To further validate a stochastic particle deposition model, three-dimensional deposition patterns predicted by that model were compared with corresponding spatial particle deposition data obtained from SPECT measurements. In the in vivo inhalation experiments, two different polydisperse aerosols with mass median aerodynamic diameters of 1.6 μ m and 6.8 μ m were inhaled by 12 test subjects, using different nebulizers. Predicted and measured deposition data were compared on three different levels: (1) total lung deposition, (2) deposition per hemispherical shell, and (3) deposition per airway generation. First, experimental and theoretical total lung deposition data showed good agreement for both the fine (65 ± 9% vs. 55 ± 21%) and the coarse aerosols (55 ± 8% vs. 46 ± 4%). Second, predicted deposition per hemispherical shell also corresponded well with the experimental data, both exhibiting small deposition fractions in the inner shells and a roughly quadratic increase in the outer shells. Third, fair agreement was observed for the deposition fractions per airway generation, both experimental data and modelling predictions exhibiting relatively small deposition fractions in central bronchial airway generations, followed by a steep increase in the peripheral respiratory airways. While the overall agreement between measured SPECT data and computed deposition fractions demonstrates that SPECT data can indeed be used for model validation, the current spatial resolution of the SPECT method allows only a limited validation of model predictions at the single airway generation level.
INTRODUCTION
Mathematical models of inhaled particle deposition are commonly based on morphometric lung models in which the branching network of the airway system is classified by airway generations (CitationYeh and Schum 1980; CitationKoblinger and Hofmann 1985; CitationWeibel 1991), i.e., particle deposition patterns throughout the bronchial and alveolar regions can be expressed in terms of average deposition fractions per airway generation. Such a high spatial resolution of the deposition pattern is often required for the interpretation of adverse health effects following exposure to airborne particles, such as the preferential occurrence of lung cancers in central bronchial airways.
Experimental in vivo determination of inhaled particle deposition in the human lungs is presently restricted to total and regional, i.e., tracheobronchial and alveolar, deposition (CitationICRP 1994). Thus, to compare theoretical predictions with measured regional and total deposition values for model validation purposes, predicted deposition fractions per generation have to be integrated over a defined range of airway generations, e.g. integration from generation 1 (trachea) to 15 refers to tracheobronchial deposition (CitationBergmann et al. 1997). However, measured total and regional deposition data are subject to experimental uncertainties, which may limit their use to validate predictions of local deposition patterns. Since total deposition in the human respiratory tract is the sum of extrathoracic and thoracic deposition, intersubject variations in nasal and oral deposition (CitationICRP 1994) will also affect deposition in lung airways. In addition, predictions of bronchial and alveolar deposition are based on morphometric and physiologic considerations, while corresponding experimental data are based on retention measurements, attributing the fast clearance phase to bronchial deposition and the slow clearance phase to alveolar deposition. However, the observation of a slow bronchial clearance fraction (CitationStahlhofen et al. 1995) has questioned the validity of this concept.
Even if total and regional deposition data were correctly measured, they provide only information about the sum of deposition fractions in successive airway generations within a given anatomical region, but not about deposition at the single airway generation level per se. Hence, different localized deposition patterns may lead to the same regional and total deposition values. While lung casts and airway models have been used to explore local deposition in upper bronchial airway generations (CitationCohen et al. 1990), corresponding in vivo data are presently still missing.
Limited information about localized in vivo particle deposition can be obtained from bolus inhalation experiments, in which aeosol boli are administered to specified volumetric depths in the lungs (CitationHeyder et al. 1988; CitationBrand et al. 1997). Total deposition in bolus experiments refers to deposition of particles in a sequence of airway generations from the trachea down to the generations corresponding to the selected volumetric front depths. By increasing the volumetric front depths in incremental steps, additional airway generations can be targeted in each step. However, the asymmetry and variability of the human branching system makes it impossible to assign a measured deposition fraction to a defined number of airway generations.
Experimental data from which some information on deposition in individual airway generations under in vivo inhalation conditions may be derived have recently become available through the application of Single Photon Emission Computer Tomography (SPECT), which produces images of the three-dimensional distributions of radiolabeled aerosols. While the visual inspection of SPECT images (two-dimensional cross-sections of the three-dimensional distributions) is commonly used for diagnostic purposes, CitationFleming et al. (1995) recently developed a methodology to quantify the measured three-dimensional distributions for comparison with predicted deposition fractions. This comparison first requires a hemispherical transformation of the right lung into a series of concentric equidistant hemispherical shells to convert the measured spatial activity distribution to deposition fractions in these hemispherical shells. Second, CitationFleming et al. (1995) outlined a method to convert the shell deposition fractions into deposition fractions per airway generation based on the morphometric lung model of CitationWeibel (1991). In order to utilize these SPECT data for model validation, particle deposition models must be able to predict three-dimensional deposition patterns.
CitationMartonen et al. (2000) developed a three-dimensional model of the spatial arrangement of the entire airway network for the analysis of SPECT images (CitationSchroeter et al. 2002). Airway diameters, lengths, branching, and gravity angles were based on published morphometric data, while the boundary of the lung was generated from magnetic resonance images (MRI) (CitationBurton et al. 2004). Our approach differs from that of CitationMartonen et al. (2000) in that individual paths are constructed for each inhaled particle within the boundary of the lung. Thus instead of visualizing the whole airway system, it is the three-dimensional deposition pattern that was visualized in the present study.
For the prediction of three-dimensional deposition patterns and their comparison with experimental SPECT data, we used our previously developed stochastic particle deposition model, which has extensively been validated by comparison with experimental data on total and regional deposition (CitationKoblinger and Hofmann 1985, Citation1990; CitationHofmann and Koblinger 1990, Citation1992; CitationBergmann et al. 1997). In addition to the calculation of generation-based deposition fractions, this model already contains information about the spatial arrangement of the airways and is thus capable of directly predicting three-dimensional deposition patterns. Preliminary results of modeling three-dimensional particle deposition patterns and their comparison with experimental in vivo SPECT data have previously been reported by CitationHofmann et al. (1997) and CitationBergmann et al. (1998). CitationBergmann and Hofmann (1999) and Sturm and Hofmann (2004) further described the graphical visualization of the spatial particle deposition distributions and their two-dimensional distributions in selected slices through the lungs.
The goal of the present study is two-fold: (1) To compare theoretical predictions of three-dimensional particle deposition patterns with the available SPECT data at three different levels: total deposition, deposition per hemispherical shell, and deposition per airway generation, and (2) to critically evaluate the applicability and limitations of SPECT data for validation of generation-based deposition models. The latter addresses the question, whether these model can be validated even at the level of single airway generations given the present spatial resolution of the SPECT technique.
STOCHASTIC SPATIAL DEPOSITION MODEL
The stochastic deposition model used in this work has been described in detail in previous publications (CitationKoblinger and Hofmann 1985, Citation1990; CitationHofmann and Koblinger 1990, Citation1992; CitationBergmann et al. 1997). This model has been successfully validated by comparison with experimental data on total (CitationHofmann and Koblinger 1992), regional, i.e., bronchial and alveolar (CitationHofmann and Koblinger 1992; CitationBergmann et al. 1997), and localized, i.e., in a group of bronchial airways (CitationHofmann et al. 1994), deposition over a wide range of particle sizes and breathing patterns. For the sake of brevity, only those salient features will be discussed here, which are relevant for the simulation of three-dimensional particle deposition patterns.
The Lagrangian random walk model is based on the morphology of the tracheobronchial tree analysed by CitationRaabe et al. (1976), supplemented by an acinar morphology supplied by CitationHaefeli-Bleuer and Weibel (1988). In order to utilize these data for modeling, rigorous statistical analyses of the published morphometric data were carried out to derive probability density functions for the distributions of diameters, lengths, branching, and gravity angles (CitationKoblinger and Hofmann 1985, Citation1990). For the simulation of the random path of an inhaled particle through the stochastic airway system, linear airway dimensions are randomly selected at each branching point from their probability density functions, considering also potential correlations among these parameters. By storing the spatial coordinates of each selected airway, each random pathway can be reconstructed in three-dimensional space, thus allowing the calculation of spatial particle deposition patterns.
While other generation-based morphometric lung models consider the lung as a sequence of straight tubes, the stochastic lung model is built up by a sequence of Y-shaped bifurcation units. Each airway bifurcation is composed of half of the parent airway and the two halves of both daughter airways, except for the first bifurcation, which includes the whole trachea. For the simulation of three-dimensional deposition patterns, the midpoint of a given bifurcation, defined as the intersection of the central axes of the parent and both daughter airways (CitationKoblinger and Hofmann 1990), has been designated as the deposition site in case of a deposition event, since analytical deposition equations provide information only on the deposition efficiency in a given airway but not on the actual site of deposition. Moreover, computational fluid dynamics simulations of particle deposition patterns in airway bifurcations have revealed that deposition of large particles is significantly enhanced at the bifurcation zone in bronchial airways (CitationBalásházy et al. 2003). The use of airway bifurcation numbers instead of airway generation numbers is, however, a mere question of bookkeeping and does not affect particle deposition calculations.
For the three-dimensional simulations, some subprograms and subroutines have been added to the main program (note: a detailed description of the computer algorithm is given in Sturm and Hofmann (2004)). One of these subprograms is responsible for the computation of three-dimensional deposition patterns. It calculates the spatial coordinates of the deposition sites of each aerosol particle entering the trachea and the fractions of deposited particles within a discrete number of hemispherical shells derived from the hemispherical transformation of the lung (CitationFleming et al. 1995). For the computation of the spatial deposition sites, the program consists of several specific subroutines: First, the spatial coordinates of each particle deposited in a randomly oriented airway tube are calculated. These “random” coordinates are converted to a Cartesian coordinate system with the trachea defining the z-direction by a specific transformation procedure using the transformation angles α and γ ().
FIG. 1 Method for the conversion of “random” spatial deposition coordinates into a Cartesian coordinate system with the trachea defining the z-direction. For this transformation, the two angles α and γ are used.
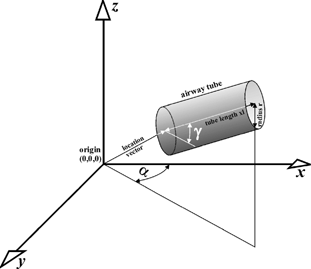
While the linear dimensions of the branching airway system are based on the stochastic lung model, information on the lung outlines and hilum positions was obtained from MRI images. Thereby, the dimensions of the two lung halves, their distance from each other, and the lengths and branching angles of the main bronchi leading to the two lungs could be adjusted to the specific lung geometry of each of the 12 subjects in the experimental study. Lengths and diameters of airways distal to the main bronchi were scaled to the specific functional residual capacity (FRC) of each subject by a constant linear scaling factor (CitationHofmann et al. 2002).
In the next step, a discrete number of individual paths (usually of the order of 105) is constructed to compute the three-dimensional distribution of airway bifurcations. At each selection of airway dimensions, the spatial positions of a given airway bifurcation are computed. Following the hemispherical transformation procedure outlined by CitationFleming et al. (1995), each airway bifurcation can be assigned to a specific shell from 1 to 10. For example, the distribution of bronchial airway bifurcations in shell number 3 and 8 is illustrated in . While the inner shell contains mostly larger bronchial airways, the outer shell comprises a wide range of bronchial air passages (note: the maximum number of bronchial airway bifurcations reported in the morphometric measurements is 21). Due to their short residual pathlengths, acinar airways usually belong to the same shell as their corresponding terminal bronchioles.
If a randomly selected airway intersects the defined lung surfaces, then an additional shell no. 11 is created and particles deposited in that shell are subsequently equally distributed among shells 9 and 10. If the randomly selected path would further continue beyond this additional shell, then it will be rejected. An alternative method to confine randomly selected paths within a given outline of the lung is to reflect the airway at the boundary surface (note: this optional selection in the code was not used in this study as it did not affect the results).
In the last step of the respective subroutine, particle deposition is calculated for randomly selected airway paths. If deposition of a particle occurs within a given bifurcation, the deposition site can directly be related to the corresponding shell, thus allowing the computation of deposition fractions per shell. Alternatively, deposition in individual generations can be computed by the standard deposition model, i.e., without storing the spatial coordinates of each deposition event, and assigned to each shell based on the relative contributions of each airway generation to a given shell (see ).
EXPERIMENTAL DATA
In a series of experiments, 12 human test subjects inhaled radiolabeled 99mTc aerosols from two different types of jet nebulizers under controlled tidal breathing conditions (CitationHashish et al. 1998). The original purpose of these experiments was to investigate the influence of particle size on the deposition pattern of clinically administered aerosols within the lung using radionuclide imaging. The purpose of the current study is to use the imaging data to test the validity of mathematical deposition models by simulating the experimental conditions. The two nebulizers studied were the Optimist (Medic-Aid Ltd, UK) and the Inspiron (Bard International Ltd, UK). They were used to produce radiolabeled aerosols of 99mTc-labelled human serum albumin. The group studied consisted of healthy non-smoking male subjects aged between 21 and 40 years, with no evidence of small airway disease. Standard lung function tests were performed on all subjects including measurements of functional residual capacity (FRC). Tests were conducted first with the Optimist and then, after one week, with the Inspiron nebulizer. This period was sufficient to allow the radioactivity from the first test to decay, before the second test was started. Subjects, seated in an upright position, at rest, and fitted with a nose clip inhaled the aerosol through a mouthpiece under otherwise natural breathing conditions. The pattern of inhalation was controlled using a closed nebulizer circuit with pneumotachographs linked to a pressure-flow transducer and displayed as a real-time pattern on a computer screen. The average respiratory parameters were as follows: tidal volume, 1081 ml (876–1533), breathing frequency, 10.8 min− 1 (8–13), inspiration time, 1.88 s (1.46–2.76), expiration time 3.65 s (2.61–4.74), and FRC, 3,757 ml (2610–4570). No significant difference between the breathing parameters used for the two nebulizers was found as verified by a paired t-test. The nebulizer circuit used valves and filters in such a way that all the activity from the device could be collected and counted in the subsequent imaging procedure. The administration was arranged to deliver approximately 20 MBq to the lungs of each subject.
The particle size distributions from the two nebulizers were measured using a laser diffraction method (Malvern Particle and Droplet Sizer) (CitationSwithenbank et al. 1977). The same apparatus was used for the human subject administration, with the particle size being measured at the mouthpiece. For both types of nebulizers, six different devices were used. The mass median aerodynamic diameters (MMAD), assuming unit density particles, were found to be 1.6 ± 0.1 μ m (1 SD) (Optimist) and 6.8 ± 0.5 μ m (1 SD) (Inspiron) and the corresponding mean geometric standard deviations, σg, 1.5 ± 0.1 and 2.4 ± 0.1 μ m (1 SD). These are subsequently referred to as the fine and the coarse aerosols, respectively.
The three-dimensional spatial distribution of inhaled radiolabeled aerosols in the lung was measured by SPECT, and corresponding anatomical information about the two lungs was obtained from magnetic resonance imaging (MRI) (CitationFleming et al. 1996). The distribution of activity was described in a matrix of cubic voxels of dimension 4.7 mm, which have a volume of 103 mm3. Planar imaging was also performed to enable the total deposition in the lungs and extrathoracic airways to be measured as a fraction of inhaled activity (CitationHashish et al. 1998).
The measured activity values in the voxels were transformed to give the deposition in each of the ten concentric shells. Each voxel was assigned to a shell based on its fractional radial distance from the hilum of the lung to the corresponding point on the lung periphery. The anatomical data for the shell transformation was obtained from the MRI images. The analysis provided a description of the average deposition concentration from the center to the periphery of the airway tree for each lung (CitationPerring et al. 1994; CitationFleming et al. 2000b). The data were then converted to deposition fractions per individual airway generation using a conceptual model of the lung, which defined the volume of each generation in each lung shell (CitationFleming et al. 1995, Citation2000a). The most recent adaption of this model (Fleming et al. 2004) is based on existing anatomical data obtained from both in vitro cast measurements (CitationWeibel 1991; CitationICRP 1994) and in vivo data from computed tomography (CT) imaging (CitationSauret et al. 2002). This method has been thoroughly validated using computer simulation (CitationFleming et al. 1997)
COMPARISON WITH EXPERIMENTAL DATA
The comparison between SPECT measurements and results of the computer simulations has been carried out on three different levels: (1) total lung deposition, which is defined here as the aerosol fraction deposited in the thoracic region, consistent with the SPECT data; (2) deposition per shell, which requires the assignment of the spatial coordinates of a simulated deposition event to a given shell; and, (3) deposition per generation, which represents the highest spatial resolution of the deposition pattern presently achievable for the whole lung. While predictions of total deposition and of the distribution among the hemispherical shells can directly be compared to the experimental SPECT data, the comparison at the airway generation level requires transformation of the measured SPECT data from the initial 3D lung activity distribution to the activity in airway generations, based on modelling assumptions.
For the deposition calculations, the measured mass-size distributions were converted to equivalent number-size distributions as the aerodynamic behavior of an inhaled particle is determined by its aerodynamic diameter, which is identical with the geometric diameter for unit density particles. Based on the assumption of lognormal distributions, the mass-size and number-size distributions are related to each other through the Hatch-Choate conversion equation (CitationHinds 1999), where the two median diameters, mass median diameter MMD and count median diameter CMD, are related through their ratio, while the geometric standard deviations are the same for both distributions.
For the comparison between the computed deposition patterns and the measured activity patterns, the deposition site of each deposited particle has to be weighted by its activity. Thus the number-size distribution of particles deposited in a given airway generation was converted back to the corresponding mass-size-distribution and then integrated to give the whole mass deposited in that generation. Assuming uniformity of concentration, each particle has the same specific activity and hence the activity-size distribution is the same as the mass-size distribution.
Total Lung Deposition
Total deposition in the SPECT measurements refers to the total activity deposited within the lungs. Thus, to be consistent with the experiment, total lung deposition in the stochastic model is defined as the fraction of particles deposited in the bronchial and pulmonary regions of the lung with the exception of the first bifurcation (i.e., trachea and the main bronchi), which is situated outside the lungs. The filtering efficiency of the oral passages was calculated by using the empirical equations proposed by CitationCheng (2003). The comparison between model predictions under defined mouth breathing conditions and the corresponding experimental data is presented in .
TABLE 1 Comparison of experimental results and theoretical predictions for fine and coarse aerosol deposition in the human lungs, expressed in percent of the total activity inhaled. Experimental data represent average values for all 12 test subjects
Given the statistical uncertainties of each approach, represented by the associated standard deviations, fair agreement was found between the experimental results and the theoretical predictions. Concerning the fine polydisperse aerosol, an average thoracic deposition of 65 ± 9% for the 12 subjects investigated was obtained from SPECT experiments, while the computed total lung deposition is 55 ± 8%. With respect to the coarse polydisperse aerosol, the average total lung deposition derived from the SPECT experiments amounts to 55 ± 21%, and the respective value from the model is 46 ± 4%. The lower value predicted by the model may be attributed to the higher deposition in the mouth, which reduces deposition in the lungs. Predicted total, i.e., thoracic and extrathoracic, deposition values are again similar to the experimental data, i.e., 60 ± 8% vs. 69 ± 9% for the fine aeorosol and 94 ± 3% vs. 86 ± 9% for the coarse aerosol.
Deposition per Shell
Based on the measured activity of radiolabeled aerosols in the lungs, CitationFleming et al. (1995) designed a hemispherical transformation method to subdivide the three-dimensional activity distribution in the right lung into 10 equidistant hemispherical shells. In contrast to the SPECT data used in our earlier analysis (CitationHofmann et al. 1997), that same data were now re-analyzed by an updated shell analysis, thereby also considering the partial volume effect. The partial volume effect results from the limited resolution of the SPECT images, which causes the position of detected gamma rays in the image to be blurred out from their position of origin. This means that the image distribution does not perfectly match the true activity distribution. Knowledge of the blurring process enables this effect to be corrected (CitationFleming et al. 2000b, Citation2001). The resulting semi-experimental shell deposition data (expressed in percent of the total lung activity), representing averages over all 12 test subjects and normalized to a total deposition in the lungs of 100%, are plotted in together with the corresponding results from the model simulations (mean ± 1 SD) for both aerosols. The standard deviations for the predicted data primarily represent the intra- and intersubject variability of the individual lung geometries and spontaneous breathing patterns. Consistent with the modified SPECT data, computed relative shell deposition (expressed in percent of the total lung activity) is similar for both fine and coarse aerosols, increasing in a roughly quadratic fashion as a function of the shell number. The observed maximum of measured and predicted deposition in the peripheral shells indicates that the alveolar region is the preferential deposition site for both nebulizer aerosols. The minor differences observed between the two approaches may well be attributed to intersubject variations of lung morphology.
FIG. 3 Deposition fractions per shell (expressed in percent of the total lung activity) for the fine and coarse aerosols derived from experimental SPECT data (EXP) and Monte Carlo modeling predictions (MC). Data are normalized to total deposition in the lungs (bronchial and alveolar regions). Associated standard deviations reflect the intra- and inter-subject variability of morphometric parameters.
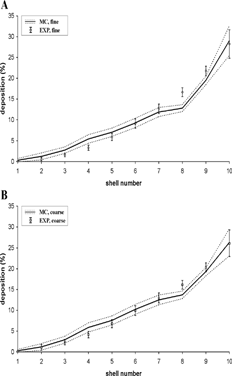
Based on the shell deposition data displayed in , deposition concentrations were obtained by dividing the relative shell deposition data by the volume of the individual shells. The computed distribution of the deposition concentration among the 10 hemispherical shells, normalized to total lung deposition, is plotted in and compared with the corresponding data derived from the SPECT measurements. While fair agreement can be observed for coarse aerosols over the whole range of shells, model predictions for the fine aerosol in the inner shells are consistently higher than the semi-experimental data. Although intersubject differences in lung morphology may play a role, this seems to be a genuine inconsistency between the results.
FIG. 4 Deposition concentrations, i.e., deposition fractions (expressed in percent of the total lung activity) in a given shell divided by the volume of that shell (in ml), for the fine and coarse aerosols derived from experimental SPECT data (EXP) and Monte Carlo modeling predictions (MC). Data are normalized to total deposition in the lungs (bronchial and alveolar regions). Associated standard deviations reflect the intra- and inter-subject variability of morphometric parameters.
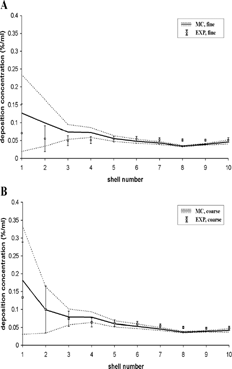
Deposition per Generation
Finally, predicted and measured deposition fractions per airway generation were compared for the fine and coarse aerosols. It must be emphasized here that the deposition fractions published by CitationFleming et al. (1995) have to be considered as semi-experimental only, as the original data were twice modified, first by the transformation to hemispherical shells and second by using CitationWeibel's (1991) updated lung model for the distribution of airways within each shell. In contrast, predicted deposition fractions for single airway generations are directly determined by the stochastic model.
Although CitationWeibel's (1991) updated lung model used for the analysis of the SPECT data consists of 23 airway generations, inhaled aerosols penetrated, on average, only to generation 21 under tidal breathing conditions. Hence, no deposition events were reported for generations 22 and 23. Likewise, deposition in the trachea was also neglected here as any deposition occurring in the trachea does not contribute to deposition in the thoracic region in the SPECT measuremements.
When comparing the deposition fractions among bronchial and acinar airway generations predicted by the stochastic asymmetric lung model with the deposition fractions derived from the SPECT measurements with the use of a deterministic symmetric lung model (CitationWeibel 1991), two fundamental problems arise. First, the stochastic lung model predicts deposition events in 27 airway generations, as compared to only 21 generations in Weibel's model (see ). This poses the problem of how to assign deposition events in generations 22–27 to the other acinar generations, as all of them contribute to deposition in the outer shells. Second, the asymmetry of division at airway bifurcations leads to a variable number of bronchial airway generations along each particle path, ranging from 12–21 (CitationKoblinger and Hofmann 1985). For example, a generation 14 airway in the stochastic lung model may be still a conductive bronchiolar airway or already an alveolated airway, while it is, by definition, a terminal bronchiole in CitationWeibel's (1991) model. In order to enable a reasonable comparison between the two approaches, the stochastic model predictions were converted to the generation structure of the SPECT data based on the following assumptions: (1) all airways in generations 22–27 of the stochastic model are acinar airways, i.e., they are partly or fully alveolated; (2) deposition in all acinar airways occurs primarily in the attached alveoli; (3) total alveolar deposition, i.e., deposition in all aveoli of the stochastic model independent of their actual generation number, are distributed among generations 15–21 of the Weibel model relative to the total number of alveoli in these generations; and (4) only deposition in conductive airways was considered for generations 12–14.
FIG. 5 Deposition fractions per generation (expressed in percent of the total lung activity) for the fine and coarse aerosols derived from experimental SPECT data (EXP) and predicted by the Monte Carlo deposition model (MC), modified to correspond to the airway structure of CitationWeibel's (1991) lung model. Data are normalized to total deposition in the lungs (bronchial and alveolar regions). Associated standard deviations reflect the intra- and inter-subject variability of morphometric parameters.
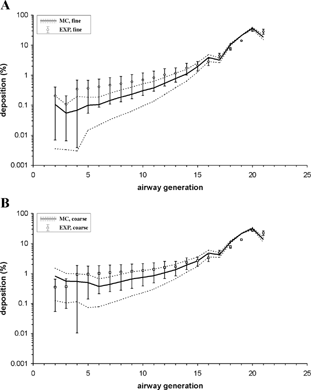
The modified stochastic model deposition patterns (expressed in percent of the total lung activity) are displayed in together with the semi-experimental curves, normalized to total deposition in the thoracic region of the human respiratory tract. As noted above, the SPECT data used in the present study are based on a new method for the shell to airway generation conversion (CitationFleming et al. 2000a, Citation2001), and thus differ from the data utilized in our earlier study (CitationHofmann et al. 1997). Deposition from airway generation 1–17 increases slightly with penetration into the lungs, while deposition fractions from generations 17–21 increase in a much steeper fashion. All curves plotted in have very similar shapes, exhibiting satisfactory overall agreement between model predictions and semi-experimental deposition data. While excellent agreement can be observed for the acinar airway generations, computed deposition fractions in generations 1–15 are consistently lower than the corresponding data derived from SPECT measurements.
To further illustrate the differences in deposition per generation between the two approaches, bronchial (integrated over generations 2–14) and acinar deposition fractions (integrated over generations 15–21), expressed as percentages of the total activity deposited in the lung, are listed in . Two distinct differences can be observed for the bronchial tree: (1) predictions of bronchial deposition fractions by the stochastic model are consistently smaller than those derived from the SPECT data, for both coarse and fine aerosols, and (2) SPECT-derived deposition fractions are the same for both aerosols, while the stochastic model correctly predicts a higher bronchial deposition for the coarse aerosol, reflecting an increased impaction efficiency for the larger aerosol. Corresponding differences for the acinar region may be attributed primarily to the above discussed filtering efficiency of the upstream bronchial tree.
TABLE 2 Relative distributions of stochastic and SPECT-derived model predictions of total lung deposition among bronchial (generations 2–14) and acinar (generations 15–21) airways, expressed in percent of the total lung activity. Experimental data represent average values for all 12 test subjects
DISCUSSION
In the present study, spatial activity distributions of radiolabeled aerosols obtained from single photon emission computer tomography have been compared with theoretical predictions of three-dimensional particle deposition generated by a stochastic particle deposition model to validate the model's predictions of deposition on a generation-by-generation basis. The optimal comparison between experiment and theory would be the direct comparison between measured spatial activity distributions and simulated three-dimensional deposition data. However, such a comparison cannot yet be realized because of the presently limited spatial resolution of the SPECT technique, so that much of the information of particle deposition in smaller regions cannot be displayed. As a consequence of this, the comparison has to be based on deposition fractions per hemispherical shell, which were converted from the original acitivity distribution by the transformation method designed by CitationFleming et al. (1995), thus no longer representing truly experimental data.
Regarding the subsequent conversion of these shell data into deposition fractions per airway generation, further mathematical transformations were applied, including morphometric data based on CitationWeibel's (1991) lung model. In contrast, deposition calculations with the stochastic lung model are based on a different lung morphology, using the morphometric data measured by CitationRaabe et al. (1976) and subsequently statistically analyzed by CitationKoblinger and Hofmann (1985). Since there are some recognizable differences between the two lung geometries in terms of numbers of airway generations, linear airway dimensions, and branching patterns, such differences may influence the present comparison. While the choice of a particular lung model does not seem to cause appreciable variations of the resulting particle deposition in the hemispherical shells, it does, however, affect the derived deposition patterns at the airway generation level. This suggests that, for the time being, the use of shell deposition data provides the most relevant comparison between the SPECT deposition measurements and particle deposition models. Because of these two transformation processes, the shell and generation deposition data derived from the original experimental SPECT data have to be considered only as “semi-experimental” evidence. In other words, the comparison is not one between experiment and theory, but rather one between two models.
The hemispherical transformation of the SPECT data, introduced by CitationFleming et al. (1995), has been applied only to the right lung, i.e., reported deposition distributions refer only to one half of the lungs, while deposition in the stochastic lung model is routinely computed for both lungs. Because of the branching asymmetry in the two lungs (3 lobes in the right lung and 2 lobes in the left lung), the question arises, whether intrapulmonary deposition is different in the two lungs. Despite the morphological differences, separate simulations for the left and the right lung produce very similar deposition patterns. Thus potential differences between semi-experimental data and model predictions do not seem to be caused by considering both lungs or only the right lung.
In the present study, comparison of particle deposition at the hemispherical shell level is based on two different representations: (1) total deposition in individual shells and (2) deposition per volume in individual shells. Concerning the deposition of aerosols in the respective hemispherical shells without normalization, experimental and theoretical distributions are very similar, with nearly negligible deposition in the inner shells and a roughly quadratic increase of deposition in the outer shells (). Because of an ever increasing volume from the innermost to the outermost shell normalization to volume results in deposition curves displaying the opposite tendency, i.e., deposition generally decreases toward the outer shells ().
Earlier comparisons between experimental data and theoretical predictions of deposition patterns among bronchial and acinar airway generations, based on the same morphometric lung model (CitationWeibel 1991), indicated that predicted deposition fractions for both fine and coarse aerosols are consistently higher than the SPECT-derived deposition fractions in most airway generations, except for the most distal airways, where the opposite tendency has been observed (CitationFleming et al. 1995, Citation1996; CitationHashish et al. 1998). Probably due to an improved analysis of the SPECT data and the application of a more realistic particle deposition model, the present study provides much better agreement between the two methods.
Although special efforts were made to adjust the stochastic lung model to the volumes and shapes of the lungs used in the experiments, intersubject variability in lung structure may still play a major role when comparing modeling predictions based on a specific lung with experimental data derived from other lungs. Indeed, SPECT data from all 12 test subjects have indicated significant intersubject variations of total and regional deposition (), which may be related to different spatial deposition patterns. Moreover, systematic differences between the two methodologies generating deposition fractions in single airway generations and hemispherical shells, e.g., by using two different lung models, may further contribute to potential differences.
Because of the relatively small number of airways in the inner shells (note: shells 1–3 refer to airway generations 1–6, see ), morphometric differences have a much more pronounced effect on particle deposition than in the bronchiolar and acinar regions, where such differences may be compensated by the large number of airways. Moreover the lengths of airways in the peripheral part of the lung are much smaller than the thickness of the shells. Inner shell uncertainties may be further enhanced by the very definition of the bifurcation midpoint as the deposition site in the model predictions is more sensitive to intersubject variations than the relatively uniform deposition within airways under in vivo conditions.
It should be noted that our modeling predictions refer to an erect position of the lung. Although the aerosol was administered in an erect position in the experiments, the SPECT and the MRI images were measured in a supine position. This effect has presently been considered by expanding the supine MRI lung shapes by an average linear expansion factor of (1.45)1/3, to correspond to the erect position in the simulations assuming that lung density does not change. This expansion, affecting volume and shape of the lungs, may add another uncertainty to the comparison of spatial deposition distributions.
In order to explore the potential effect of hygroscopic growth of inhaled particles on the spatial particle deposition pattern, which may also affect the activity-mass relationship of the inhaled aerosols, increase in particle size was simulated by assuming different growth factors ranging from 1–2 (CitationHofmann et al. 2001). While hygroscopic particle growth increased total deposition for both fine and coarse aerosols, their relative distributions throughout the lungs hardly changed. This suggests that hygroscopic growth will not appreciably affect the present comparison of spatial deposition patterns.
Mucociliary clearance in the bronchial airways may be another factor which could have modified the initial deposition patterns in the SPECT measurements by shifting the deposited activity toward the trachea, i.e., closer to the inner shells. Considering that the total time elapsed between the onset of inhalation and the end of the SPECT measurement is about 20–30 minutes, mucus velocities are only high enough in the large bronchial airways to produce an appreciable effect. Thus, the distribution of particles initially deposited within the first 4 shells may be shifted to the inside by one shell at maximum, without changing in the peripheral shells.
In addition to the limited spatial resolution of the SPECT data and the use of different lung models in both approaches, the validation method presented here is also limited by the inhalation of two relatively wide aerosol size distributions, producing similar shell distributions (). Thus the inhalation of particles with narrower size distributions, preferably being monodisperse, or the inhalation of a wide range of particle sizes, would significantly improve the applicability of SPECT data for model validation.
The standard deviations associated with the computed average values (see and , , ) are caused by the variability and asymmetry of the stochastic lung model, which also affects the transformation from the generation structure of the lung model to the shell structure, and by the individual breathing patterns. An additional uncertainty may arise from the representation of the measured size distributions by lognormal distributions. As ideal lognormal distributions are rarely found in practice, the assumed median and geometric standard deviations for the fine and coarse aerosol used for the deposition calculations must be considered as approximations. This uncertainty also affects the transformation of the activity (mass) distribution to the number distribution and back to the activity distribution, since these transformations are based on the assumption of lognormality. However, variations of the relatively wide size distributions within a reasonable range hardly affected particle deposition, so that the issue of lognormality may have only a minor impact on the uncertainty of the deposition calculations.
In conclusion, deposition data derived from SPECT images provide valuable experimental information about the differential distribution of particles in the human lungs, which can be used to validate particle deposition models. However, the spatial information from SPECT imaging is limited by its spatial resolution, which is about 15 mm, while the sampling of the distribution is performed with a voxel size of considerably less than this (4.7 mm) to avoid data loss due to digitization. This resolution means that determination of deposition in individual airways is rather limited. However, the use of conceptual models does enable average concentrations in a given generation to be estimated. Although there are limitations to the accuracy that can be achieved, quite reasonable estimates of deposition per generation could be obtained. While this method may not allow the accurate determination of deposition efficiencies in airway generations, it offers the possibility of estimating the relative distribution of deposited matter.
Acknowledgments
This research was supported in part by EU Contract No. FIGD-CT-2000-00053 (theoretical work) and by AstraZeneca (experimental work). The authors gratefully acknowledge the contributions of L. Koblinger and R. Bergmann to the initial development of the three-dimensional deposition model.
REFERENCES
- Balásházy , I. , Hofmann , W. and Heistracher , T. 2003 . Local Particle Deposition Patterns May Play a Key Role in the Development of Lung Cancer . J. Appl. Physiol. , 94 : 1719 – 1725 .
- Bergmann , R. , Hofmann , W. and Koblinger , L. 1997 . Particle Deposition Modeling in the Human Lung: Comparison between Monte Carlo and ICRP Model Predictions . J. Aerosol Sci. , 28(Suppl. 1) : S433 – S434 . [CROSSREF]
- Bergmann , R. , Hofmann , W. , Fleming , J. S. and Conway , J. H. 1998 . Simulation of Three-Dimensional Particle Deposition Patterns . J. Aerosol Sci. , 29 (Suppl. 1) 29 : S947 – S948 . [CROSSREF]
- Bergmann , R. and Hofmann , W. 1999 . Monte Carlo Simulation of Two-Dimensional SPECT Images . J. Aerosol Med. , 12 : 127
- Brand , P. , Rieger , C. , Schulz , H. , Beinert , T. and Heyder , J. 1997 . Aerosol Bolus Dispersion in Healthy Subjects . Europ. Respir. J. , 10 : 460 – 467 . [CROSSREF]
- Burton , R. T. , Isaacs , K. K. , Fleming , J. S. and Martonen , T. B. 2004 . Computer Reconstruction of a Human Lung Boundary Model from Magnetic Resonance Imaging . Respir. Care , 49 : 180 – 185 . [PUBMED] [INFOTRIEVE] [CSA]
- Cheng , Y. S. 2003 . Aerosol Deposition in the Extrathoracic Region . Aerosol Sci. Technol. , 37 : 659 – 671 . [CROSSREF]
- Cohen , B. S. , Sussmann , R. G. and Lippmann , M. 1990 . Ultrafine Particle Deposition in a Human Tracheobronchial Cast . Aerosol Sci. Technol. , 12 : 1082 – 1091 .
- Fleming , J. S. , Nassim , M. , Hashish , A. H. , Bailey , A. G. , Conway , J. , Holgate , S. , Halson , P. , Moore , E. and Martonen , T. B. 1995 . Description of Pulmonary Deposition of Radiolabeled Aerosol by Airway Generation Using a Conceptual Three-Dimensional Model of Lung Morphology . J. Aerosol Med. , 8 : 341 – 356 .
- Fleming , J. S. , Hashish , A. H. , Conway , J. H. , Nassim , M. A. , Holgate , S. T. , Halson , P. , Moore , E. , Bailey , A. G. and Martonen , T. B. 1996 . Assessment of Deposition of Inhaled Aerosol in the Respiratory Tract of Man Using Three-Dimensional Multimodality Imaging and Mathematical Modeling . J. Aerosol Med. , 9 : 317 – 327 . [PUBMED] [INFOTRIEVE] [CSA]
- Fleming , J. S. , Hashish , A. H. , Conway , J. H. , Hartley-Davies , R. , Nassim , M. A. , Guy , M. J. , Coupe , J. , Holgate , S. T. , Moore , E. A. , Bailey , A. G. and Martonen , T. B. 1997 . A Technique for Simulating Radionuclide Images from the Aerosol Deposition Pattern in the Airway Tree . J. Aerosol Med. , 10 : 199 – 212 .
- Fleming , J. S. , Conway , J. H. , Holgate , S. T. , Bailey , A. G. and Martonen , T. B. 2000a . Comparison of Methods for Deriving Aerosol Deposition by Airway Generation from Three-Dimensional Radionuclide Imaging . J. Aerosol Sci. , 31 : 1251 – 1259 . [CROSSREF]
- Fleming , J. S. , Sauret , V. , Conway , J. H. , Holgate , S. T. , Bailey , A. G. and Martonen , T. B. 2000b . Evaluation of the Accuracy and Precision of Lung Aerosol Deposition Measurement from Single-Photon Emission Computed Tomography Using Simulation . J. Aerosol Med. , 13 : 187 – 198 . [PUBMED] [INFOTRIEVE] [CSA]
- Fleming , J. S. , Conway , J. H. , Bolt , L. , Epps , B. , Bailey , A. G. , Holgate , S. T. and Martonen , T. B. 2001 . Comparison of SPECT Data on Regional Aerosol Deposition with Empirical Modelling . J. Aerosol Med. , 14 : 417 [CROSSREF]
- Haefeli-Bleuer , B. and Weibel , E. R. 1988 . Morphometry of the Human Pulmonary Acinus . Anat. Rec. , 220 : 401 – 414 . [PUBMED] [INFOTRIEVE] [CROSSREF]
- Hashish , A. H. , Fleming , J. S. , Conway , J. , Halson , P. , Moore , E. , Williams , T. V. , Bailey , A. G. , Nassim , M. and Holgate , S. T. 1998 . Lung Deposition of Particles by Airway Generation in Healthy Subjects: Three-Dimensional Radionuclide Imaging and Numerical Model Prediction . J. Aerosol Sci. , 29 : 205 – 215 . [CROSSREF] [CSA]
- Heyder , J. , Blanchard , J. D. , Feldman , H. A. and Brain , J. D. 1988 . Convective Mixing in the Human Respiratory Tract: Estimates with Aerosol Boli . J. Appl. Physiol. , 64 : 1273 – 1278 . [PUBMED] [INFOTRIEVE]
- Hinds , W. C. 1999 . Aerosol Technology—Properties, Behavior, and Measurement of Airborne Particles , p. 97 New York : John Wiley & Sons .
- Hofmann , W. and Koblinger , L. 1990 . Monte Carlo Modeling of Aerosol Deposition in Human Lungs. Part II: Deposition Fractions and their Sensitivity to Parameter Variations . J. Aerosol Sci. , 21 : 675 – 688 . [CROSSREF] [CSA]
- Hofmann , W. and Koblinger , L. 1992 . Monte Carlo Modeling of Aerosol Deposition in Human Lungs. Part III: Comparison with Experimental Data . J. Aerosol Sci. , 23 : 51 – 63 . [CROSSREF]
- Hofmann , W. , Koblinger , L. , Brand , P. , Ferron , G. and Heyder , J. 1994 . The Effect of Convective Mixing on Particle Transport in the Human Respiratory Tract . Ann. Occup. Hyg. , 41 ( Suppl. 1 ) : 167 – 174 . [CSA]
- Hofmann , W. , Koblinger , L. , Bergmann , R. , Fleming , J. S. , Hashish , A. H. and Conway , J. H. 1997 . Spatial Aerosol Deposition Patterns in the Human Lung: Stochastic Predictions vs Experimental SPECT Data . Ann. Occup. Hyg. , 41 ( Suppl. 1 ) : 576 – 581 .
- Hofmann , W. , Morawska , L. and Bergmann , R. 2001 . Environmental Tobacco Smoke Deposition in the Human Respiratory Tract: Differences between Experimental and Theoretical Approaches . J. Aerosol Med. , 14 : 317 – 326 . [PUBMED] [INFOTRIEVE] [CROSSREF] [CSA]
- Hofmann , W. , Asgharian , B. and Winkler-Heil , R. 2002 . Modeling Intersubject Variability of Particle Deposition in Human Lungs . J. Aerosol Sci. , 33 : 219 – 235 . [CROSSREF]
- International Commisison on Radiological Protection (ICRP) . 1994 . Human Respiratory Tract Model for Radiological Protection , Oxford : Pergamon Press . ICRP Publication 66, Ann. ICRP 24, nos 1–3
- Koblinger , L. and Hofmann , W. 1985 . Analysis of Human Lung Morphometric Data for Stochastic Aerosol Deposition Calculations . Phys. Med. Biol. , 30 : 541 – 556 . [PUBMED] [INFOTRIEVE] [CROSSREF]
- Koblinger , L. and Hofmann , W. 1990 . Monte Carlo Modeling of Aerosol Deposition in Human Lungs. Part I: Simulation of Particle Transport in a Stochastic Lung Structure . J. Aerosol Sci. , 21 : 661 – 674 . [CROSSREF] [CSA]
- Martonen , T. B. , Schroeter , J. D. , Hwang , D. , Fleming , J. S. and Conway , J. H. 2000 . Human Lung Morphology Models for Particle Deposition Studies . Inhal. Toxicol. , 12 ( Suppl. 4 ) : 109 – 121 . [PUBMED] [INFOTRIEVE] [CROSSREF]
- Perring , S. , Summers , Q. , Fleming , J. S. , Nassim , M. A. and Holgate , S. T. 1994 . A New Method of Quantification of the Pulmonary Regional Distribution of Aerosols Using Combined CT and SPECT and its Application to Nedocromil Sodium Administered by Metered Dose Inhaler . Br. J. Radiol. , 67 : 46 – 53 . [PUBMED] [INFOTRIEVE]
- Raabe , O. G. , Yeh , H. C. , Schum , G. M. and Phalen , R. F. 1976 . Tracheobronchial Geometry: Human, Dog, Rat, Hamster , Albuquerque, New Mexico, , USA : Lovelace Foundation . Lovelace Foundation Report LF-53.
- Sauret , V. , Halson , P. H. , Brown , I. W. , Fleming , J. S. and Bailey , A. G. 2002 . Study of the Three-Dimensional Geometry of the Central Conducting Airways in Man, Using Computed Tomography (CT) Images . J. Anat. , 200 : 123 – 134 . [PUBMED] [INFOTRIEVE] [CROSSREF]
- Schroeter , J. D. , Fleming , J. S. , Hwang , D. and Martonen , T. B. 2002 . A Computer Model of Lung Morphology to Analyze SPECT Images . Comp. Med. Imaging Graph. , 26 : 237 – 246 . [CROSSREF] [CSA]
- Stahlhofen , W. , Scheuch , G. and Bailey , M. R. 1995 . Investigation of Retention of Inhaled Particles in the Human Bronchial Tree . Radiat. Prot. Dosim. , 60 : 311 – 319 .
- Sturm , R. and Hofmann , W. 2005 . Visualization of the Three-Dimensional Particle Deposition Patterns in the Lung Derived from Monte Carlo Modeling: Methodology and Various Applications . Comp. Biol. Med. , 35 : 41 – 56 . [CROSSREF] [CSA]
- Swithenbank , J. , Beer , J. M. , Taylor , D. S. , Abbott , D. and McGreath , G. C. 1977 . A Laser Diagnostic Technique for Measurement of Droplet and Particle Size Distribution . Prog. Astronaut. Aeronaut. , 53 : 421 – 437 .
- Weibel , E. R. 1991 . “ Design of Airways and Blood Vessels Considered as Branching Trees ” . In The Lung: Scientific Foundations , Edited by: Crystal , R. G. and West , J. B. 711 – 720 . New York : Raven Press .
- Yeh , H. C. and Schum , G. M. 1980 . Models of Human Lung Airways and their Application to Inhaled Particle Deposition . Bull. Math. Biol. , 42 : 461 – 480 . [PUBMED] [INFOTRIEVE] [CROSSREF]