Two new experimental procedures are proposed to evaluate the performance of DMAs operated with different gases for sheath and polydisperse-aerosol-carrier flows. The first procedure evaluates the potential flow mixing in DMAs. An organic compound vapor of heavy molecular weight is doped, and then its concentration in the DMA aerosol-carrier streams is detected by gas chromatography. DMAs that strongly separate the doped vapor from the carrier are qualified as the separation tools to extract particles of desired sizes from an organic-vapor-rich gas flow. The separation is of importance for the particle composition characterization using existing chemical analytic instruments.
The second procedure investigates the sizing accuracy of DMAs operated under the conditions of interest. A tandem DMA (TDMA) setup was applied to achieve the test objective. The first DMA was operated with different gases for sheath and polydisperse-aerosol-carrier flows. The same gas used for the first DMA sheath flow was used for both aerosol-carrier and sheath flows in the second DMA. This study evaluated the performance of Nano-DMA using different gas pairs (Ar, N2, CO2, and He). In the first test, at sheath flow rates of 7.5 and 15 lpm, approximately 0.1% or less of the organic vapor in the polydisperse-aerosol-carrier stream reached the DMA monodisperse-aerosol-carrier stream for gas pairings of Ar, N2, and CO2. For He with other gases, more organic compound vapor was detected at the Nano-DMA downstream, probably because He has high diffusivity when paired with other gas media. Either N2 or Ar gas was used as the Nano-DMA sheath flow in the second test to reduce the DMA's operational cost.
The second test shows that the classified particle size can be estimated from the measured electrical mobility using the gas property of sheath flow for cases of N2-Ar pairs. For the cases of He as polydisperse-aerosol-carrier flow with an other gas (N2/Ar) as the sheath flow, the classified particle size is less than estimated using the sheath flow gas property, possibly due to the diffusiophoresis effect. The experimental validation of the diffusiophoresis effect was further carried out by switching the roles of He-N2/Ar as the sheath and aerosol carrier flows in Nano-DMA.
1. INTRODUCTION
Differential mobility analyzers (DMAs) have been widely applied in a variety of aerosol studies and applications for particles in the submicron and nanometer diameter ranges. Typically, DMAs either size particles or classify relatively monodisperse particles from polydisperse particles. Examples include the popularly used SMPS (Scanning Mobility Particle Sizer, consisting of one DMA and one condensation particle counters, CPC, or aerosol electrometer) and monodisperse aerosol generation by the evaporation-condensation-and-DMA-classification technique (CitationLiu and Pui 1974). The other widely applied DMA system is the so-called tandem differential mobility analyzer (TDMA). The system includes two DMAs (one for obtaining monodisperse particles and the other for sizing particles after the first DMA-classified particles have passed through an aerosol conditioner), and one condensation particle counter. TDMA systems have been used to calibrate the performance of DMAs, to study the droplet growth or evaporation in a well-controlled aerosol conditioner (CitationRader et al. 1986), to measure the organic composition of fine aerosol (CitationJoutsensaari et al. 2000), to study the hydration properties of diesel soot particles (CitationWeingartner et al. 1996), to investigate the sintering rate of agglomerates (Koichi et al. 2001), to obtain the particle density of particles (CitationBoulaud et al. 1996), to characterize fuel oil combustion aerosol (CitationLeskinen et al. 1997), to study the particle coagulation rate constant (CitationChan et al. 2001), and to study hygroscopic behavior of atmospheric aerosol (CitationChen et al. 2003).
To study modern aerosol systems in a great detail, we need to characterize the particle composition and measure the particle size distribution. To achieve these tasks, aerosol researchers are marrying the sizing ability of DMAs with the chemical composition detection ability of existing chemical analytic instruments. Examples include DMA-TOFAS (Time-of-Flight Aerosol Spectrometer) (CitationMichael et al. 1976), DMA-ICP-AES (inductively coupled plasma-atomic emission spectrometry) (CitationWeber et al. 1990), DMA-ICP-MS (inductively coupled plasma mass spectrometry) (CitationMakynen et al. 1997; CitationMyojo et al. 2002), and TDPBMS (thermal desorption particle beam mass spectrometer) (CitationSakurai et al. 2003). In such systems, the presence of organic and/or semi-organic vapors in the sampled aerosol-laden stream becomes a concern. Unfortunately, organic and semi-organic compound vapors are usually present in modern aerosol reactors and combustion systems. To separate particles from a vapor-rich gas stream, CitationMyojo et al. (2002) used a DMA as a gas-exchange tool to displace particles from vapor-rich air to argon gas. The particle-laden argon gas flow was then introduced to ICP-MS for particle chemical analysis. Polystyrene latex (PSL) particles of known sizes (down to 100 nm) were used to calibrate the sizing accuracy of the test DMA. In a similar approach, a highly efficient electrostatic gas particle separator (an EAA-like apparatus, electrical aerosol analyzer) was used to displace particles in organic-vapor-rich air to inert gases (CitationFissan et al. 2003). Particles were extracted from the vapor-rich air and placed in an inert gas stream. A gas chromatograph was used to measure the organic vapor composition in particle-free air, and ICP-MS was utilized to analyze the chemical content of extracted particles.
Another application of DMAs involving the use of different gases for sheath and polydisperse-aerosol-carrier flows is monodisperse particle classification after nanoparticles are synthesized in aerosol reactors, in which organic or metal-organic precursors are often used. In some aerosol reactors, helium of very high purity is required to control the quality of synthesized particles. To reduce the DMA's operational cost, a different inert but less expensive gas, for example, N2, is used as the sheath flow. In this application, sizing accuracy is of concern. To address the concern, attempts have been made using the scanning electron microscope (SEM) and image analysis (CitationKarg et al. 1992). DMA-classified charged particles were collected on SEM tubs and their sizes were examined by SEM. Two concerns emerged. One is the representative size of the particles collected and imaged. The other is the sizing resolution of SEM-imaged particles close to the lower size detection limit of SEMs. The imaged particle size was further influenced by the threshold setting used to identify the particle boundary. For these reasons, instead of SEM, the DMA technique has been adopted by NIST (National Institute of Standard and Technology) for the sizing of 100 nm PSL particle standard reference material (CitationKinney et al. 1991). To the knowledge of the authors, there has been no experimental investigation on the sizing performance of DMAs operated with different gases for sheath and aerosol-carrier flows, especially for particles in the nanometer diameter range.
With the increased interest in exploring nanoscale applications of monodisperse nanoparticles, the above-described concerns need to be carefully addressed. A new experimental procedure is thus proposed to investigate the problems. Two tests are included in the procedure: the first evaluates the potential flow mixing in DMAs when operated with different gases for polydisperse-aerosol-carrier and sheath flows, and the second examines the sizing accuracy of DMAs under the operating conditions of interest.
2. EXPERIMENTAL SETUPS
A nanometer differential mobility analyzer (Nano-DMA, TSI model 3085; CitationChen et al. 1998) was selected as the test DMA. Gases used in this study were CO2, N2, Ar, and He. The experimental setups are described in detail below.
Experimental Setup to Investigate the Flow Mixing in Nano-DMA
is a schematic diagram of the experimental setup used to evaluate the potential flow mixing in a Nano-DMA when operated with different gases for the DMA sheath and polydisperse-aerosol-carrier flows. In this experiment, a vapor-rich and particle-free inert gas was used as the polydisperse-aerosol-carrier flow. The tested inert gas was either carbon dioxide (Grade 2.8), nitrogen (Grade 4.8), argon (Grade 4.8), or helium (Grade 4.7), all supplied by Airgas of Missouri. The vapor-rich stream was generated by splitting the total Nano-DMA polydisperse-aerosol-carrier gas flow into two streams. One split stream was bubbled through a liquid bottle of methyl tert-butyl ether (MTBE, Aldrich 99+%) prior to its mixing with the other. MTBE was selected because it is a common gasoline additive to promote more complete combustion and reduce emissions of carbon monoxide (CO) and organic compounds. Two laminar flowmeters were used to monitor the flowrates of the streams passing through the MTBE bottle and the total polydisperse-aerosol-carrier stream. The total flowrate was kept at 1.5 lpm. The Nano-DMA sheath flow gas was also selected from the listed gases. A helium-sealed rotameter (Cole-Parmer) was used to monitor the sheath flowrate of the Nano-DMA. A valve downstream from the Nano-DMA excess gas flow path was utilized to adjust the DMA aerosol-carrier flowrates to 1.5 lpm, which was monitored with a separate laminar flow meter. The Nano-DMA monodisperse-aerosol-carrier flow was sent directly into a gas chromatograph (HP 6890N) to perform online analysis of the concentration of MTBE vapor. The GC was equipped with a DB-wax high-resolution capillary column (30 mm × 0.53 mm × 1 um film), interfaced directly to a HP quadrupole mass spectrometer as the detector. The chromatographic analysis was optimized to provide the required degree of separation based on the resolution of target compounds. The GC was operated in the temperature-programming mode with an initial column temperature of 40°C for 5 min. Helium-carrier gas of ultrahigh purity was used with a flow rate of 3.4 ml/min in the GC. Two sheath-to-aerosol-carrier flowrate ratios were investigated in this experiment, 5:1 and 10:1. Prior to the experiment, all of the flowmeters in the setup were calibrated by a Galibrator (Gillian) with the listed gases. No voltage was applied on the Nano-DMA, because we were interested only in the investigation of potential flow mixing in this phase of the study.
Experimental Setup for Nano-DMA Sizing Evaluation
is a schematic diagram of the experimental setup used to investigate the sizing accuracy of a Nano-DMA when operated with different gases for the sheath and aerosol-carrier flows. In the setup, monodisperse silver particles were generated using the evaporation-condensation-and-DMA-classification technique. A Lingerberg tube furnace was used to evaporate silver powder (Aldrich, 99.99%) placed in a combustion boat located in the middle of a ceramic tube (Al2O3, Coors Ceramics Co). To produce polydisperse nanoparticles of desired mean sizes, the tube furnace temperature was varied over the range from 900 to 1100°C. Filtered gas (Ar, N2, or He) at 0.5–1.0 lpm was introduced through the ceramic tube to transport the silver vapor. The silver-vapor-rich stream was mixed with a cold stream of the same gas (1.5–2.5 lpm) to quench the hot, silver-vapor-rich stream at the exit of the ceramic tube. Polydisperse silver particles in the nanometer size range were then generated through nucleation and condensation. By varying the furnace temperature and the quenching flowrate, the mean size and concentration of polydisperse silver particles was thus controlled. To obtain monodisperse silver particles of the desired sizes, the polydisperse particle stream was passed through a Kr-85 radioactive neutralizer and a Nano-DMA. A laminar flowmeter was used to monitor the flowrate of the polydisperse aerosol stream. The sheath flow used in the Nano-DMA was either Ar or N2 gas. A Gillian Gilibrator was used to monitor the Nano-DMA sheath flowrate. A rotameter and a valve were used to monitor and control the excess aerosol and aerosol-carrier flowrates of the first DMA. To obtain each desired particle size, the applied voltage on this first Nano-DMA was calculated based on the gas properties of the first Nano-DMA sheath flow. To size particles classified by the first Nano-DMA, the monodisperse particle stream after the first Nano-DMA was then introduced to a SMPS system, consisting of a second Nano-DMA (TSI Model 3085), DMA platform (TSI Model 3080), and ultrafine condensation particle counter (UCPC; TSI Model 3025A). The mass flowmeters, used to monitor the sheath and excess flowrates, in the DMA platform was calibrated with test gases using the Galibrator prior to the experiment. With this setup, the first Nano-DMA was operated with different gases for the sheath and polydisperse-aerosol-carrier flows, and the flow gas used in the second Nano-DMA was the same as used in the first Nano-DMA sheath flow. The sizing accuracy of the first Nano-DMA can be obtained by comparing the particle diameter, Dp1, set by the first Nano-DMA to the peak particle diameter, Dp2, measured by the second Nano-DMA. The sheath and aerosol-carrier flowrates of both Nano-DMAs used in this experiment were kept at 15 and 1.5 lpm, respectively.
3. RESULT AND DISCUSSION
Evaluation of Flow Mixing in Nano-DMA
To investigate the potential flow mixing in the Nano-DMA operated with different gases for the sheath and polydisperse-aerosol-carrier flows, MTBE vapor was used as a tracer. This approach assumes that the organic compound vapor detected in the Nano-DMA monodisperse-aerosol-carrier flow is primarily due to gas mixing between the sheath and polydisperse-aerosol-carrier flows, instead of diffusion of the organic vapor. To minimize the vapor diffusion in the gas media used in the Nano-DMA, an organic compound of large molecular weight was selected. One can also estimate the diffusion length of the selected chemical compound vapor in the Nano-DMA gas media and compare it with the characteristic dimension of the Nano-DMA classification zone to support the selection. The chemical vapor diffusion length can be estimated by Equation (Equation1) (CitationHindes 1999):
shows the ratios of MTBE concentrations in monodisperse-aerosol-carrier and polydisperse-aerosol-carrier gas flows with different pairs of gases. The flowrate ratio of the sheath-to-polydisperse-aerosol-carrier flows was kept at 5:1 in this case (with a sheath flowrate of 7.5 lpm and aerosol carrier gas flowrate of 1.5 lpm). The data were obtained by taking the ratio of areas under the GC signal curves when introducing the aerosol-carrier gas flows into a GC. No calibration for the MTBE concentration measurement is needed in this GC application. Because the MTBE vapor can diffuse to the walls of tubing and the GC column, a background subtraction was also utilized during the analysis of the experimental data. A vapor-free helium gas was also used to purge the system for each run to keep the background minimal.
TABLE 1 Ratios of MTBE (methyl tert-butyl ether) vapor concentration in the monodisperse-aerosol-carrier flow to that in the polydisperse-aerosol-carrier flow when the Nano-DMA operates with different gases for sheath and aerosol-carrier flows (pairing among He, Ar, N2, and CO2): 7.5 lpm for the sheath flowrate and 1.5 lpm for the aerosol-carrier flowrate
As shown in , the area ratios are in general about 0.1% or less, except for the cases of pairing He with an other gas (Ar/N2/CO2). As expected, the best cases are when the Nano-DMA was operated with the same gas for the sheath and polydisperse-aerosol-carrier flows. DMAs are typically designed with this operational gas condition in mind. The cases of Ar-N2 pairs produced the second best result for the vapor-particle separation. For the combinations of CO2, N2, and Ar, all the ratios are all around 0.1%. However, the ratio is increased for the pairs of He with an other gas (Ar/N2/CO2), and the worst case occurs with the helium-argon pair where the flow mixing is probably due to the mutual gas diffusion between two gas streams.
Shown in are the binary gas diffusion coefficients and the diffusion lengths at the sheath flowrates of 7.5 and 15 lpm for different paired gases. The data given in were calculated using Equations (1) and (2). In general, the estimated diffusion lengths for all the gas pairs are less than 50% of the annular spacing (i.e., 0.971 cm) between the collection rod and outer cylinder of Nano-DMA, even at the lower sheath flow rate of 7.5 lpm. Further, the cases of the pairs of He and Ar/N2/CO2 have longer diffusion lengths when compared with those of other gas combinations. The longer diffusion length indicates a better chance for the MBTE vapor to reach the monodisperse-aerosol-carrier stream of Nano-DMA. It is consistent with the observation of the higher ratio values for the cases of He as the polydisperse-aerosol-carrier flow and an other gas (Ar/N2/CO2) the sheath flow. The ratios are even higher for the cases of He as the sheath flow and an other gas (Ar/N2/CO2) as the polydisperse-aerosol-carrier flow. This may be due to the diffusiophoresis effect (CitationDerjaguin and Yalamov 1972). The MTBE vapor can be viewed as particles in the nanometer size range. When Ar/N2/CO2 carrier gas diffuses into He sheath gas, the effect tends to move particles in the same direction due to the unbalanced molecular bombardment on either side of the MTBE molecules. The chance for MTBE vapor to reach the Nano-DMA downstream is thus increased.
TABLE 2 Binary diffusivities of different inert gas mixtures (pairing among He, Ar, N2, and CO2) and diffusion lengths at flow rates of 7.5 and 15 lpm
summarizes the MTBE vapor ratios when the Nano-DMA was operated at the sheath-to-polydisperse-aerosol-carrier gas flowrate ratio of 10 (with the polydisperse-aerosol-carrier flowrate kept at 1.5 lpm and sheath flowrate at 15 lpm). The experimental observations are similar to those obtained at the sheath flowrate of 7.5 lpm. The ratios for different paired gases at this DMA sheath flow rate are in general less than the data given in , indicating that less MTBE vapor can be detected at the monodisperse aerosol stream as the Nano-DMA sheath gas flowrate is increased. The result is primarily attributed to the reduction of the vapor residence time in the Nano-DMA because of the increased sheath flow rate. Note that the data for the pairs of CO2 (sheath)-He and CO2 (sheath)-Ar are not given in . At the 15 lpm CO2 flowrate, the flow passage in the pressure regulator froze due to a large pressure drop across the regulator after a period of the Nano-DMA operation. Steady flow control was impossible once the regulator was frozen and partially blocked by ice.
TABLE 3 Ratios of MTBE (methyl tert-butyl ether) vapor concentration in the monodisperse-aerosol-carrier flow to that in the polydisperse-aerosol-carrier flow when the Nano-DMA operates with different gases for sheath and aerosol-carrier flows (pairing among He, Ar, N2, and CO2): 15 lpm for the sheath flowrate and 1.5 lpm for the aerosol-carrier flowrate
Evaluation of Nano-DMA Sizing Accuracy
The described tandem DMA setup was used in this phase of the study to determine the sizing accuracy of DMA-classified particles obtained from operating DMAs with different gases for the carrier and sheath. In this part of the investigation we studied only cases in which the organic-compound vapor concentration in the Nano-DMA classified particle stream was less than 0.1% of that in the polydisperse-aerosol-carrier flow of the Nano-DMA. We also excluded the cases paired with CO2 gas. This exclusion stemmed from the technical difficulty of controlling a steady CO2 flow stream for an extensive period of time during the experiment. Further, we decided to study only selected cases at the sheath flowrate of 15 lpm. This decision was make simply to minimize the chance for the polydisperse-aerosol-carrier gas to penetrate deeply into the sheath flow and eventually reach the monodisperse-aerosol-carrier stream. The minimization of the chance of gas penetration is further supported by the binary diffusion lengths of N2-Ar, N2-He, He-Ar pairs (given in ) at the sheath flowrate of 15 lpm. In general, the lengths are less than 35% of the annular spacing between the Nano-DMA collection rod and its outer cylinder, i.e., the Nano-DMA characteristic particle classification zone. Since the gas of the Nano-DMA polydisperse-aerosol-carrier flow has much less chance to get in the monodisperse-aerosol-carrier gas stream in the selected cases operated at the chosen sheath flow rate, the size distribution of classified particles can be measured by a SMPS (scanning mobility particle sizer), consisting of a Nano-DMA, a DMA platform, and a ultrafine condensation particle counter (UCPC). By applying a fixed voltage on the first Nano-DMA, particles of an unknown size, Dp1, were classified. The set voltage for particles of the Dp1 size was calculated with the assumption that the central electrical mobility of DMA-classified particles could be estimated using the gas properties of the first Nano-DMA sheath flow. By scanning the voltage on the second Nano-DMA, the peak diameter, Dp2, of the measured size distributions was recovered from the measured peak voltage, using the gas property of the second Nano-DMA sheath flow. Because the same gas was used as the aerosol-carrier and sheath flows in the second Nano-DMA, the accuracy of Dp2 measurement was determined by the errors in the sheath flowrate, applied voltage, and Nano-DMA physical dimension measurements. In this test the aerosol-carrier flowrate was always kept at 1.5 lpm.
The relation between the electrical mobility of particles Z pc classified by DMAs and a certain voltage V applied to the DMA central rod is given in the following equation (CitationKnutson and Whitby 1975)
TABLE 4 Gas properties (viscosity and mean free path) of Ar, N2, and He at 20°C used in Equation (Equation4)
The uncertainty in Dp1 and Dp2 can be estimated from Equations (Equation3) and (Equation4), given the uncertainties of q c , V, μ, C, and λ. The relative error in the voltage is less than 0.1% because of the accurate calibration of the high voltage power supply. We neglect the uncertainties of μ and λ due to a lack of information. An approximate 2% relative error for the slip coefficient C is typically encountered when gases other than air are used (CitationRadar 1990). Since rotameters were used to monitor the sheath flow, q c , in the experiment, the relative error of the sheath flowrate was estimated at less than 5%. The relative errors propagated through Equations (3) and (4) for Dp1 and Dp2 were thus estimated accordingly. For each particle size, five different runs were performed for the Dp2 measurement. The average Dp2 of five runs are presented in , , , . The propagated relative errors of Dp1 are shown in all the figures as an error bar in the horizontal direction. The magnitude of each Dp2 error bar (in the vertical direction) shown in the plots is the sum of the propagated relative error and the relative variation of five runs.
FIG. 3 Comparison of estimated, Dp1, and measured, Dp2, particle sizes for the gas pairs of (a) Ar-Ar and (b) N2-N2. The sheath and aerosol-carrier flowrates were kept 15 and 1.5 lpm, respectively. The estimated particle size was based on the gas property of the sheath flow used in the Nano-DMA.
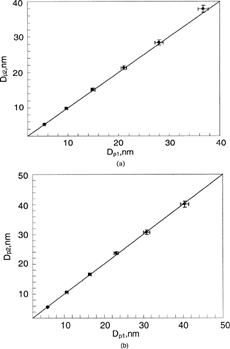
FIG. 4 Comparison of estimated, Dp1, and measured, Dp2, particle sizes when Nano-DMA was operated with the Ar-N2 composition: (a) Ar as the aerosol-carrier gas and N2 as the sheath gas; (b) N2 as the aerosol carrier gas and Ar as the sheath gas. The sheath and aerosol-carrier flowrates were kept at 15 and 1.5 lpm, respectively. The estimated particle size was based on the gas property of the sheath flow used in the Nano-DMA.
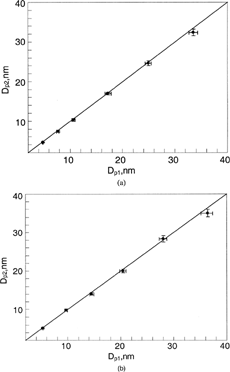
FIG. 5 Comparisons of estimated, Dp1, and measured, Dp2, particle sizes when Nano-DMA was operated with the gas combination of He-Ar: (a) Ar as the sheath flow and He as the aerosol carrier flow; (b) He as the sheath flow and Ar as the aerosol carrier flow. The sheath and aerosol-carrier flowrates were kept at 15 and 1.5 lpm, respectively. The estimated particle size was based on the gas property of the sheath flow used in the Nano-DMA.
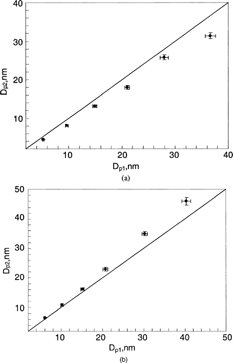
FIG. 6 Comparisons of estimated, Dp1, and measured, Dp2, particle sizes when Nano-DMA was operated with the combination of He-N2: (a) N2 as the sheath flow and He as the aerosol carrier flow; (b) He as the sheath flow and N2 as the aerosol carrier flow. The sheath and aerosol-carrier flowrates were kept at 15 and 1.5 lpm, respectively. The estimated particle size was based on the gas property of the sheath flow used in the Nano-DMA.
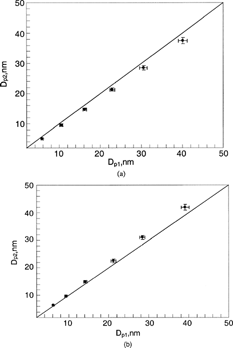
Before running the pairs of different aerosol-carrier and sheath flow gases, reference experiments were performed. In these reference experiments, Ar-Ar and N2-N2 gas combinations were used. The comparison between the estimated and measured particle sizes (Dp1 v.s. Dp2) is shown in . The one-to-one line is also given in the figure as the reference for perfect agreement between Dp1 and Dp2. As expected, all the data points are lie closely near the one-to-one line, which indicates good agreement between two particle sizes was obtained in these cases.
shows the comparison of estimated and measured sizes (Dp1 v.s Dp2) when the Nano-DMA was operated with the Ar-N2 combination. Two different conditions were studied in this gas pair. (a) is for the case of N2 as the sheath flow and Ar as the aerosol-carrier gas and for the reversed case. Six different particle diameters for each condition were chosen to evaluate the Nano-DMA sizing accuracy. For each particle size, the value of Dp2 is the average of five runs. The relative errors of Dp1s and Dp2s are shown in the figure as error bars. The one-to-one line is also graphed in the figure as a reference. In general, the particle diameter, Dp1, set by the first Nano-DMA is in reasonably good agreement with the peak diameter, Dp2, measured by the second Nano-DMA. The data points for particles of larger sizes seem to lie slightly below the one-to-one line when compared with those for smaller particle sizes. However, the deviation is not conclusive, due to the experimental uncertainty.
and are comparisons for the combinations of Ar (sheath)-He and N2 (sheath)-He, respectively. Helium gas was used as the polydisperse-aerosol-carrier gas flow in both gas pairs. A significant difference between the measured particle size, Dp2, and the estimated, Dp1, was observed for all the test particle sizes. All the data points are located below the one-to-one line, which indicates that the actual particle size, Dp2, of classified particles is less than the estimated particle size, Dp1. The deviation is more obvious for larger particle sizes. By comparing two figures, one can further see that the difference in the Ar-He pair is more significant than that in the N2-He pair. The finding is contradictory to the inference from the hypothesis that the binary gas diffusion in Nano-DMA alters the effective gas property and thus affects the classified particle size. If the hypothesis were correct, the Dp1 should have been less than Dp2, because the voltage setting for the Dp1 classification was calculated using the gas properties of sheath flow in the first Nan-DMA. The gas viscosities and mean free paths of He, Ar, and N2 are given in . From the table, one can expect that the effective viscosity of the N2-He mixture should remain nearly unchanged and the mean free path should increase slightly compared with those of pure N2 gas. For the Ar-He mixture, the effective viscosity should be reduced slightly and the mean free path should increase slightly compared with those for pure Ar gas. The effects of the reduced viscosity and/or the increased mean free path on the classified particle size should lead to a larger particle size when the applied voltage and sheath flowrate are kept constant (according to Equation (Equation4)). The difference can not be explained by the flow fluctuation in the Nano-DMA either, because the spreading of the measured size distributions is nearly identical for these gas pairs and pairs of the same gas. One possible explanation for this observation is the diffusiophoresis effect (CitationDerjaguin and Yalamov 1972). The effect is the result of unequal gas molecular bombardment on either side of the particles due to the concentration gradient established in the diffusing gas mixture. In these studied cases, the N2/Ar pair of heavy molecular weight was diffusing towards the Nano-DMA's outer cylinder and the helium gas of light molecular weight is moving in the opposite direction. Due to the unequal molecular bombardment, charged particles in the Nano-DMA will experience an additional force in the direction of the moving heavy gas (i.e., in the opposite direction of the established DMA-electrical field). With constant voltage and flow rates applied, particles of smaller sizes—of higher electrical mobility, would be classified due to the need to overcome the diffusiophoretic effect. The effect was expected to be more severe when Ar gas was used as the sheath flow, consistent with the experimental observation.
To further experimentally validate the hypothesized diffusiophoretic mechanism, we also exchanged the roles of the sheath and aerosol-carrier gases used in the cases presented in and , that is, He as sheath gas and Ar/N2 as carrier gas. The result is shown in and . All the data points in the figures are located in the area above the one-to-one line, indicating that the actual particle size, Dp2, of classified particles is larger than the estimated particle size, Dp1. The deviation is more obvious as the particle size increases. In these two cases, the N2/Ar pair of heavy molecular weight was diffusing towards the Nano-DMA's inner rod and the helium gas of light molecular weight is moving towards the outer cylinder. Because of the diffusiophoretic effect, larger particles would be classified. The effect was even more pronounced for the case with Ar as the aerosol carrier flow gas.
4. SUMMARY
Differential mobility analyzers (DMAs) are powerful tools for sizing and classifying particles in the submicron and nanometer size ranges. The operation of DMAs typically assumes the same gas for the sheath and polydisperse-aerosol-carrier flows. In some modern applications of nanoparticles of high purity, DMAs operated with different gases for the sheath and aerosol-carrier flows are used to classify monodisperse nanoparticles generated in aerosol reactors. For example, helium gas of very high purity is usually employed to closely control the particle synthesis in aerosol reactors.
In this work, however, a different and less expensive gas is proposed for the sheath flow to reduce the operational cost. The accuracy of DMA-classified size is of concern in this kind of applications. In other applications, DMAs are utilized as a separator to produce an inert gas stream with desired size nanoparticles for chemical composition characterization. The particle-vapor separation is of importance because of interference from the presence of organic vapor in the aerosol-carrier gas stream, especially in particle streams sampled from the exhaust of combustion systems or aerosol reactors. Both the flow mixing and sizing accuracy of DMAs are concerns in these applications.
Two new experimental procedures were proposed in this work to evaluate DMA performance using different gases for the sheath and polydisperse-aerosol-carrier flows. The first procedure evaluated flow mixing in DMAs using a vapor tracer dopant in the polydisperse-aerosol-carrier flow. The second procedure evaluated the particle sizing accuracy of DMAs using the tandem DMA technique. In this study, the procedure was realized with a Nano-DMA. Gases used in this work were CO2, N2, Ar, and He. In the procedure, MBTE vapor was introduced into the polydisperse-aerosol-carrier flow as the tracer, and its concentration in the monodisperse-and polydisperse-aerosol-carrier flows were detected by a highly-sensitive GC. The ratio of the two readings indicates the level of flow mixing in the Nano-DMA. At a sheath flowrate of 7.5 lpm, approximately 0.1% or less of the MBTE vapor in the polydisperse-aerosol-carrier stream could be detected in the DMA monodispers-aerosol-carrier stream for gas pairings among CO2, N2, and Ar. The increase of sheath flowrate to 15 lpm further reduced the MBTE vapor concentration in the monodisperse-aerosol-carrier stream. For the cases pairing He (as the polydisperse-aerosol-carrier flow) with an other gas (as the sheath flow), the Nano-DMA was operated at a sheath flowrate of 15 lpm to achieve similar particle-vapor separation performance as in the cases of pairing among N2, Ar, and CO2. The worst separation performance occurred in the cases in which helium was used as the sheath flow and an other gas as the polydisperse-aerosol-carrier flow. Binary gas diffusion is one possible explanation for the observation. The detected ratio variation is consistent with the change of the binary gas diffusion lengths as different gases are paired.
In the second procedure of this study, a tandem DMA setup was used. The first Nano-DMA operated with different gases for sheath and polydisperse-aerosol-carrier flows, and the second Nano-DMA used the same gas as the first Nano-DMA sheath flow for the carrier as well. In this test, only Ar or N2 gas were used as the Nano-DMA sheath flow. The N2-Ar combination gave a good agreement between Dp1, the particle diameter classified by the first Nano-DMA, and Dp2, the peak particle diameter measured by the second Nano-DMA. For pairs of He-Ar and He-N2, a significant difference between Dp1 and Dp2 was observed, possibly due to the diffusiophoresis effect. With He as carrier flow gas and Ar/N2 as sheath flow gas, the effect creates additional force acting on particles in the direction opposite to the imposed electrical force in the Nano-DMA classification zone. The net result is that particles of smaller size—having higher electrical mobility—were classified. In contrast, particles of larger size were classified when the aerosol carrier and sheath flow gases were switched, that is, He as sheath gas and Ar/N2 as carrier flow.
Acknowledgments
The authors are grateful for the financial support provided by National Science Foundation (Grant No. CTS-0304649) and Department of Energy Office of Science Environmental Management Science Program through a contract by the Oak Ridge National Laboratory.
REFERENCES
- Allen , M. D. and Raabe , O. G. 1985 . Slip Correction Measurements of Spherical Solid Aerosol Particles in an Improved Millikan Apparatus . Aerosol Sci. and Technol. , 4 : 269 – 286 . [CSA]
- Boulaud , D. , Pourprix , M. , Gougeon , R. , Bronec , E. L. and Renoux , A. 1996 . On the Radial Flow Differential Mobility Analyzers for the Determination of Aerosol Particle Density and Mass . J. Aerosol Sci. , 27 : S307 – S308 . [CSA]
- Chan , T. W. and Mozurkeewich , M. 2001 . Measurement of the Coagulation Rate Constant for Sulfuric Acid Particles as a Function of Particle Size Using Tandem Differential Mobility Analysis . J. Aerosol Sci. , 32 : 321 – 339 . [CSA]
- Chen , D. , Pui , D. Y. H. , Hummes , D. , Fissan , H. , Quant , F. R. and Sem , G. J. 1998 . Design and Evaluation of a Nanometer Aerosol Differential Mobility Analyzer (Nano-DMA) . J. Aerosol Sci. , 29 : 497 – 509 . [CSA]
- Chen , L. Y. , Jeng , F. T. , Chen , C. C. and Hsiao , T. C. 2003 . Hygroscopic Behavior of Atmospheric Aerosol in Taipei . Atmospheric Environment , 37 : 2069 – 2075 . [CSA]
- Derjaguin Yalamov , B. V. and Yu. , I. 1972 . “ The Theory of Thermophoresis and Diffusiophoresis of Aerosol Particles and Their Experimental Testing ” . In International Review in Aerosol Physics and Chemistry Edited by: Hindy , G. M. and Brock , J. R. Vol. 3 , p1-200
- Fissan , H. and Asbach , C. 2003 . Development and Evaluation of a Highly Efficient Electrostatic Gas Particle Separator with Minimal Effect on the Gas Phase . AAAR conference . 2003 , Anaheim, CA.
- Fissan , H. , Hummes , D. , Stratmann , F. , Buscher , P. , Neumann , S. , Pui , D. Y. H. and Chen , D. 1996 . Experimental Comparison of Four Differential Mobility Analyzers for Nanometer Aerosol Measurements . Aerosol Sci. and Tech. , 24 : 1 – 13 . [CSA]
- Fuller , N. E. , Schettler , D. P. and Giddings , J. C. 1966 . A New Method for Prediction of Binary Gas-Phase Diffusion Coefficients . J. Ind. Eng. Chem. , 58 : 19 – 27 . [CSA]
- Hinds , W. C. 1999 . Aerosol Technology: Properties, Behavior, and Measurement of Airborne Particles , 2nd edition , 156 New York : John-Wiley & Son .
- Hummes , D. , Stratmann , F. , Neumann , S. and Fissan , H. 1996 . Experimental Determination of the Transfer Function of a Differential Mobility Analyzer (DMA) in the Nanometer Size Range . Part. Part. Syst. Charact. , 13 : 327 – 332 . [CSA]
- Joutsensaari , J. , Vaattovaara , P. , Laaksonen , A. , Vakeva , M. and Hameri , K . 2000 . Organic Tandem Differential Mobility Analyzer . J. Aerosol Sci. , 31 : S775 – S776 . [CSA]
- Karg , E. , Dua , S. K. and Ferron , G. A. 1992 . Performance of a Differential Mobility Analyzer at Different Gas Composition . J. Aerosol Sci. , 23 : S1389 – 392 . [CSA]
- Kinney , P. D. , Pui , D. Y. H. , Mulholland , G. W. and Bryner , N. P. 1991 . Use of the Electrostatic Classification Method to Size 0.1 mm SRM Particle-A Feasibility Study . J. Res. Natl. Inst. Stand. Technol. , 96 : 147 – 176 . [CSA]
- Knutson , E. O. and Whitby , K. T. 1975 . Aerosol Classification by Electric Mobility: Apparatus, Theory, and Applications . J. Aerosol Sci. , 6 : 443 – 451 . [CSA]
- Koichi , N. , Shimadaa , M. , Okuyama , K. and Deppertb , K. 2002 . Evaluation of the Change in the Morphology of Gold Nanoparticles during Sintering . J. Aerosol Sci. , 33 : 1061 – 1074 . [CSA]
- Leskinen , A. , Alander , T. and Raunemaa , T. 1997 . Tandem DMA Technique in Analysis of Light Fuel Oil Combustion Aerosol . J. Aerosol Sci. , 28 : S545 – S546 . [CSA]
- Liu , B. Y. H. and Pui , D. Y. H. 1974 . A Submicron Aerosol Standard and the Primary Absolute Calibration of the Condensation Nuclei Counter . J. Colloid Interface Sci. , 47 : 155 – 171 . [CSA]
- Makynen , J. M. , Jokiniemi , J. K. , Ahonen , P. P. , Kauppinen , E. I. and Zilliacus , R. 1997 . AHMED Experiments on Hygroscopic and Inert Aerosol Behavior in LWR Containment Conditions: Experimental Results . Nuclear Engineering and Design , 178 : 45 – 59 . [CSA]
- Michael , H. , Schwartz , H. and Ronald , P. A. 1976 . Theoretical Basis of the Time-of-Flight Aerosol Spectrometer: A Method for Monitoring the Size Distribution of Submicron Aerosol Particles . J. Aerosol Sci. , 7 : 281 – 296 . [CSA]
- Myojo , T. , Takaya , M. and Ono-Ogasawara , M. 2002 . DMA as a Gas Converter from Aerosol to “Argonsol” for Real-Time Chemical Analysis Using ICP-MS . J. Aerosol Sci. and Tech. , 36 : 76 – 83 . [CSA]
- Rader , D. J. 1990 . Momentum Slip Correction Factors for Small Particles in Nine Common Gases . J. Aerosol Sci. , 21 : 161 – 168 . [CSA]
- Rader , D. J. and Mcmurry , P. H. 1986 . Application of the Tandem Differential Mobility Analyzer to Studies of Droplet Growth or Evaporation . J. Aerosol Sci. , 17 : 771 – 787 . [CSA]
- Sakurai , H. , Tobias , H. J. , Park , K. , Zarling , D. , Docherty , K. S. , Kittelson , D. B. , McMurry , P. H. and Ziemann , P. J. 2003 . On-line Measurements of Diesel Nanoparticle Composition and Volatility . Atmospheric Environment , 37 : 1199 – 1210 . [CSA]
- Scheibel , H. G. and Porstendorfer , J. 1983 . Generation of Monodisperse Ag and NaCl Aerosol with Particle Diameters between 2 and 300 nm . J. Aerosol Sci. , 14 : 113 – 126 . [CSA]
- Weber , A. , Baltensperger , U. , Gaggeler , H. W. , Keil , R. , Tobler , L. and Schmidt-Ott , A. 1990 . In-Situ Studies of Silver Agglomerates by ICP-AES and a CNC . J. Aerosol Sci. , 21 : S55 – S58 . [CSA]
- Weingartner , E. , Burtscher , H. and Baltensperger , U. 1996 . Hydration Properties of Diesel Soot Particles . J. Aerosol Sci. , 27 : S695 – S696 . [CSA]