In our ongoing efforts to achieve the high-efficiency charging of aerosol nanoparticles under low-pressure conditions, our group has recently developed an ion beam aerosol charger (IBAC) that ionizes aerosol nanoparticles using an He+ ion beam (CitationSeto et al. 2003). In earlier studies we have observed both increases and decreases in the currents from the charged particles, depending on the pressure when the polydisperse particles were irradiated by the ion beam. None of our previous studies elucidated the mechanisms of the charging itself, however. In the present paper we evaluate the charging probability of monodisperse aerosol nanoparticles using a low-pressure differential mobility analyzer (LP-DMA) and aerosol electrical condenser (AEC). The particles were negatively charged by the attachment of free electrons generated via the ionization of carrier gas by ion beam irradiation under a pressure of more than 350 Pa. A charging probability of more than 60% was obtained experimentally for the particles of 10–40 nm in mobility diameter under the pressure of 350–650 Pa. The mobility of the particles was almost the same before and after charging in a tandem LP-DMA analysis, with no multiply charged particles observed. The charging probability of nanoparticles was estimated based on the diffusion charging theory. Lastly, experiments were performed to demonstrate the performance of the IBAC in the charging of neutral particles in comparison with that of an α-ray source.
INTRODUCTION
The electrostatic mobility is a strong function of aerosol particle size, especially in the nanometer size range. For this reason, techniques and devices for the electrical classification of aerosols such as the differential mobility analyzer (DMA) are highly effective in obtaining nanoparticles within very narrow size bands (CitationKnutson and Whitby 1975; CitationWinklmayr et al. 1991) and building them up to nanostructured materials. Now that the low-pressure operation of the DMA has been realized for some years (CitationSeto et al. 1997), the low-pressure DMA (LP-DMA) can be used in conjunction with laser ablation, plasma chemical vapor deposition (PCVD), and other vapor phase material processing techniques performed under reduced pressure conditions. Our group recently applied a technique combining laser ablation with the LP-DMA technique to produce a series of electrostatically classified nanoparticles with a unique set of optical, magnetic, and catalytic properties that varied with particle size (CitationOrii et al. 2003; CitationSeto et al. 2004; CitationKohno et al. 2004). The aerosol process serves very well for the production of high-purity, highly monodispersed nanoparticles in a sequential manner, yet the low charging probability of the nanoparticles and several other factors circumscribe the production yield within a low range on the order of micrograms per hour. The diffusion charging by bipolar ions in the charge equilibrium state neutralizes most of the particles, drastically reducing the population of charged particles, especially that of nanoparticles under reduced pressure conditions (e.g., about 0.1% for 10 nm at 1000 Pa; CitationSeto et al. 1997). The high-efficiency charging of aerosol nanoparticles under a reduced pressure condition is therefore considered one of the most important prerequisites for the successful application of the aerosol process to industrial-scale material synthesis.
The objective of the present study is to develop a high-efficiency charging system for nanoparticles (2–20 nm in diameter) under reduced pressure conditions (200–1000 Pa). For this purpose, we have developed an ion beam aerosol charger (IBAC) with the following features and advantages.
1. | The apparatus generates a high concentration of positive ion via the cold cathode Penning ionization of gas. This process effectively charges aerosol nanoparticles under reduced pressure conditions with little damage and no contamination. | ||||
2. | The apparatus can be easily turned on and off, and the energy (ion concentration) can be tuned by the acceleration voltage. | ||||
3. | The particle loss is very low due to the nearly complete absence of electrostatic field generated in the aerosol stream (limited to that generated by the space charge effect). | ||||
4. | A skimmer and differential pumping system is used to create the pressure difference between the ion source (< 10 Pa) and aerosol stream (< 1330 Pa). The focused ion beam penetrates small orifice (0.3–2.0 mm in diameters) in the skimmer. |
In our first report on the ion beam aerosol charger (CitationSeto et al. 2003), we observed the change in the total electrical current from polydisperse Si nanoparticles synthesized by pulsed laser ablation. The increase of the aerosol current due to the positive ion (He+) attachment was measured under a pressure lower than 400 Pa. At pressures exceeding 400 Pa we observed unexpected increases in the negative current, probably due to the generation of secondary electrons from the neutral helium atom. However, we did not closely examine the charging mechanism or the effects of the initial charge state, particle size, or ion species. In this study we used a series of LP-DMAs to produce size-selected aerosols and an aerosol electrical condenser (AEC) to detect the number concentrations of charged aerosols with specific polarities. We also analyzed the charging probability in relation to particle size, pressure, and ion species in order to clarify the mechanisms of aerosol charging by ion beam.
CHARGING OF AEROSOL NANOPARTICLES UNDER REDUCED PRESSURE CONDITIONS
Diffusion charging becomes dominant as the particle size decreases. Upon reaching the nanoparticle (< 20 nm diam.) regime, it becomes difficult to carry more than double charges. Given that the effects of multiply charged particles are rendered negligible in this regime, unipolar ion sources become the best candidates for the high-efficiency charging of aerosol nanoparticles (CitationRomay et al. 1991). Unipolar ions are generated when gas species are ionized by irradiation of energy sources such as radioactive rays (CitationAdachi et al. 1985, Citation1987) or soft x-rays (CitationHan et al. 2003) under electrostatic fields. Unipolar ions are also generated by gas discharges such as corona discharges. In the case of argon, they are positively ionized as:
The particles are positively charged by the Brownian diffusive collision with positive ions.
Other inert gases such as helium or nitrogen are often used as carrier gases, and they similarly generate positive ions. Negative ions are also thought to be generated within the system by the attachment of electrons with ppm-order gaseous impurities (G; O2, NO, H2O, etc).
The instability in the level of impurities within the system makes it difficult to control the concentration of negative ions. It can also be very difficult to generate negative ions by corona discharge in the inert gas system.
The generation rates of both positive and negative ions decrease as the pressure falls. CitationRomay et al. (1991) investigated the unipolar charging of aerosol particles under low-pressure conditions using α-ray charger. They succeeded in obtaining a charging probability of over 70% for 10–20 nm particles under a pressure of 20 kPa-air. In experiments using a low-pressure condensation particle counter (CPC) to detect the particles, they observed reasonable agreement with the numerical results calculated based on the Fuchs' charging theory. Yet the lowest pressure applied in their system was 20 kPa, which was too high to apply for the synthesis of non-agglomerated nanoparticles by laser ablation (CitationCamata et al. 2000; CitationSuzuki et al. 2001) and for the evaluation of particulate contaminants generated in the thin film preparation process by plasma CVD. Further, a radioactive source cannot be used as an ion source under pressures below 1 kPa due to the increase in the mean free path of the gas molecules (about 13 μm for He at 1kPa) and the wide range of energy rays (about 13 m for α-ray at 1 kPa of He atmosphere).
Gas discharge apparatuses such as the corona discharger and Hewitt charger have also been used as sources to provide unipolar ions, mostly under atmospheric conditions (CitationKirsch and Zagnit'ko 1990; CitationKruis and Fissan 2001). In the Hewitt charger technique, the effect of particle precipitation by the electrostatic field is eliminated by separating the ion source and aerosol stream using an electrically grounded mesh or slit. The generated ions accelerate towards the mesh or slits, pass through, and exit within an aerosol stream. Several other gases with lower ionization potentials have also been used to increase the ion concentration and improve the mixing between the ions and aerosol. However, the discharge voltage decreases as the pressure falls (about 200 V for a 10 mm gap He atmosphere under 1000 Pa), making it impossible to achieve a stable corona discharge under low-pressure conditions.
The concepts of our ion beam aerosol charger are similar to those of the Hewitt charger. The main difference in our system is the application of higher efficiency charging using cold cathode Penning ionization of noble gas (argon or helium), a process performable under low-pressure conditions. As schematically shown in , the ion beam was generated via the focusing and acceleration by the electrostatic field up to 5 kV. When positioned about 200 mm away from the ion source, the ion probe (a) detected a beam current in the μ A range. A skimmer with a differential pumping system was installed to create a pressure difference between the ion source and aerosol pathway.
FIG. 1 Schematic illustration of the charging of aerosol nanoparticles by the ion beam. The nanoparticles were charged by the direct impact of the beam and secondary electrons generated from the surrounding He gas.
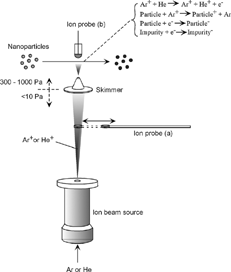
According to the measurement of the ion current by the ion probe (b) located at the aerosol stream, the current was decreased from few μ A to the order of 10 nA under a vacuum due to the beam divergence and precipitation at the skimmer. shows the ion current I i measured by the probe (b) with changing the pressure of aerosol stream. When the pressure of the aerosol stream increased, the ion current decreased due to the interaction with neutral helium atoms, ultimately reaching a negative current at pressures above 700 Pa. This behavior of the ion current in relation to the pressure suggests the occurrence of an ion-beam-induced plasma process expressed as:
Assuming that the decrease in the ion current corresponded to the generation of secondary electron, the electron current I e was obtained from the difference in the ion beam current from the initial state (I e = I i −I i0; where I i0 is ion current under the vacuum condition). The electron density, N e , can be calculated from the measured current as (CitationJauberteau et al. 2004),
FIG. 2 The pressure dependence of the ion current and electron density measured by the ion probe at the aerosol stream.
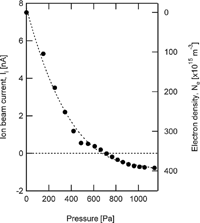
The ion beams emerging from the skimmer directly irradiated the aerosol nanoparticles, transferring their charge as:
This process is the main route for positive aerosol charging under the very low pressure conditions. On the other hand, with the increase of pressure, the ion beam might be decelerated by the interaction with surrounding gas and the particles are charged by the diffusion of positive ions as expressed by Equation (Equation2). Also the secondarily generated electrons immediately attached to the particles and imparted a negative charge due to their high diffusivity compared to the low diffusivity of the positive ions as discussed later in detail:
This process is thought to be the main route for the negative charging of aerosol with positive ion beams via the process observed in our first report (CitationSeto et al. 2003). Similar phenomena have been observed by the aerosol charging by free electron (CitationDuBard et al. 1983; CitationO'Hara et al. 1989; CitationRomay and Pui 1992), in the dusty plasma (CitationMatsoukas and Russell 1995; CitationFridman et al. 1996; CitationForsyth and Liu 2002), and in the polar mesosphere (CitationRapp and Lubken 2001).
There are also neutralization processes that take place in the presence of both positive ions and electrons,
Thus, the master equation for the population balance of q-charged particles can be expressed by the following equation when the charging of aerosol is governed by the process described as Equations (Equation2), (Equation7), (Equation8), and (Equation9).
The combination coefficient between positive ion and q-charged particle, η q +, was calculated based on the limiting sphere model (CitationFuchs 1963) using the method previously presented by CitationAdachi et al. (1985).
FIG. 3 Change in the combination coefficient for initially (a) neutral particles and (b) charged particles.
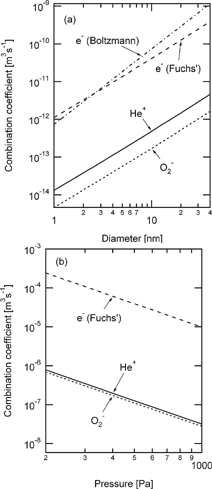
TABLE 1 Mobility of ions and electrons in He used in the calculation (100 Pa)
The combination coefficient between electron and particle is calculated from two models. The first is the application of Fuchs' limiting sphere model solely to the electron behavior by substituting electron properties (diffusion coefficient and mass) for those of ion in Equation (Equation13). This results in a combination coefficient about two orders of magnitude higher than that of the ions, as shown in and .
The second model is based on the Boltzmann distribution of electron energy in the plasma (CitationMatsoukas and Russell 1995). This model gives the following relation:
EXPERIMENTAL PROCEDURES
shows the experimental system used in this study. The details of the ion beam source and aerosol charging system have been described elsewhere (CitationSeto et al. 2003). Helium or argon positive ions generated at the ion source are accelerated by the electric field up to 5 kV and penetrate the skimmer, creating a pressure difference between the ion beam source and the charging chamber evacuated by differential pumping system. Silicon nanoparticles produced by laser ablation in the presence of helium carrier gas were used as test aerosols. The nanoparticle generation by the laser ablation system used in this study has been described elsewhere (CitationSeto et al. 2001). Having confirmed that the initial particle charging takes place in the laser-induced plasma plume, we did not use any neutralizer or charger for the first step.
FIG. 4 Experimental system used in this study. (case 1) Evaluation of charging probability of monodispersed particles, (case 2) tandem LP-DMA system, and (case 3) charging of polydisperse neutral particles.
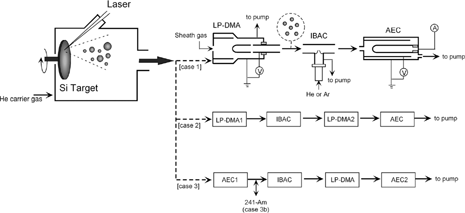
The number concentration of particles was electrically measured by an aerosol electrical condenser (AEC) combined with femtoammeter (Keithley 6514) in place of a condensation particle counter (CPC) due to the poor functioning of the latter under reduced-pressure conditions (200–1,000 Pa). The number concentration of aerosol, n, was calculated from the measured current, I, by assuming that the number of charge is unity:
Three cases of the experimental set up were used as shown in . The size-dependent charging probability of the monodispersed particles was characterized by transporting the test particles in He carrier gas and classifying them by an LP-DMA (Case 1 in ). In this case, the flow rates of carrier gas and sheath gas of the LP-DMA were 0.4 and 2.0 SLM (standard litter per minutes), respectively. The change of the charging probability was evaluated as functions of the mobility diameter of the aerosol, the polarity of the charge, the acceleration energy of the ion beam, and the pressure of the background gas.
Two LP-DMAs in series (tandem LP-DMA; case 2 in ) were used to measure the mobility of the aerosol after the ion beam charging. We should note that tandem LP-DMA experiments had never been attempted before, and it was very difficult to operate two DMAs with matching aerosol and sheath gas flow rates. By a careful tuning of pumping capacity and the conductance of the flow path, we succeeded in tandem LP-DMA operation under just one condition (p = 530 Pa).
Aerosol measurements in realistic situations generally call for an evaluation of the charging characteristics of neutral particles. The test aerosols generated by laser ablation contained particles initially charged in the laser-induced plasma plume. Therefore, as shown in the case 3 in , we precipitated most of the originally charged particles using the AEC (AEC1) as an electrostatic precipitator before the IBAC. The neutral particles were irradiated by the ion beam and the mobility spectra were measured using LP-DMA and AEC (AEC2). A radioactive source (241-Am α -ray source) was placed in the aerosol stream in some of the experiments for comparison (case 3b).
RESULTS
Ion Beam Charging of Monodispersed Aerosol
The AEC was used to measure the change in the aerosol electrical current induced by the ion beam irradiation of the monodispersed nanoparticles (case 1 in ) while applying different acceleration energy for the ion beam. shows the change in the AEC current for the Si nanoparticles with a mobility diameter of 19 nm classified by the DMA. The pressure of the system was set at 600 Pa. The AEC was operated both in the positive and negative mode. A decrease in the positive current and an increase in the negative current were observed. We were also intrigued to note that the total current (=|I +|+|I −|) seemed conserved. Though the neutral particles remained undetected under AEC observation, this phenomenon indicated a switching of the positive charges on the particles to negative charges, a process that might have stemmed from the large difference in the combination coefficients of He+ ions and electrons with particles. The increase in the acceleration energy might have increased the electron density. As a result, the percentage of the negatively charged nanoparticles penetrating the plasma region was almost proportional to the acceleration energy.
FIG. 5 Change in the current of monodispersed particles with a mobility diameter of 19 nm against acceleration energy. The AEC was operated under both positive and negative modes.
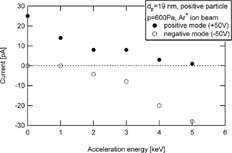
We also measured the size-dependent charging probability of the IBAC by scanning the applied voltage to the LP-DMA, as shown in . In this case, the acceleration energy was constant at 5keV. The measured particle currents were deviated at each measurement due to the charge up of the system and the instability of particles concentration generated by laser ablation. When the pressure of the system was 600 Pa, the particles with an initial positive charge exhibited a peak mobility diameter of around 17 nm (see ). A significant decrease in the positive current and an increase in the negative current were observed when the IBAC was turned on. The dotted lines indicate an almost zero current when the AEC was operated under opposite polarity (negative mode at IBAC off and positive mode at IBAC on). We thus confirm that the particles were negatively charged and tentatively attribute this condition to the electron attachment in the plasma via the process expressed in Equations (7) and (9). Only a slight decrease of the negative current was observed when the negatively charged particles were introduced into the IBAC () and the IBAC was turned on. Though unable to measure diameters larger than 25 nm due to the discharging inside the LP-DMA column under the pressure of 600 Pa, we obtained a charging probability (= n on −/n off +) of more than 60% among smaller-diameter particles in the range from 10–20 nm. We also confirmed that the particle losses in the IBAC were not significant since the size distribution of the negatively charged particles was left unchanged by the ion beam irradiation, as shown in .
FIG. 6 Size distribution of Si nanoparticles measured by LP-DMA and AEC. Change in the current by ion beam irradiation after the classification. Initially (a) positive and (b) negative particles were charged by the IBAC, respectively.
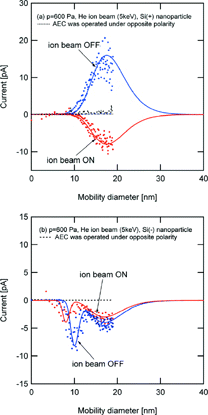
The pressure dependence of the change in the AEC current was evaluated using initially positive particles. Due to the instability of the particle generation by laser ablation and electrical charge-up in the system, we could obtain stable particle counts for the particles of less than 20 nm in diameter only at 600 Pa as previously shown in . The change of the charging probability (= n on − < eqid15 > n off +) and the retaining probability (= n on +/n off +) was measured by changing the pressure of the system. Sixty to 80% of the initially positive particles switched over to a negative polarity at pressures above 350 Pa, regardless of the particle size (20–40 nm; ), while 5–20% of the initially positive particles retained their positive polarity (). In the case of the initially negative particles, no change in the electric current was observed under our experimental conditions (250–660 Pa, 20–40 nm).
Mobility Analysis Using Tandem LP-DMA
shows the mobility spectrum of monodispersed nanoparticles measured by the second LP-DMA. As mentioned earlier, the aerosol and sheath flow rates for a pair of LP-DMAs are very difficult to match in a tandem LP-DMA configuration. We successfully adjusted the aerosol : sheath gas flow ratio to 0.4:1.6 SLM (standard litter per minutes) for both LP-DMAs at the pressure of 530 Pa by carefully tuning the conductance and pressure. The initial mobilities were set at 5 × 10−6 and 3 × 10− 6 m2/Vs (10 and 15 nm, respectively) by changing the voltage applied to the first LP-DMA. As seen in , the narrow bands of particles were successfully measured by the second LP-DMA. The measurements of the negative current indicated the same mobility peaks when the IBAC was turned on and off. Peaks of multiply charged particles were undetectable after the ion beam irradiation, indicating that most of the particles were singly charged. The absolute height of the mobility peak fell to 10% of the height of the initial peak of the positive particles, a value somewhat lower than that obtained in the the measurement for monodispersed particles under the pressure of 600 Pa (60–80% in ). More precise control of pressure and flow rates will be necessary for a detailed analysis of the pressure dependence of the charging efficiencies. A slight difference in the peak mobility was observed when Ar+ was used as the ion species (), but the peak did not reach to the mobility of doubly charged particles (it might fall in the range of experimental error). Almost the same mobility of peaks can be observed in . It should also be noted that the discharge was somewhat more stable in the ion source when Ar was used as the ion species.
DISCUSSION
Calculation of Charging Probability
The absence of multiply charged particles in the tandem DMA experiments indicated that the particles were only singly charged. In the steady state condition, the ratio of charged particles can be obtained from the ratio of combination coefficients from Eqauations (10) and (11), as follows (CitationAdachi et al. 1985; CitationSeto et al. 1997):
FIG. 9 Size dependency of the calculated charging probability against particle diameter. The properties of (a) He+ and O2 ions and (b) He+ and electron were used in the calculation.
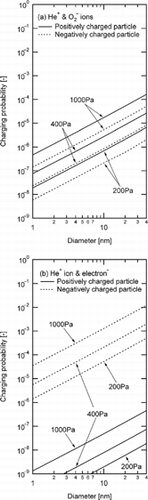
Other factors might also have played a part, however, including the space distribution of free electrons and positive ions. Given that the diffusivity of the electron was much larger than that of the positive ions, the latter might have been localized near the skimmer without mixing with the aerosol. Due to the limitation of pumping capacity, the aerosol stream was 10 mm in diameter against the skimmer diameter of 2 mm. As a result, the unipolar charging of aerosol by the free electrons might take place depending on the aerosol pathway. This phenomenon might be confirmable from the experimental result demonstrating the survival of 5–10% of the positive particles after IBAC operations (). Another factor might have been the limited utility of the diffusion charging theory in predicting the interaction between electrons and nanoparticles under low-pressure conditions. New experimental tools will be required to measure the total number concentration of neutral aerosol (unknown parameter in the present study). One option would be a new type of low-pressure CPC applicable to our pressure range (200–1000 Pa), a device to be used in one of our next studies.
Charging of Initially Neutral Polydisperse Particles
The charging probability of the initially neutral particles was evaluated as an application of the IBAC to aerosol-charging instruments under low-pressure conditions. Since the particles were initially charged during the laser ablation process, all of the charged particles were precipitated by the first AEC, as shown in case 3 in . shows the change in the size distribution of silicon nanoparticles under the pressure of 600 Pa. In the initial distribution (without precipitation by AEC1), the peak current of 30 pA was measured from 20 nm-diameter particles. No currents from the particles were detected when AEC1 was subsequently operated. When the IBAC was turned on, the new size distribution was created with a peak diameter close to the diameter of the original peak (around 20 nm). Quite remarkably, we observed an apparent increase in the current carrying particles of less than 10 nm in diameter. Given that no measurable current was observed when 241-Am was installed within the system, the α-ray source was rendered unusable as a charger under the reduced pressure conditions (around 600 Pa). Notwithstanding the rather high number concentration of the particles (on the order of 106 particles/cc even after the classification) and our inability to determine the total number concentration of aerosol, the IBAC was found to be applicable to the negative charging of the initially neutral aerosol.
CONCLUSIONS
This study has been the first to evaluate the performance of an ion beam aerosol charger (IBAC) in the charging of silicon nanoparticles under low-pressure conditions using low-pressure DMAs and an aerosol electrical condenser (AEC). The charging probability more than 60% was obtained experimentally for the particles of 10–40 nm in mobility diameter under the pressure of 350–650 Pa. The analytical prediction using the diffusion charging theory underestimated the charging probability, yet the generation of free electron by the ion beam-induced ionization of carrier gas was nonetheless found to play an important role in the negative charging of the aerosol nanoparticles. Through the successful application of a tandem LP-DMA device the mobility of the particles (10 and 15 nm, at 530 Pa) remained unchanged during the charging by IBAC and no multiple charged particles were detected. Finally, the performance of the IBAC as a unipolar aerosol charger was finally demonstrated for the charging of initially neutral polydisperse particles.
Acknowledgments
The authors would like to thank to Prof. Motoaki Adachi at Osaka Prefecture University for his valuable discussions. This work was supported by the Industrial Technology Research Grant Program in 2002 from NEDO
REFERENCES
- Adachi , M. , Kousaka , Y. and Okuyama , K. 1985 . Unipolar and Bipolar Diffusion Charging of Ultrafine Aerosol Particles . J. Aerosol Sci. , 16 : 109 – 123 . [CSA]
- Adachi , M. , Okuyama , K. , Kousaka , Y. , Kozuru , H. and Pui , D. Y. H. 1987 . Diffusion Charging of Ultrafine Aerosol Particles by Positive Helium, Argon, and Nitrogen Ions . J. Appl. Phys. , 62 : 3050 – 3052 . [CROSSREF]
- Camata , R. P. , Hirasawa , M. , Okuyama , K. and Takeuchi , K. 2000 . Observation of Aerosol Formation during Laser Ablation Using a Low-pressure Differential Mobility Analyzer . J. Aerosol Sci. , 31 : 391 – 401 . [CROSSREF]
- DuBard , J. L. , McDonald , J. R. and Sparks , L. E. 1983 . First Measurements of Aerosol Particle Charging by Free Electrons—a Preliminary Report . J. Aerosol Sci. , 14 : 5 – 10 . [CROSSREF]
- Forsyth , B. R. and Liu , B. Y. H. 2002 . Exhaust Aerosol of a Plasma Enhanced CVD System: II. Electrical Charging and Transport . Aerosol Sci. Technol. , 36 : 526 – 535 . [CROSSREF]
- Fridman , A. A. , Boufendi , L. , Hbid , T. , Potapkin , B. V. and Bouchoule , A. 1996 . Dusty Plasma Formation: Physics and Critical Phenomena. Theoretical Approach . J. Appl. Phys. , 79 : 1303 – 1314 . [CROSSREF]
- Fuchs , N. A. 1963 . On the Stationary Charge Distribution on Aerosol Particles in a Bipolar Ionic Atmosphere . Geofis. Pura Appl. , 56 : 185 – 193 . [CROSSREF]
- Han , B. , Shimada , M. , Choi , M. and Okuyama , K. 2003 . Unipolar Charging of Nanosized Aerosol Particles Using Soft X-ray Photoionization . Aerosol Sci. Technol. , 37 : 330 – 341 . [CROSSREF]
- Jauberteau , J. L. , Jauberteau , I. and Aubreton , J. 2004 . Electrostatic Probe Measurements in an Expanding Microwave Discharge Sustained in an Ar-N2-H2 Gas Mixture: Investigation on Plasma Parameters . J. Phys. D. , 37 : 1241 – 1247 . [CROSSREF]
- Kirsch , A. A. and Zagnit'ko , A. V. 1990 . Field Charging of Fine Aerosol Particles by Unipolar Ions . Aerosol Sci. Technol. , 12 : 465 – 470 . [CSA]
- Kruis , F. E. and Fissan , H. 2001 . Nanoparticle Charging in a Twin Hewitt Charger . J. Nanoparticle Res. , 3 : 39 – 50 . [CROSSREF]
- Knutson , E. O. and Whitby , K. T. 1975 . Aerosol Classification by Electric Mobility: Apparatus, Theory, and Applications . J. Aerosol Sci. , 6 : 443 – 451 . [CROSSREF]
- Kohno , M. , Orii , T. , Hirasawa , M. , Seto , T. , Murakami , Y. , Chiashi , S. , Miyauchi , Y. and Maruyama , S. 2004 . Growth of Single-Walled Carbon Nanotubes from Size-Selected Catalytic Metal Particles . Appl. Phys. A , 79 : 787 – 790 . [CROSSREF]
- Matsoukas , T. and Russell , M. 1995 . Particle Charging in Low-Pressure Plasmas . J. Appl. Phys. , 77 : 4285 – 4292 . [CROSSREF]
- O'Hara , D. B. , Clements , J. S. , Finney , W. C. and Davis , R. H. 1989 . Aerosol Particle Charging by Free Electrons . J. Aerosol Sci. , 20 : 313 – 330 . [CROSSREF] [CSA]
- Orii , T. , Hirasawa , M. and Seto , T. 2003 . Tunable Narrow-Band Light Emission from Size-Selected Si Nanoparticles Produced by Pulsed Laser Ablation . Appl. Phys. Lett. , 85 : 3395 – 3397 . [CROSSREF]
- Rapp , M. and Lubken , F. J. 2001 . Modelling of Particle Charging in the Polar Summer Mesosphere: Part 1-General Results . J. Atom. Solar-Terr. Phys. , 63 : 759 – 770 . [CROSSREF]
- Romay , F. J. , Pui , D. Y. H. and Adachi , M. 1991 . Unipolar Diffusion Charging of Aerosol-Particles at Low Pressure . Aerosol Sci. Technol. , 15 : 60 – 68 .
- Romay , F. J. and Pui , D. Y. H. 1992 . Free Electron Charging of Ultrafine Aerosol Particles . J. Aerosol Sci. , 23 : 679 – 692 . [CROSSREF] [CSA]
- Seto , T. , Nakamoto , T. , Okuyama , K. , Adachi , M. , Kuga , Y. and Takeuchi , K. 1997 . Size Distribution Measurement of Nanometer-Sized Aerosol Particles using DMA under Low-Pressure Conditions . J. Aerosol Sci. , 28 : 193 – 206 . [CROSSREF]
- Seto , T. , Kawakami , Y. , Suzuki , N. , Hirasawa , M. and Aya , N. 2001 . Laser Synthesis of Uniform Silicon Single Nanodots . Nano Letters , 1 : 315 – 318 . [CROSSREF]
- Seto , T. , Orii , T. , Hirasawa , M. , Aya , N. and Shimura , H. 2003 . Ion Beam Charging of Silicon Nanoparticles in Helium Background Gas—Design of the Ion Beam Aerosol Charger (IBAC) . Rev. Sci. Inst. , 74 : 3027 – 3030 . [CROSSREF]
- Seto , T. , Koga , K. , Akinaga , H. , Takano , F. , Orii , T. and Hirasawa , M. 2004 . Laser Synthesis and Magnetic Properties of Monodispersed Core-Shell Nanoparticles . Appl. Phys. A , 79 : 1165 – 1167 . [CROSSREF]
- Suzuki , N. , Makino , T. , Yamada , Y. , Yoshida , T. and Seto , T. 2001 . Monodispersed, Nonagglomerated Silicon Nanocrystallites . Appl. Phys. Lett. , 78 : 2043 – 2045 . [CROSSREF]
- Winklmayr , W. , Reischl , G. P. , Lindner , A. O. and Berner , A. 1991 . A New Electromobility Spectrometer for the Measurement of Aerosol Size Distribution in the Size Range from 1 to 1000 nm . J. Aerosol Sci. , 22 : 289 – 296 . [CROSSREF] [CSA]