Abstract
The charge distribution of airborne MS2 bacteriophage nanoparticles and the efficiency of electrical-mobility–based capture mechanisms with bipolar charging were studied. MS2 virions form large agglomerated particles in a suspension. The average charge on airborne MS2 virions can be as high as one unit charge (negatively charged). The application of both soft X-ray irradiation and alpha rays from a Po-210 bipolar charger was shown to not only reduce the average charge on MS2 virion particles but also partially fragment the larger MS2 virion agglomerates, thereby increasing the number of ultrafine MS2 virion particles. A cylindrical electrostatic precipitator with a mounted soft X-ray emitter was used to determine the effectiveness of electrical capture methods for virus particles. At low applied voltages, it was found that the capture efficiency of ultrafine virus particles can be increased by applying in situ soft X-ray irradiation with electrostatic precipitation. It has also been shown that in the presence of both a positive and negative corona, virus particles are readily captured with log removal values exceeding 4. The unit developed and demonstrated in this work is a compact, low-pressure drop system that can be readily mounted in ventilation ducts or air supply systems to remove ultrafine particles such as viruses.
INTRODUCTION
There is a renewed interest in the removal of bioaerosols and bioagents from air streams (CitationEPRI 2002). Bioaerosols could be problematic in indoor and enclosed environments such as hospitals, office buildings, and aircraft cabins, and thus there is a need to design devices that effectively remove such particles. The use of conventional filtration is quite prevalent; however, such systems are plagued with problems of high-pressure drop, high maintenance, and concern of growth and release of byproducts from biological particles. Electrical-mobility–based capture systems offer a method to overcome these limitations.
Electrostatic precipitators (ESPs) are commonly used to control fine particle emissions in industry and in several indoor air cleaning systems. ESPs are highly efficient, with overall mass-based capture efficiencies of approximately 99%. However, due to the decrease in particle charge with decrease in size, and increase in the drag forces with increase in size, ESPs typically have a collection efficiency minimum in the submicrometer-size region (CitationFlagan and Seinfeld 1988). Though not predicted by conventional ESP models, the capture efficiency has also been found to decrease significantly as particle size decreases in the ultrafine particle size region (CitationWatanabe et al. 1995; CitationKim and Biswas 1996; CitationYoo et al. 1997; CitationZhuang et al. 2000; CitationKulkarni et al. 2002). This decrease has been attributed to partial charging of ultrafine particles (CitationZhuang et al. 2000). Researchers have attempted to enhance charging of ultrafine and nanometer-sized particles by using photoelectric processes (CitationBurtscher et al. 1982; CitationJung et al. 1988). Biswas and coworkers (CitationNamiki et al. 2002; CitationKulkarni et al. 2002) were the first to show the enhanced charging of nanoparticles in unipolar coronas by the use of soft X-ray irradiation. A cascading effect in the generation of ions was attributed to the improvement in charging of nanoparticles, thus enhancing their capture in electrostatic precipitators.
Studies have shown that soft X-ray irradiation can be successfully used as bipolar and unipolar charger (CitationHan et al. 2003); however, it is the interaction with a corona that enhances the charging efficiencies of nanoparticles (CitationKulkarni et al. 2002).
Airborne virus particles, or virions, are typically in the 20–400 nm size range, and are a good example of nanoparticle bioaerosols. Smaller viruses typically contain one subunit, which consists of an outer protein capsid, internal nucleic acid, and other internal proteins (CitationMadigan et al. 1997). Airborne virus particles have been studied as a means of developing a calibration curve for estimating the molecular masses of large organic complexes (CitationBacher et al. 2001) and to develop an integrated virus detection system (IVDS) for estimating virus particle concentrations and virus particle agglomeration in liquid systems (CitationWick and McCubbin 1999a).
While virus capsid agglomeration has been documented in several studies (CitationThomas et al. 1998; CitationWick and McCubbin 1999a), previous studies of airborne virus particles have employed the use of electrospray to allow for only single-virus particle aerosolization (CitationBacher et al. 2001; CitationWick and McCubbin 1999a). Because of their small size, virus particles have a high deposition efficiency within the alveoli of mammalian respiratory systems and can lead to a variety of medical illnesses. It is necessary to develop efficient capture mechanisms for virus particles in the next generation of particle control devices. It has been observed that virus particles will pass through low molecular weight cutoff filters despite having molecular weights significantly larger than the filter cutoff weight (CitationWick and McCubbin 1999b). Because virus particles are naturally charged, electrical-field–based methods offer a potential route for their capture. A soft X-ray–enhanced corona system (CitationKulkarni et al. 2002) that was demonstrated to be effective for nanoparticle capture could also be potentially used as a low-pressure device for virus particle capture. Furthermore, due to the highly oxidizing environment (CitationNamiki et al. 2004), the trapped biological particles could also be completely inactivated.
To design effective methods for virus particle capture, there is a need to develop a fundamental understanding of their transport characteristics. The charge distribution of airborne viri is an important parameter, especially if electrical-field–based systems are to be designed for their capture. As virus particles tend to aggregate in natural systems, the characteristics of virus particle agglomerates need to be studied. The effects of bipolar charging on airborne viri must also be studied to determine if recently developed charging mechanisms for ultrafine inorganic particles are suitable for ultrafine biological particles. To the best of our knowledge, this is the first-ever study of the capture of airborne virus particles and measurement of their electrical properties. Here, data concerning the nascent charge distribution of virus particles, virus particle agglomeration, fragmentation by alpha rays and soft X-ray irradiation, and particle capture in a soft X-ray–enhanced corona generation system are presented and interpreted. Using electrostatic precipitation and bipolar chargers, the electrical properties of MS2 bacteriophage, a surrogate of the poliovirus, are established.
EXPERIMENTAL METHODS AND PLAN
The overall objective was to characterize the charge distribution of airborne virus particles and determine the effectiveness of electrical-mobility–based methods for their capture. summarizes the tests that were conducted in this study.
TABLE 1 Summary of experiments performed
ESP with Mounted X-Ray Emitter
A cylindrical ESP, shown in , was constructed with a mounted soft X-ray emitter (Hamamatsu Photonics Ltd., Japan, Model L6941). The ESP had a stainless steel outer collecting electrode 15 cm in length and 5 cm in diameter. To prevent X-ray leakage, the collecting electrode was enclosed by a poly vinyl chloride (PVC) tube. The discharge electrode, also made of stainless steel, was 0.323 mm in diameter and was insulated on the ends to prevent premature corona formation. A 2 cm circular hole was drilled through the middle of the collecting electrode to allow for soft X-ray irradiation inside the ESP. X-ray irradiation (3.5–9.5 keV, λ = 0.12–0.41 nm) spread inside the ESP at an angle of approximately 120°. To prevent particles from depositing on the X-ray emitter and to keep the system airtight, a thin polyamide film (KaptonTM 30HN, DuPont Corp., 30 μ m thick) was placed in front of the X-ray emitter. It was estimated that 90% of the soft X-rays penetrated through the polyamide film (CitationKulkarni et al. 2002). A potential difference across the discharge and collecting electrodes was formed by applying a DC voltage with a high voltage power supply (Glassman High Voltage Inc., EL series). The voltage-current characteristics were measured using a DC microammeter with approximately 10 lpm of air flowing through the ESP.
Size Distribution of MS2 Bacteriophage Particles and Large Agglomerate Measurement
MS2 bacteriophage is an RNA virus that infects male Escherichia coli. A complete MS2 virion has an outer protein capsid consisting of 180 copies of a coat protein with 129 amino acid residues (23220 amino acid residues in total). Inside the capsid are a protein molecule (the “A” protein) and an RNA molecule. MS2 is an excellent model for any virus that has an outer protein capsid and contains no lipid component, as most protein capsids will respond to electrical forces in the same fashion. Like many smaller viri, an MS2 virion has a near-spherical, icosahedral geometry (triangulation number = 3), with an approximate diameter of 27.5 nm (CitationGolmohammadi et al. 1993).
MS2 Bacteriophage is almost identical in size to the human poliovirus.
Freeze-dried MS2 virions (ATCC® 11303-B2) were suspended in ultrafiltered deionized water. The suspension contained 0.0745 g/L of MS2 virion particles.
A schematic diagram of the system used to measure the particle size distribution is shown in . MS2 virion aerosols were generated by placing the suspension in a glass Collison nebulizer or a stainless steel atomizer and drying the droplets produced with a diffusion dryer. The flow rate through the system was 10 lpm with the nebulizer and 4 lpm with the atomizer. The virus particles were then introduced into the electrostatic precipitator with a mounted X-ray emitter, either directly or through a Po-210 bipolar charger (neutralizer). Measurements were made under the following experimental conditions: (1) a Collison nebulizer without neutralizer, (2) stainless steel atomizer without neutralizer, (3) nebulizer with neutralizer, (4) nebulizer with soft X-ray, and (5) nebulizer with neutralizer and soft X-ray ().
FIG. 1b (b) Experimental setup used to measure size distribution, charge, and capture efficiency of MS2 Virion particles in a soft X-ray enhanced electrostatic precipitator. FCE, Faraday Cup Electrometer.
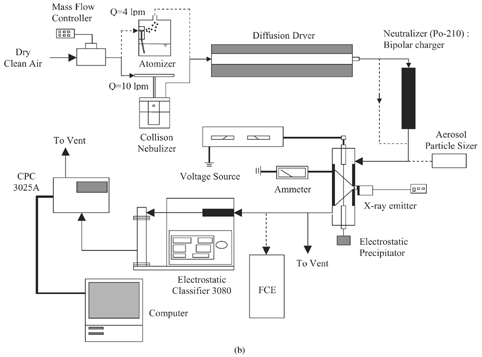
After exiting the ESP outlet, particle size distributions were measured using a Scanning Mobility Particle Sizer (SMPS) system (electrostatic classifier (EC) 3080, differential mobility analyzer (DMA) 3081, and condensation particle counter (CPC) 3025A; TSI Inc., St. Paul, MN, USA) with the CPC operating in the high flow (1.5 lpm) mode. The system measured concentrations of particles in the size range of 5–225 nm. In the SMPS system, an impactor removes the larger particles (greater than 600 nm). The flow then enters a Kr-85 neutralizer, where the particles attain a Boltzmann charge distribution. The effectiveness of the Kr-85 neutralizer for the measurements performed was verified by measuring the total charge of the aerosol after and was found to be close to 0 (result of bipolar neutralization). It must be noted that in the five experimental conditions described above, the addition and removal of a neutralizer to and from the experimental system refers to a Po-210 bipolar charger that is not part of the SMPS system (see ). The measured distribution for experimental condition (Set 2, ) was used as a baseline size distribution for the measurement of capture efficiency of MS2 particles in the ESP. To check for the presence of large agglomerated MS2 virion particles, an aerodynamic particle sizer (APS) 3320 was used to measure particles with aerodynamic diameters in the 0.3–20 μ m range. Measurements of agglomerate concentrations were made after the particles left the diffusion dryer outlet and after the outlet of the Po-210 bipolar charger (neutralizer) using both the Collison nebulizer and the stainless steel atomizer.
To examine the fragmentation of airborne particle agglomerates, total particle concentrations were measured at the inlet and outlet of the Po-210 bipolar charger with and without an impactor in the system. With a flow rate of 10 lpm, the impactor cut-point diameter was 600 nm. Total concentrations were measured using a CPC 3022A. Results were compared to total concentrations at the bipolar charger inlet and outlet without the use of the impactor.
An electrical field of 5 kV was used to deposit the particles gently onto carbon-coated electron microscope grids for viewing in a scanning electron microscope (SEM; Hitachi S-4500).
Measurement of Electric Charge on MS2 Bacteriophage Particles
The experimental setup used to measure the average charge on each MS2 bacteriophage particle included a Faraday Cup electrometer (FCE; to measure the current on collected particles) and a CPC 3022A. The flow rate through the system was 10.3 lpm, with 10 lpm going to the electrometer and 0.3 lpm going to the CPC 3022A (low flow mode). The average number of unit charges on MS2 bacteriophage particles was calculated by
Concentration-mobility and current-mobility plots were recorded. After nebulization and drying, a flow stream of particles of a single electrical mobility was produced by sending particle flow through a DMA 3081. Electrical Mobility is based on the equation (CitationHinds 1999):
Capture Efficiency of MS2 Bacteriophage Particles by Electrostatic Precipitation
After measuring the MS2 size distribution with 0 kV applied in the ESP, size distributions were measured at the ESP outlet with positive applied potentials of 3 kV, 6 kV, 8 kV, 9 kV, and 10 kV across the discharge and collecting electrodes. The measurement method was similar to that used by CitationKulkarni et al. (2002). Size distribution measurements were made with and without the in situ application of soft X-rays. Capture efficiency was calculated based on the following equation:
RESULTS AND DISCUSSION
V-I Characteristics of the Electrostatic Precipitator
shows the corona current generated in the electrostatic precipitator as a function of the applied voltage. For both positive and negative applied voltages, the corona inception voltage was 8.8 kV. The presence of a corona current indicates the production of excess positive ions when a positive potential is applied, and excess negative ions when a negative potential is applied (CitationFlagan and Seinfeld 1988). X-ray irradiation has been shown to produce bipolar ions by the photoionization of gas molecules (CitationKulkarni et al. 2002; CitationHan et al. 2003). With soft X-ray irradiation present within the ESP, the corona inception voltage dropped to approximately 6.1 kV for both positive and negative applied potentials. Similar to results found by CitationKulkarni et al. (2002), at any given applied potential the corona current was higher in the presence of X-ray irradiation, and increasing the applied potential by a given amount with X-ray irradiation increased the measured current more than when X-ray irradiation was not present. The current measurements were found to be nearly identical for positive and negative applied voltages in this study.
Size Distribution of MS2 Virion Particles and Breakup of Agglomerates During Neutralization
The size distributions of the generated particles after passing through a diffusion dryer are shown in for a set of different conditions (Set 2, ). Two aerosolization techniques were used—an atomizer, wherein smaller droplets are generated, and a Collison type nebulizer, which produced slightly larger droplets. Both resulted in broad distributions (σ g = 1.92 and 1.64, respectively) with geometric mean diameters of 37 nm (Set 2b) and 44 nm (Set 2a) for the atomizer and nebulizer, respectively (). The broad distribution of MS2 virion particles and the higher geometric mean diameters (compared to the single-unit particle diameter of 27.5 nm) are attributed to the formation of aggregates in the water suspension due to hydrophobic interaction of the viral protein capsids (CitationThomas et al. 1998). Each droplet therefore contains multiple virus particles that remain in the agglomerated state after drying. Agglomeration of aerosolized protein molecules has been reported by several other studies (CitationNiven et al. 1994; CitationBrown and Slusser 1994) and it is no surprise that virus particles, which are enclosed by outer protein capsids, also form agglomerates. The slightly smaller mean size in the case of the atomizer relative to the nebulizer was due to the droplet sizes generated being smaller and the greater potential of breaking loose some of the aggregates. Particles smaller than the typical single unit MS2 bacteriophage diameter of 27.5 nm were caused by fragmentation of some of the viri, which could have occurred during the freeze-drying process of preparing the MS2 bacteriophage pellets, resulting in some protein capsids breaking and leaving fragments of protein and RNA. The aerosolization process could also have resulted in fragmentation of some of the particles. The total number concentration was greater in case of the nebulizer because of the higher concentration of droplets that are generated by the Collison nebulizer.
FIG. 3 MS2 particle size distribution aerosolized by (a) a Collison nebulizer without neutralizer, (b) stainless steel atomizer without neutralizer, (c) nebulizer with neutralizer, (d) nebulizer with soft X-ray, and (e) nebulizer with neutralizer and soft X-ray. The SMPS system had an impactor to remove large agglomerates prior to entry into the Kr-85 neutralizer, and a negative voltage was applied to the central DMA electrode.
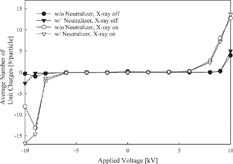
TABLE 2 Integral properties of measured size distributions under a variety of conditions (Set 2, )
An interesting observation is that the number concentration of particles in the 10 nm to 225 nm size range increased by 2.1–2.5 times (as measured by the CPC) when the aerosol was neutralized prior to introduction into the SMPS by passing through a Po-210 neutralizer or by irradiating with bipolar ions generated by the soft X-ray system (Sets 2a, 2c, 2d; ). This is attributed to the presence of aggregates larger than 225 nm that break up on charge neutralization. The APS was used to detect the presence of the larger particles in the range of 0.3–20 μ m and confirm the presence of large aggregates. The mean size of the particles as measured by the APS (in the range 0.3–20 μ m) was 2.4 μ m. The SEM pictures in confirm the presence of aggregates both in the water suspension and after nebulization. On passing through a neutralizer, the concentration of large particles decreased by about 27.5%, and the corresponding increase in concentration of total particles as measured by a CPC was 22.4 ± 1.3%. An impactor was used in the flow path to remove the large agglomerated particles (cutoff size 600 nm), and the total number concentration increase was only 4.6 ± 2.5%, indicating that the larger particles are preferentially broken up on charge neutralization. The above numbers are an average of six different measurements and are statistically significant in indicating that the large agglomerates break up preferentially on neutralization. Studies have shown that bipolar charging is effective in breaking covalent bonds (CitationFisher et al. 1999; CitationSekiguchi et al. 2000, 2001), and therefore can disrupt hydrophobic interactions by acting as a source of activation energy to fragment and add or remove charge from agglomerated particles. Virus particles are typically transported on large carrier agglomerates (which do not consist entirely of virus particles), and bipolar charging may be able to fragment virus particles from large carriers. More detailed studies are currently underway to firmly understand the size-selective partial deagglomeration process during charge neutralization.
FIG. 4 SEM images of MS2 agglomerates: (a) suspensions in solution, (b) airborne virion particles after nebulization.
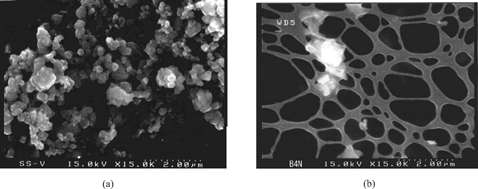
The phenomenon of aggregation and breakup has implications both in the use of electrical mobility methods for the measurement (as the instrument itself has a neutralization device) of virus particles and their capture in electrical fields. Most SMPS systems, like the one used in this study, contain an impactor that will remove large agglomerates and therefore minimize any fragmentation caused by the bipolar charge neutralizer (Kr-85) that is required for size distribution measurements. This was verified by measuring the total charge after the aerosol passed through the neutralizer, and it was close to 0. As stated earlier, when using the SMPS for size distribution measurements, the impactor was always in place to remove the larger particles, hence no bias occurred in the measured size distributions. However, caution must be exercised when reporting the size distributions of viral particles, as they would be very system specific.
Nascent Charge of MS2 Virion Particles
The average number of unit charges per MS2 particle at the exit of the diffusion dryer was determined and found to be −0.14 for the atomizer and −0.91 for the nebulizer. Nebulization produced particles with an average charge close to minus one electron, which is significantly large for particles with a geometric mean diameter of 44 nm. The Collison nebulizer was made of a glass material, which may potentially accumulate charge and transfer it to the particles as they are generated. In contrast, the atomizer had a grounded stainless steel container that probably did not result in any accumulation of charges and had lower charge transfer rates to the particles during the generation process.
Irradiation with a Po-210 bipolar charger caused a log charge reduction of approximately 3 for MS2 particles (log charge reduction is calculated as the log of the charge at Po-210 inlet divided by the charge at Po-210 outlet) with both the atomizer and the nebulizer. The bipolar chargers will remove charge from highly charged particles (in the absence of an electric field); the reduction in average charge shows that bipolar chargers are highly effective as charge neutralizers with virus aerosols. Charge neutralization was also correlated to the agglomerate particle breakup (described above). Large particles can carry higher charges than their ultrafine counterparts. However, once the large agglomerates enter a neutralizer (bipolar charger, either Po 210 or soft X-ray), the charge is removed by the energy from X-rays or alpha rays. Agglomerates are most likely held together by a liquid bridge force, which can be disrupted by soft X-ray and alpha ray irradiation. Once fragmented, the smaller particles have a lower probability of gaining and retaining charge (CitationZhuang et al. 2000), thus bipolar chargers are more effective at reducing the MS2 virion charge than would be expected if fragmentation did not occur. While a firm reason for this cannot be elucidated in this study, the observations of agglomerate breakup and charge reduction are consistent.
As the neutralization process had an effect on the agglomerate break up, a DMA without the neutralizer was used to select particles by electrical mobility (with both applied positive and negative voltage). The particles were generated by the nebulizer and dried and classified; in another experiment they were passed through an impactor (cutoff size of 600 nm). The number concentration of these mobility-classified particles was determined by a CPC, and they were passed through a FCE to determine the resulting electrometer current. The results are plotted in . Though the particles have a net negative charge, there is a broad distribution as both positive and negative mobilities were observed. Multiplying the average charge at each electrical mobility (calculated by EquationEquation (1) using the currents from ) and the corresponding concentration of particles at that mobility (from ) then summing over all mobilities gives the total charge concentration. This value was −4.75 × 109 unit electron charges/m3 when no impactor was in place, consistent with the average charge of −0.91 reported earlier. With the impactor in place, there is an excess positive charge of 1.82 × 1010 unit electron charges/m3. This clearly shows that the net negative charge on MS2 particles comes from highly negative charge on larger MS2 agglomerates.
Charge of Virus Particles at Outlet of ESP with Corona and Soft X-Ray Irradiation
A series of experiments was conducted wherein the particle number concentrations and the FC electrometer current were measured at the ESP outlet for an array of positive and negative applied voltages (Set 7, ). The estimated average particle charge (using EquationEquation (1)) at the ESP outlet is plotted in as a function of the applied voltage. Although the average charge of nebulized MS2 virion particles was −0.91 unit charges when measured at the diffusion dryer outlet, particles lost their negative charge by the time they reached the ESP outlet, even without an applied voltage or the presence of a bipolar charger. It is postulated that highly charged large agglomerates are captured in the ESP by image potential interactions with the collecting electrode of the ESP, resulting in a very low average charge. At applied voltages less than ±6 kV (no corona initiated), the average charge on the particles remains close to zero. The charged particles are indeed removed in the ESP; hence the particles leaving the ESP are uncharged. A detailed discussion of this is provided in the next section. As the applied voltage is further increased (> ±6 kV) and with soft X-ray irradiation, the particles leaving the ESP carry a net charge. The estimated average charge increases as the voltage is increased due to the effective charging in the presence of the corona, which is further enhanced by the soft X-ray irradiation (CitationKulkarni et al. 2002).
Capture Efficiencies
The capture efficiency of particles as a function of size at different conditions (Set 8, ) is shown in . The capture efficiency curves at the higher applied voltages of 9 and 10 kV have been left out of the figure as capture efficiency at these voltages exceeded 99% for all particle sizes (). When the applied voltage is below the corona inception voltage with no X-ray irradiation, the capture efficiency curves are identical for particles less than 100 nm (See graphs for 3 and 6 kV in ). This implies that a certain fraction of the particles are uncharged and they are not captured in the ESP (confirmed by the average charge of 0 measured at the ESP outlet; ). The fraction of uncharged virus particles increases as the size decreases, consistent with the charging theories of CitationFuchs (1963) and the derivations described in CitationZhuang et al. (2000). How the virus particles gain and lose charge was not examined in this work, and thus a detailed estimation of the parameters described in CitationZhuang et al. (2000) was not done. However, the low charges on the ultrafine particles are attributed to poor diffusion charging and low ion attachment coefficients (CitationAdachi et al. 1985; CitationYoo et al. 1997; CitationZhuang et al. 2000; CitationKulkarni et al. 2002).
FIG. 7 (a) Capture efficiency of different particle diameter viral particles from 10 to 225 nm for various applied voltages and soft X-ray irradiation. (b) Log capture of viral aerosols as a function of applied voltage.
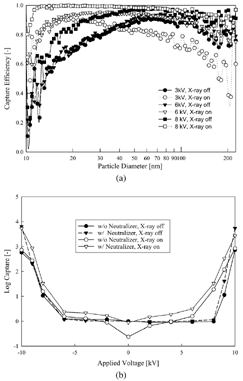
TABLE 3 Total capture efficiency (%) of particles under different conditions
A significant decrease in the fraction of uncharged particles is observed due to X-ray irradiation. The capture efficiency of the ultrafine particles increases significantly because X-ray irradiation can directly photoionize particles, enhance the electron and ion concentration in the gas space by photoionizing metal surfaces, and enhance the unipolar atmosphere creation by separating the X-ray–generated bipolar atmosphere (CitationKulkarni et al. 2002). For a 3 kV applied voltage, the capture of particles greater than 40 nm decreases on application of X-ray irradiation. This phenomenon is due to the bipolar ion generation that neutralizes some of the larger particles due to insufficient separation of the ions. Interestingly, particles smaller than 40 nm are more effectively charged when the X-ray irradiation is applied at 3 kV. This phenomenon is not observed at higher applied voltages (e.g., 6 kV), wherein ion separation is more effective, and neutralization of particles does not occur at any size, but enhanced charging is observed. To challenge the soft X-ray–corona system, the virus particles were neutralized prior to entry into the ESP. Without soft X-ray irradiation and in the absence of the corona, capture efficiencies dropped to 29.6% (). However, on irradiating with the soft X-ray, the capture efficiencies were back to about 99.5%, thus proving the effectiveness of the unit at capturing particles even if they enter the ESP uncharged.
At higher applied voltages, the capture efficiency of the particles is high, with total removal efficiencies close to 100% (). The log removal as a function of applied voltage is shown in and “4 log” removals were readily observed when X-ray irradiation was applied in the presence of a corona. Soft X-ray irradiation significantly improves the capture efficiency; however, as the applied voltage was increased, the enhanced effects of X-ray decrease because corona generation results in capture efficiencies near 100% regardless of the X-ray condition. It should be noted that lower applied voltages could be used if soft X-ray irradiation is used, and it is highly probable that soft X-ray irradiation (and alpha radiation) can be used to effectively inactivate the potential biological activity of virus particles, the subject of later work.
CONCLUSIONS
MS2 bacteriophage virions were used as a model for the study of capture of ultrafine virus particles. By hydrophobic interactions between neighboring protein capsids, virus particles tend to form large agglomerated structures, as seen by scanning electron microscopy. There is a measurable high negative charge on large agglomerated particles. Bipolar charging with soft X-ray and alpha rays successfully reduced the charge on particles, and this also resulted in partial fragmentation of agglomerates. As neutralizers, bipolar chargers have an increased effectiveness for virus particles, as they not only reduce the charge but also reduce the average particle size, which decreases the probability of a particle gaining and retaining charge.
A certain fraction of the particles was found to be uncharged and hence not removed in electrical fields. Electrostatic fields in the presence of a corona were found to remove the airborne virus particles effectively with a high collection efficiency. X-ray irradiation can create a bipolar atmosphere that can be separated by a unipolar electric field and that enhanced the charging of the ultrafine virus particles. This is consistent with the behavior of enhanced charging of inorganic particles in similar environments. X-ray irradiation significantly improved particle capture efficiency and had a greater effect on ultrafine particles at low applied voltages. However, corona generation alone was a sufficient charging mechanism for virus particles of all sizes. A potential advantage of the soft X-ray enhanced corona system is that it can inactivate the captured biological agents. Additional work along these lines will be performed to confirm the inactivation of captured viri. Further studies on charging mechanisms of these ultrafine bioaerosols, their agglomeration, and breakup also need to be conducted, and a firmer understanding of conditions that lead to a specific charge distribution on virus particles needs to be developed.
Acknowledgments
The work was partially supported by a Midwest Regional Center of Excellence (MRCE) NIH Biodefense Grant to Washington University in St. Louis. Christopher Hogan acknowledges support of a NSF-REU Grant from Washington University in St. Louis.
Notes
*Refers to DMA polarity.
a Nebulizer w/o neutralizer.
b Atomizer w/o neutralizer.
c Nebulizer w/ neutralizer.
d Nebulizer w/ X-ray.
e Nebulizer w/ neutralizer and X-ray.
REFERENCES
- Adachi , M. , Kousaka , Y. and Okuyama , K. 1985 . Unipolar and Bipolar Diffusion Charging of Ultrafine Aerosol Particles . J. Aerosol Sci. , 16 : 109 – 123 .
- Bacher , G. , Szymanski , W. W. , Kaufman , S. L. , Zollner , P. , Blaas , D. and Allmaier , G. 2001 . Charge-Reduced Nano Electrospray Ionization Combined with Differential Mobility Analysis of Peptides, Proteins, Glycoproteins, Noncovalent Protein Complexes and Viruses . J. Mass Spectrometry , 36 : 1038 – 1052 .
- Brown , A. R. and Slusser , J. G. 1994 . Propellant-Driven Aerosols of Functional Proteins as Potential Therapeutic Agents in the Respiratory Tract . Immunopharmacology , 28 ( 3 ) : 241 – 257 .
- Burtscher , H. , Scherrer , L. , Siegmann , H. C. , Schmidt-Ott , A. and Federer , B. 1982 . Probing Aerosols by Photoelectric Charging . J. Appl. Phys. , 53 ( 5 ) : 3787 – 3791 .
- EPRI . 2002 . “ Electrotechnologies for Countering Bioterrorism ” . In Technical Report 1006903 , Palo Alto, CA : Mezei and Rohr, Project Managers .
- Fisher , B. O. , Thomas , M. K. , Hatherly , P. A. , Codling , K. , Stankiewicz , M. , Karawajczyk , A. and Roper , M. 1999 . Soft X-Ray Photoionization and Fragmentation of SO2 Studied by Threshold Photoelectron-Photoion-Photoion Coincidence (TPEPIPICO) Spectroscopy . J. Phys. B , 32 ( 18 ) : 4437 – 4446 .
- Flagan , R. C. and Seinfeld , J. H. 1988 . Fundamentals of Air Pollution Engineering , Englewood Cliffs, NJ : Prentice Hall .
- Fuchs , N. A. 1963 . On the Stationery Charge Distribution on Aerosol Particles in a Bipolar Ionic Atmosphere . Geofisica Pura E Applicata , 56 : 185 – 193 .
- Golmohammadi , R. , Valegard , K. , Fridborg , K. and Liljas , L. 1993 . The Refined Structure of Bacteriophage MS2 at 2.8A resolution . J. Mole. Biol. , 234 : 620 – 639 .
- Han , B. , Shimada , M. , Choi , M. and Okuyama , K. 2003 . Unipolar Charging of Nanosized Aerosol Particles Using Soft X-ray Photionization . Aerosol Sci. Technol. , 37 : 330 – 341 .
- Hinds , W. C. 1999 . Aerosol Technology , 2nd ed. , New York : John Wiley and Sons, Inc. .
- Jung , T. , Burtscher , H. and Schmidt-Ott , A. 1988 . Multiple Charging of Ultrafine Aerosol Particles by Aerosol Photoemission (APS) . J. Aerosol Sci. , 19 : 485 – 490 .
- Kim , Y. J. and Biswas , P. 1996 . “ Controlled Studies of Submicron Particle Capture in Electrostatic Precipitators ” . In Presented at the 15th AAAR annual conference Orlando, FL October 14–18
- Kulkarni , P. , Namiki , N. , Otani , Y. and Biswas , P. 2002 . Charging of Particles in Unipolar Coronas Irradiated by in-situ Soft X-Rays: Enhancement of Capture Efficiency of Ultrafine Particles . J. Aerosol Sci. , 33 : 1279 – 1296 .
- Madigan , M. T. , Martinko , J. M. and Parker , J. 1997 . Biology of Microorganisms , 8th ed. , Upper Saddle River, NJ : Prentice Hall .
- Niven , R. W. , Ip , A. Y. , Mittelman , S. D. , Farrar , C. , Arakawa , T. and Prestrelski , S. J. 1994 . Protein Nebulization: I. Stability of Lactate Dehydrogenase and Recombinant Granulocyte-Colony Stimulating Factor to Air-Jet Nebulization . Inte. J. Pharm. , 109 ( 1 ) : 17 – 26 .
- Namiki , N. , Otani , Y. , Kulkarni , P. and Biswas , P. 2002 . “ Effective Collection of Ultrafine Particles with Soft X-Ray Irradiation under Applied Electric Field and/or Corona Discharge ” . In Proceedings of the 16th International Symposium on Contamination Control , 1 – 10 . Anaheim, CA, , USA : Institute of Environmental Sciences & Technology .
- Namiki , N. , Cho , K. and Biswas , P. 2004 . Tubular Reactor Synthesis of Doped Nanostructured Titanium Dioxide and Its Enhanced Activation by Corona and Soft X-Ray . App. Cat. B: Environmental , in review
- Sekiguchi , T. , Ikeura-Sekiguchi , H. and Baba , Y. 2000 . Site-Specific Fragmentation of Acetone Adsorbates on Si(100) in the Carbon 1s Absorption Edge . Surface Science , 454–456 : 363 – 368 .
- Sekiguchi , T. , Ikeura-Sekiguchi , H. , Imamura , M. , Matsubayashi , N. , Shimada , H. and Baba , Y. 2001 . Fragmentation and Charge-Neutralization Pathways Depending on Molecular Orientations at Surfaces . Surf. Sci. , 482–485 : 279 – 284 .
- Thomas , J. J. , Falk , B. , Fenselau , C. , Jackman , J. and Ezzell , J. 1998 . Viral Characterization by Direct Analysis of Capsid Proteins . Anal. Chem. , 70 : 3863 – 3867 .
- Watanabe , A. , Tochikobu , F. , Koizumi , Y. , Tsuchida , T. , Hautanen , J. and Kauppinen , I. E. 1995 . Submicron Particle Agglomeration by an Electrostatic Agglomerator . J. Electrostatics , 34 : 367 – 383 .
- Wick , C. H. and McCubbin , P. E. 1999a . Characterization of Purified MS2 Bacteriophage by the Physical Counting Methodology used in Integrated Virus Detection System (IVDS) . Toxicol. Methods , 9 ( 4 ) : 245 – 252 .
- Wick , C. H. and McCubbin , P. E. 1999b . Passage of MS2 Bacteriophage through Various Molecular Weight Filters . Toxicol. Methods , 9 ( 4 ) : 265 – 273 .
- Yoo , K. H. , Lee , J. S. and Oh , M. D. 1997 . Charging and Collection of Submicron Particles in Two-Stage Parallel Plate Electrostatic Precipitators . Aerosol Sci. Technol. , 27 : 308 – 323 .
- Zhuang , Y. , Kim , Y. J. , Lee , T. G. and Biswas , P. 2000 . Experimental and Theoretical Studies of Ultra-Fine Particle Behavior in Electrostatic Precipitators . J. Electrostatics , 48 : 245 – 260 .