zhang B. 3D bioprinting as a prospective therapeutic strategy for corneal limbal epithelial stem cell deficiency. Int J Bioprint. 2023;9(3):710. doi:10.18063/ijb.710.
- Mörö A, Samanta S, Honkamäki L, Rangasami VK, Puistola P, Kauppila M, Narkilahti S, Miettinen S, Oommen O, Skottman H. Hyaluronic acid based next generation bioink for 3D bioprinting of human stem cell derived corneal stromal model with innervation. Biofabrication. 2022;15(1):015020. doi:10.1088/1758-5090/acab34.
- Ludwig PE, Huff TJ, Zuniga JM. The potential role of bioengineering and three-dimensional printing in curing global corneal blindness. J Tissue Eng. 2018;9:2041731418769863. doi:10.1177/2041731418769863.
- Ortega Í, Deshpande P, Gill AA, MacNeil S, Claeyssens F. Development of a microfabricated artificial limbus with micropockets for cell delivery to the cornea. Biofabrication. 2013;5(2):025008. doi:10.1088/1758-5082/5/2/025008.