The aim of the project was to investigate the interactions between micronized salbutamol sulphate, budesonide, and formoterol fumarate dihydrate and different canister surfaces materials (Aluminium, anodized aluminium, perfluoroalkoxy, fluorinated ethylene propylene—polyether sulphone, and polytetrafluoroethylene) used in pressurized metered dose inhalers (pMDIs).
The surface component approach for polar and apolar interfacial interactions was used to predict the adhesion behavior of micronized drugs with the inner surfaces of pMDI canisters. This was achieved using a combination of in situ colloid probe atomic force microscopy (AFM) measurements and theoretical treatment of the surface free energy measurements, via a contact angle–based technique of the interacting surfaces.
All three drugs exhibited similar dispersive surface energy free values. A greater variation was, however, found in the polar component of the surface free energy measurements. These results were also reflected in the dispersive and polar components of the canister materials. Moreover, the linear relationship between the work of adhesion and AFM measured adhesion was shown to be correlated on the polar contributions of the surface free energies of the interacting materials. AFM measurements indicated that salbutamol sulphate was found to have the strongest adhesive forces with respect to the canister surface materials while budesonide and formoterol fumarate dihydrate appeared to have similar adhesive characteristics. The present study suggests that investigations into the design and characterization of pMDI formulations would benefit from considerations of the polar contribution of the surface free energy and relative work of adhesion of the drug and various components of a pMDI system.
INTRODUCTION
The majority of pressurized metered dose inhaler (pMDI) formulations are based on suspensions of micronized drug in propellant, with or without stabilizing excipients. Historically, device materials used with both chlorofluorocarbon (CFC) and hydrofluoroalkane (HFA) propellants have been found to cause suboptimal operation due to elastomer swelling, polymer extraction, poor lubrication, adsorption, and adhesion of the formulation to container walls (CitationTiwari et al. 1998). Examples of recent patents dealing with these issues include US 6,143,277 (CitationAshurst et al. 1996) which describes coating the inside of aerosol containers with fluorocarbon and non-fluorocarbon polymers.
Within a suspension-based pMDI system, there is a considerable risk for irreversible adhesion of micronised drug particulates to internal canister wall. For example, an increase in the force of adhesion between drug particles and canister walls may result in a decrease in emitted dose (CitationMichael et al. 2001; CitationYoung et al. 2003). When considering low dose medicaments (such as formoterol fumarate dihydrate: approximately 6–12 μ g/dose) the possibility of drug loss to the canister wall becomes critical, since the internal surface area of the canister may theoretically approach the projected surface area of the total suspended drug.
To date, research has focused on the nature and range of the interactions presents in aqueous and non-aqueous systems. However, only a limited amount of research has been conducted on investigating the physical interactions between drug particles and pMDI canister surfaces (CitationRogueda et al. 2003). Furthermore, such observations have generally been empirical and have studied the subject in a semi-quantitative manner (CitationYoung and Buckton 1990; CitationChiboswki et al. 1992; CitationParson et al. 1992; CitationPodczeck et al. 1996; CitationYoung et al. 2003). Consequently, a more rational approach into the understanding of these interactions would be beneficial in formulating pMDI suspensions.
The primary objective of this quantitative study is to assess the direct contribution of the relative interactions between drug particulates and a series of pMDI canister materials. Furthermore, by applying the Surface Component Approach (SCA) model for colloidal systems (CitationTraini et al. 2005), it may be possible to relate theoretical adhesion measurement to practical measurements of the surface energies of interacting surfaces. More specifically, the study involved an attempt to correlate the interfacial free energy of interaction (thermodynamic work of adhesion), modelled using the van Oss theory for interfacial forces in non-aqueous media and the surface energy of different polymer coated and non-coated aluminium canister walls (measured using conventional contact angle methods) with direct adhesion measurements of micronised drug probes on respective surfaces (using atomic force microscopy, AFM).
Surface Component Approach for Particle Interaction
The traditional DLVO theory (CitationDerjaguin and Landau 1941; CitationVerwey and Overbeek 1948) is based on the assumption that interactions between two molecules or particles suspended in a liquid consists of the sum of the attractive London–van der Waals (LW) dispersion forces, and the predominantly repulsive electrostatic interaction (electrical double layers). However, such theory has not been fully validated in non-aqueous pMDI media (CitationVervaet and Byron 1999; CitationSmyth 2003).
In the system under investigation the interactions involved are more complex: London–van der Waals forces, electrostatic double layer interactions and Lewis acid/base interactions (comprise of hydrogen bonding and entropic contribution) (Citationvan Oss et al. 1988; Citationvan Oss 1994) all come into play when two particle surfaces approach closer than a few nanometers in distance. Due to the overlay of a very diffuse electrical double layer (derived from a combination of a low dielectric constant and low ionic strengths), the electrostatic repulsive forces acting between particles are very small (CitationKitahara 1974; CitationPugh et al. 1983; CitationWyatt and Vincent 1989). It is predicted, therefore, that the LW and surface polarity factors will predominate in such systems. With the aim of understanding adhesion of drug particulates to canister walls in non-aqueous systems, the theory for interactions of this kind of system is reviewed in the following section.
A more complete overview of the surface component approach (SCA) can be found in the literature (Citationvan Oss et al. 1988; Citationvan Oss 1994; CitationTraini et al. 2005). Briefly, the SCA model for the stability of colloidal particles considers two types of interactions: the apolar Lifshitz–van der Waals component (which comprises the dispersion, as well as the induction and orientation contributions to the van der Waals interactions) and a polar, or Lewis acid-base component.
As the apolar and polar components of the free energies of interfacial interaction is additive (CitationFowkes 1983; Citationvan Oss et al. 1988), the total surface free energy of a solid is determined by the sum of the apolar (γLW) and the polar (γAB) component.
Therefore, according the Good-Girifalco-Fowkes combining rule (CitationGood and Girifalco 1960; CitationFowkes 1964), the interfacial energy parameters between dissimilar solid surfaces (1 and 2) in an apolar medium (3), γ 3 + and γ3 − = 0), the free energy of interaction can be used in the simplified form (Equation [1]):
Accordingly, the force of adhesion can be related to the thermodynamic work of adhesion (Wad = ΔG132) by applying one of the contact mechanics models. The Derjaguin-Müller-Toporov (DMT) model is typically applied to small particles with a high stiffness and a small curvature radius, while the Johnson, Kendall, and Roberts (JKR) model is applicable for systems with large particle radii, high surface energies, and compliant materials (CitationJohnson et al. 1971; CitationDerjaguin et al. 1975). Both models are valid depending on the interacting materials and their geometries.
The relationship between the force of adhesion (Fad) and the thermodynamic work of adhesion (W ad) is represented in the following equation:
Theoretical Estimation and Direct Surface Energy Measurements Correlation
This study investigated the correlation of the interfacial free energy of interaction, developed using the theoretical SCA model in non aqueous media, and particle adhesion measurements on different polymer coated and non-coated aluminium canister walls. Theoretical adhesion values were calculated from contact angle measurements, while in situ adhesion measurements of micronized drug probes on the respective surfaces were conducted using atomic force microscopy (AFM) (CitationBinnig and Quate 1986).
MATERIALS AND METHOD
Micronized salbutamol sulphate (salbutamol), budesonide, and formoterol fumarate dihydrate (formoterol) were supplied by AstraZeneca (R & D Charnwood, Loughborough, UK). Water was produced by reverse osmosis (Millipore, Molsheim, France). All organic solvents were supplied by BDH (Poole, UK) and were of analytical grade. The model propellant, 2H, 3H decafluoropentane (mHFA), was supplied by Apollo Scientific (Derbyshire, UK) and was purified according method described elsewhere (CitationGoebel and Lunkenheimer 1997). The subsequent purity of the mHFA was in excess of 99.9%, with moisture content less than 9 ppm. Water diffusion into the system could have profound impact on the propellant polarity and consequently particle adhesion (CitationBlondino and Byron 1998; CitationMiller 1990). Subsequently, fresh samples of mHFA were used for each experiment. The canister materials were: Aluminium (ALU), anodise aluminium (AN-ALU), and polymer coated pMDIs (perfluoroalkoxy (PFA), fluorinated ethylene propylene–polyether sulphone (FEP–PES) and polytetrafluoroethylene (PTFE)) (Presspart, Lancashire, UK). All were used as supplied.
Scanning Electron Microscopy of pMDI Canister Materials
Scanning electron microscopy (SEM) was used to characterize the morphology of the canister surfaces. Samples were fixed on adhesive black carbon tabs, which were pre-mounted on aluminium stubs and coated with a thin gold film using a sputter coater (model S150B, Edwards High Vacuum, Sussex, UK). Samples were imaged using a JEOL 6310 SEM (Jeol, Tokyo, Japan) at 10 KeV.
Surface Morphology of the Canister Materials
Previous investigations have suggested that canister morphology significantly influences the drug-surface adhesion (CitationYoung et al. 2003). The morphology of the substrates was therefore checked by AFM. Surface materials were cut from commercially available pMDI canisters, using metal snips, to produce ∼ 1 cm2 substrates suitable for analysis. Each substrate was mounted on an AFM sample stub with epoxy resin, washed with isopropyl alcohol (commonly used to clean surfaces in the pharmaceutical industry), and dried with nitrogen prior to analysis.
Detailed topographical information of the three drug crystal surfaces was acquired by AFM. Imaging was conducted in air, using Tapping ModeTM with a high-aspect-ratio silicon probe (OTESP, Digital Instruments, UK), at a scan rate of 0.7 Hz.
All AFM studies were performed using a commercially available Multi Mode AFM with a Nanoscope III controller (Digital Instruments (DI), Cambridge, UK). The root mean squared surface roughness (Rrms) of the deviations was then calculated from the AFM height data over a 5 μ m × 5 μ m area via the following Equation (Equation3):
Model Drugs Compact Preparation
Drugs surface energy measurements were obtained from compacts. Model surfaces from the micronized drug materials were prepared by direct compression using a servo-hydraulic press (Specac, Model 25010, Specac Ltd., Kent, UK). Approximately 250 mg of micronised drug material was weighed into a 10 mm stainless steel die, spread evenly in an attempt to ensure constant porosity throughout the disc, and compacted at a compression force of 10 kN. Prepared drug compact surfaces were stored in tightly sealed containers in a controlled environment (25°C, 44% RH) for at least 24 hours prior to use.
Contact Angle Measurements for Model Drugs Compacts and Canister Surfaces
Surface free energy of the canister surfaces and drug materials were investigated using the sessile drop method (CitationGood and Girifalco 1960; CitationGood and Stromberg 1979; Citationvan Oss et al. 1988; CitationGood 1992; CitationBuckton et al. 1995). The canister materials were washed with methanol and dried using filtered nitrogen prior to analysis. However, the drug materials were formed into model compacts using a method described above.
Equilibrium advancing contact angle (CA) of each sample was measured with a NRL goniometer (Rame'-Hart Inc., Mountain Lakes, New Jersey, USA) equipped with a 2.3X objective lens and a 10X Ramsden type eyepiece. Measurements were conducted under ambient conditions (25°C, 44% RH) using three liquids (water, diiodomethane and ethylene glycol) with known non-polar, acid (electron acceptor), and base (electron donor) surface tension components. The total surface free energy (γTot) of each material was then calculated from the sum of non-polar and polar components (Citationvan Oss et al. 1998) using the Young-Duprè equation (Equation4):
In Situ Atomic Force Microscopy Measurements
The interactions of drug particles with canister surfaces, were evaluated using AFM equipped with an in situ cell (DI, Cambridge, UK), using mHFA. The mHFA contained a saturated solution of the respective drugs under investigation (CitationTraini et al. 2005). It is essential to note that conventional AFM systems are currently limited to studies in air and/or low vapour pressure liquid environments. Thus, mHFA was chosen for its similarities to the physico-chemical properties of HFA227, used in pMDIs (CitationRogueda 2003, ).
TABLE 1 Comparative table of some properties of propellants mHFA and HFA227
The use of mHFA as a model system for studying the behavior of pMDIs, when characterization methods cannot be adapted to the pressure regimen, has been extensively studied by CitationRogueda (2003).
The AFM is an ideal tool for the single particle studies because it can provide quantitative information on both the separation energy (via force–distance measurements) and surface geometry (via topographic images). Individual micronised drug particles were mounted onto micro-fabricated AFM cantilevers (nominal spring constant 0.38 N · min− 1) using a quick setting epoxy resin. Extreme care was taken during drug probe preparation to limit the amount of drug-glue contact. Detailed methodology for drug probe preparation and measurements are described elsewhere (CitationDucker et al. 1991; CitationYoung et al. 2004).
The integrity of all three drug probes were investigated prior to and post measurement using a high-magnification 500× long-working-distance reflective microscope. Drug probes appeared visibly proud of the cantilever surface, with no observable differences between the start and end of the experimental procedure (indicating no macroscopic change in drug probe morphology).
The interaction (adhesion) between each drug probe and canister surface material was measured using the colloid probe approach under in situ conditions with mHFA (CitationDucker et al. 1991; CitationYoung et al. 2003) by ramping the drug probe, toward, in contact, and away from the surface. This produced a force-distance curve which could be examined to produce the force of adhesion and energy of separation.
A total of 4096 force–distance curves were determined for each individual drug probe and three substrate surfaces (scan area: 10 × 10 μ m) under in situ conditions with the following settings: approach retraction cycle 500 nm, cycle rate 4.07 Hz, and constant compliance region 60 nm and a compressive loading of 23 nN. The area under each force–distance curve between each drug probe and canister surface was integrated to obtain separation energy values. The adhesion energy between each canister surface and drug was repeated in triplicate (n = 3).
A drawback in the use of the colloid probe technique in adhesion studies is the fact that the actual size and geometry of the AFM probe is unknown and, therefore cannot be normalized. Consequently, variability in probe contact radius geometry is to be expected. Accordingly, to avoid significant variations in contact area between each probe and the model canister surface, the same drug probes (3 for each drug) were used for all the measurements and a great deal of care and attention were taken to maintain the integrity of the colloid probe throughout the study. This allows the inter-relationship between each probe and the various substrate surfaces to be elucidated.
Statistical Analysis
The statistical analysis of the energy of interaction between individual colloid probes and the respective substrates were compared using one-way analysis of variance. Levels of significance were based on 95% probability values (ANOVA, Fisher's pairwise p < 0.05).
RESULTS AND DISCUSSION
Characterization of Surface Morphology
To fully understand the relationship between surface free energy (γ), work of adhesion (W) and drug–surface interactions in a mHFA system, canister surfaces were first investigated using tapping mode AFM and SEM. Representative AFM surface plots of the ALU, AN-ALU, PFA, FEP-PES and PTFE and relative scanning electron micrographs of the different canister surfaces are shown in .
FIG. 1 Taping mode height images (right) and SEM microphotographs (left) of ALU, AN-ALU, PFA, FEP-PES, and PTFE canister surfaces.
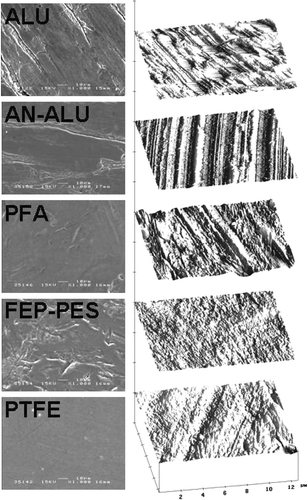
In general, SEM inspection of the canisters surface morphology suggested ALU and AN-ALU to have relatively planar surfaces containing linear striations similar to that reported previously (CitationYoung et al. 2003). In comparison, PTFE had a homogeneous surface, while both PFA and FEP-PES presented creased, lined surfaces, probably due to the non-uniformity of the applied coating. In addition, the height data obtained by AFM was processed to produce root mean squared roughness values. Analysis of the RRMS values over multiple 5 μ m × 5 μ m areas of each surface suggested statistical differences (ANOVA p < 0.05) (n = 25). The surface roughness measurements are shown in .
TABLE 2 Analysis of the root mean square surface roughness (RRMS) values of each canister surface materials (n = 25, ± Std)
The rank order of the surface roughness for the canister surfaces were as follows: PFA > PTFE > ALU > AN-ALU > FEP-PES.
Variations in surface roughness between canister surfaces were expected. It has been previously reported that a roughness value of less than 100 nm will not have a significant influence on surface energy measurements (CitationBuckton et al. 1995). The critical effects of surface rugosity and more specifically surface asperities on particle adhesion is still an issue of debate.
Determination of Contact Angle and Relative Surface Energy Values for Drug Compacts and Canister Surface Surfaces
The surface energy components (γLW, dispersive and γ+, γ− polar) and total surface free energy (γTOT) of the canister surfaces and drug compacts were determined using the contact angle method, and are summarized in .
TABLE 3 Surface energy values for canister surface material and drug compacts calculated by contact angle (mJ · m−2) (n = 3, ± STD; Surface energy values of drug compacts previously published in Traini et al. 2005)
The dispersive γLW surface energy parameter for the three drug materials suggested no significant difference.
Greater differences were found in the electron-donor (γ−) and electron-acceptor (γ+) surface energy parameters. Analysis of the polar components indicates a difference in rank order for γ+: salbutamol > budesonide > formoterol and for γ−: formoterol > budesonide > salbutamol. Generally, the total specific surface energy values γTot showed salbutamol to have the highest value, while budesonide and formoterol presented lower values that were not significantly different.
When comparing canister surfaces, significant differences in the dispersive γLW surface energy were observed. Analysis of the data indicated a rank order for dispersive γLW surface energy parameter of: AN-ALU > ALU > FEP-PES > PTFE > PFA, In addition, ALU and AN-ALU had γLW values were approximately double those of the polymer coated canisters. A reasonable explanation for such difference in surface energy values may be related to the chemical nature of the coating material used. It is generally agreed that surface free energy decreases with increased fluorine content (CitationZisman 1963).
Analysis of the polar components of the surface free energy indicated a difference in rank order for γ +: PTFE > ALU > AN-ALU > PFA > FEP-PES, and for γ−: AN-ALU > ALU > PFA > FEP-PES > PTFE. Overall, the total specific surface energy values γTot showed a ranking decrease of: AN-ALU > ALU > FEP-PEF > PTFE > PFA. As expected, greater values of γTot were observed for the non-polymer coated canister surfaces.
Determination of the Theoretical Work of Adhesion of Different Polymer Coated and Non-Coated Aluminium Canister Walls
The experimentally calculated surface energies of the drugs and can surfaces were modelled using the SCA in non aqueous media to give theoretical values for the work of adhesion between each drug and canister surface (CitationTraini et al. 2005). The dispersive component of the thermodynamic work of adhesion (of the three drug particulates against the respective canister surfaces) together with the acid/base, and total component, in the presence of mHFA are presented in .
TABLE 4 Theoretical thermodynamic work of adhesion (mJ · m−2) (Δ G123) calculated using the SCA model between the drug materials and canister surfaces
Surface tension parameters for mHFA were: γLW = 13.59, γ+ = 0 and γ− = 0 mJ/m2. Comparison of the LW and AB work of adhesion, for each drug with canister surfaces, suggested adhesive forces are controlled by the combination of dispersive and polar interactions. This is exemplified when comparing the rank order in predicted adhesion using dispersive or polar and dispersive calculations. For example, calculation of the work of adhesion for salbutamol with can surfaces shows a rank order for dispersive forces as: AN-ALU > ALU > FEP-PES > PTFE > PFA. However, taking into consideration the acidic and basic component of the free energy, the rank order is: AN-ALU > ALU > PTFE > PFA > FEP-PES. Clearly, the potential for such modification in total surface free energy may significantly influence the particle adhesion in and subsequent formulation performance.
Analysis of budesonide and formoterol theoretical work of adhesion (), suggested similar changes in rank order, when combining dispersive and polar components. With the aim of quantifying the relative contribution of the various surface free energy components (of both substrate surfaces and drugs) to the thermodynamic work of adhesion of the interacting materials, via the SCA theoretical approach, the values were directly compared with separation energy measurements using in situ AFM.
In Situ Atomic Force Adhesion Measurements of Polymer Coated and Non-Coated Aluminium Canister Walls
Measurement of adhesive forces and separation energies between individual particles and substrate surfaces by in situ AFM, and the influence of various interactive forces on particle adhesion, have been reported previously (CitationAshayer et al. 2003; CitationYoung et al. 2003; CitationHooton et al. 2004; CitationYoung et al. 2004). However, a direct link between the macroscopic measurements of surface energy, theoretical predictions of the thermodynamic interfacial interactions and particle adhesion have not been investigated to such an extent (CitationBegat et al. 2004; CitationTraini et al. 2005).
An obvious issue to consider when comparing the adhesion of a drug particle on a series of can substrates is the variation in roughness. Previous studies, have suggested an increase in surface roughness gives rise to a log-normal distribution in adhesion measurements (CitationPrice et al. 2002; CitationYoung et al. 2004) in comparison to normally distributed measurements for highly smooth substrate surfaces (CitationPrice et al. 2002; CitationBuckton et al. 1995).
Frequency distribution histogram measurements of the separation energy between the colloid drug probes and canister surfaces each exhibited a log-normal distribution. These data suggest therefore that the surface roughness of the canister material does directly influence the adhesion measurements, and its distribution, under in situ conditions (CitationPrice et al. 2002; CitationYoung et al. 2004). As previously stated, 3 drug probes were utilised to measure the interactions with all substrates to allow rank correlation of the AFM measurements. It is interesting to note, however, that surface energy dominated the system and the effects of roughness did not play such a significant role.
Median separation energy values (e0.5) were calculated for each individual specific log-normal distribution. A summary table of the AFM measurements for salbutamol, budesonide, and formoterol are presented in , , and , respectively.
TABLE 5 AFM median separation energy values for salbutamol drug probes on canister surfaces (Tips 1–3)
TABLE 6 AFM median separation energy values for budesonide drug probes on canister surfaces (Tips 1–3)
TABLE 7 AFM median separation energy values for formoterol drug probes on canister surfaces (Tips 1–3)
The AFM data for salbutamol drug probes suggested that the force of adhesion followed the following decreasing rank order of: AN-ALU > ALU > PTFE > FEP-PES > PFA for all three probes. For budesonide drug probe, the rank order was: AN-ALU > ALU > PTFE > PFA > FEP-PES. Finally, for formoterol drug probe, the rank order was: AN-ALU > PTFE > ALU > PFA > FEP-PES.
Comparisons Between Thermodynamic Work of Adhesion and AFM Measurements
From Equation (Equation2), it is intuitive to derive that the thermodynamic work of adhesion is directly proportional to the force of adhesion. Thus by plotting the measured separation energy against the theoretical surface energy/work of adhesion, it may, therefore, become possible to correlate theoretical measurements of the interfacial free energy of interaction with experimental measurements of the adhesive interaction.
For comparison, the relationship between the surface free energy calculated by CA (LW-CA), the theoretical work of adhesion (using the dispersive surface free energy only) and the total SCA theoretical work of adhesion (using dispersive and polar contributions of the surface free energy) with AFM separation energy measurements were plotted for salbutamol, budesonide, and formoterol in , , and , respectively.
FIG. 2 Plot of salbutamol drug probe AFM separation energy against γLW dispersive surface free energy calculated by CA (A), γLW dispersive work of adhesion value (B), and Total work of adhesion (Wad Tot) (C), calculated using the SCA model the for the 5 canister materials. (n = 3 drug probes, • = Tip 1; ○ = Tip 2; ▾ = Tip 3.)
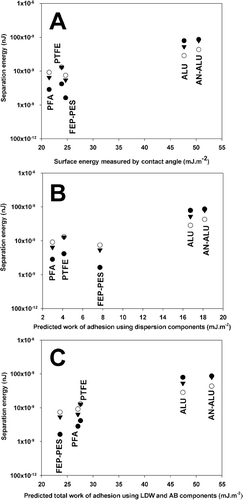
FIG. 3 Plot of budesonide drug probe AFM separation energy against γLW dispersive surface free energy calculated by CA (A), γLW dispersive work of adhesion value (B), and Total work of adhesion (Wad Tot) (C), calculated using the SCA modelthe for the 5 canister materials. (n = 3 drug probes, • = Tip 1; ○ = Tip 2; ▾ = Tip 3.)
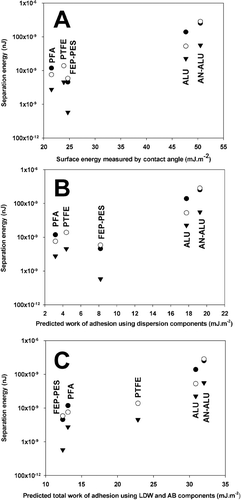
FIG. 4 Plot of formoterol separation energy against γLW dispersive surface free energy calculated by CA (A), γLW dispersive work of adhesion value (B), and Total work of adhesion (Wad Tot) (C), calculated using the SCA model the for the 5 canister materials. (n = 3 drug probes, • = Tip 1; ○ = Tip 2; ▾ = Tip 3.)
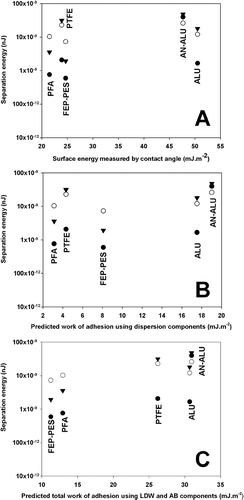
Comparison of the AFM adhesion measurements with theoretical values for salbutamol indicated a direct relationship with surface free energy measured using the CA method (with a correlation coefficient of the linear relationship equal to R2 = 0.7952, R2 obtained from the mean value of the three probes). However, when considering the theoretical work of adhesion (calculated using the SCA method), dispersive components resulted in a less significant relationship (R2 = 0.7020). In comparison, when assessing the relationship between adhesion measurement and the total theoretical adhesion values a more positive relationship was observed (R2 = 0.9260). Such observations suggest the SCA results in an improved relationship between theory and experimental values, when considering interactions between drug particles and different canister materials.
For example, when considering interactions between salbutamol sulphate and energetically similar polymers, FEP-PES presents the lowest adhesion measurements. Such observations, however, are not equivalent to the rank order of polymer surface energy (LW), where PFA < PTFE < FEP-PES. In comparison, when utilising the SCA, the rank order of predicted adhesion and measured separation energy become analogous. Similar results were observed for budesonide and formoterol interactions.
In general, the linear relationship between theoretical and measured adhesion was observed to improve when comparing theoretical surface free energy and SCA values. Subsequently, when comparing these results with the total theoretical SCA work of adhesion (in mHFA), a similar rank order to that of AFM was observed. Such observations highlight the importance of including the dispersive and AB components of the drug polymer and non-aqueous media when considering formulation development. These latest findings were unforeseen and corroborated a still highly debated opinion that although non-aqueous, partially fluorinated liquids are by no means apolar. Even for budesonide and formoterol the correlation coefficient was observed to increase from R2 = 0.6947 to 0.7133 for budesonide and from 0.6768 to 0.8012 for Formoterol giving an indication of the improved relationship between theory (derived by SCA) and experimental values (measured by AFM and CA).
CONCLUSIONS
The present study suggests that it may be possible to predict the interactions in pMDIs systems from thermodynamic considerations of the surface free energy measurement of the interacting materials, derived using the theoretical surface component approach (SCA). This investigation provided further experimental evidence for the existence of non-DLVO type of interactions in pMDI suspension based colloidal systems. Whilst the classical DLVO theory could not satisfactorily illustrate the above phenomena, the inclusion of Lewis AB forces in the SCA model, correlated well with the observed drug particulates behavior different substrate interactions. In addition, this investigation demonstrates that partially fluorinated liquids are not non-polar, and their weak polarity plays an important role in dictating interactions in this kind of pMDI system. Although good correlations were observed between SCA and particle adhesion, it is important to note topography will influence direct comparison. The link between roughness SCA and adhesion is currently under investigation by the authors.
REFERENCES
- Ashayer , R. , Luckham , P. F. , Manimaaran , S. and Rogueda , P. 2003 . Investigation of the Molecular Interactions in a pMDI Formulation by Atomic Force Microscopy . Eur. J. Pharm. Sci. , 21 ( 4 ) : 533 – 543 . [CSA]
- Ashurst , G. , Herman , C. S. , Li , L. and Riebe , M. T. Metered Dose Inhaler for Salmeterol . Patent Number: 6, 143, 277 . 1996 .
- Begat , P. , Morton , D. A. V. , Staniforth , J. N. and Price , R. 2004 . The Cohesive-Adhesive Balances in Dry Powder Inhaler Formulations I: Direct Quantification by Atomic Force Microscopy . Pharm. Res. , 21 ( 9 ) : 1591 – 1597 . [PUBMED] [INFOTRIEVE] [CROSSREF] [CSA]
- Binnig , G. and Quate , C. F. 1986 . Atomic Force Microscope . Phys. Rev. Lett , 56 : 930 – 933 . [PUBMED] [INFOTRIEVE] [CROSSREF] [CSA]
- Blondino , F. E. and Byron , P. R. 1998 . Surfactant Dissolution and Water Solubilisation in Chlorine-Free Liquefied Gas Propellants . Drug Devel. Indus. Pharm , 24 : 935 – 945 . [CSA]
- Buckton , G. , Darcy , P. and McCarthy , D. 1995 . The extent of errors associated with contact angles 3. The influence of surface roughness effects on angles measured using a Wilhelmy plate technique for powders . Colloids and Surfaces A: Physicochem. Engineer. Asp , 95 : 27 – 35 . [CROSSREF] [CSA]
- Chibowski , E. , Bolivar , M. and Gonzalez Caballero , F. 1992 . Studies on the Surface Free Energy Components of Nitrofurantoin . J. Colloid and Interface Sci. , 154 ( 2 ) : 400 – 410 . [CROSSREF] [CSA]
- Derjaguin , B. V. and Landau , L. D. 1941 . Theory of the Stability of Strongly Charged Lyophobic Sols and of the Adhesion of Strongly Charged Particles in Solutions of Electrolytes . Acta Physicochimica U.S.S.R. , 14 : 663 [CSA]
- Derjaguin , B. V. , Müller , V. M. and Toporov , Y. P. 1975 . Effect of Contact Deformations on the Adhesion of Particles . J. Colloid and Interface Sci , 53 : 314 – 326 . [CROSSREF] [CSA]
- Ducker , W. A. , Senden , T. J. and Pashley , R. M. 1991 . Direct Measurement of Colloidal Forces Using an Atomic Force Microscope . Nature , 353 : 239 – 241 . [CROSSREF] [CSA]
- Fowkes , F. M. 1964 . Attractive Forces at Interfaces . Indust. Engineer. Chem , 56 : 40 – 52 . [CROSSREF] [CSA]
- Fowkes , F. M. 1983 . “ Physico-Chemical Aspects of Polymer Surfaces ” . Edited by: Mittal , K. L. Vol. 2 , p. 583 New York : Plenum .
- Goebel , A. and Lunkenheimer , K. 1997 . Interfacial Tension of the Water/n-alkane Interface . Langmuir , 13 ( 2 ) : 369 – 372 . [CROSSREF] [CSA]
- Good , R. J. 1992 . Contact-Angle, Wetting, and Adhesion—A Critical-Review . J. Adhes. Sci. Technol , 6 : 1269 – 1302 . [CSA]
- Good , R. J. and Girifalco , L. A. 1960 . A Theory for Estimation of Surface and Interfacial Energies. III. Estimation of Surface Energies of Solids from Contact Angle Data . J. Phys. Chem , 64 : 561 – 565 . [CSA]
- Good , R. J. and Stromberg , R. R. 1979 . Surface and Colloid Science , New York : Plenum Press .
- Hertz , H. 1882 . On the Contact of Elastic Solids . J. Reine Angew. Math , 92 : 156 – 171 . [CSA]
- Hooton , J. C. , German , C. S. , Allen , S. , Davies , M. C. , Roberts , C. J. , Tendler , S. J. B. and Williams , P. M. 2004 . An Atomic Force Microscopy Study of the Effect of Nanoscale Contact Geometry and Surface Chemistry on the Adhesion of Pharmaceutical Particles . Pharma. Res. , 21 ( 6 ) : 953 – 961 . [CROSSREF] [CSA]
- Johnson , K. , Kendall , K. L. and Roberts , A. D. 1971 . Surface Energy and the Contact of Elastic Solids . Proc. Royal Soc. London. A , 324 : 301 – 313 . [CSA]
- Kitahara , A. 1974 . Zeta Potential in Non-Aqueous Media and its Effect on Dispersion Stability . Prog. Organic Coat , 2 : 81 – 98 . [CROSSREF] [CSA]
- Michael , Y. , Snowden , M. J. , Chowdhry , B. Z. , Ashurst , I. C. , Davies-Cutting , C. J. and Ripley , T. 2001 . Characterisation of the Aggregation Behaviour in a Salmeterol and Fluticasone Propionate Inhalation Aerosol System . Int. J. Pharma , 221 : 165 – 174 . [CROSSREF] [CSA]
- Miller , N. C. The Effects of Water in Inhalation Suspension Aerosol Formulations . Paper presented at the Respiratory Drug Delivery . Edited by: P. , R. B. pp. pp. 249 – 257 . Boca Raton, FL : CRC Press .
- Parson , G. E. , Buckton , G. and Chatham , S. M. 1992 . The Use of Surface Energy and Polarity Determinations to Predict Physical Stability of Non-Polar, Non Aqueous Suspension . Int. J. Pharma , 83 : 163 – 170 . [CROSSREF] [CSA]
- Podczeck , F. , Newton , J. M. and James , M. B. 1996 . The Adhesion Force of Micronised Salmeterol Xinafoate Particles to Pharmaceutically Relevant Surface Materials . J. Phys. D: Applied Physi , 29 : 1878 – 1884 . [CROSSREF] [CSA]
- Price , R. , Young , P. M. , Edge , S. and Staniforth , J. N. 2002 . The Influence of Relative Humidity on Particulate Interactions in Carrier-Based Dry Powder Inhaler Formulations . Int. J. Pharma. , 246 ( 1–2 ) : 47 – 59 . [CROSSREF] [CSA]
- Pugh , R. J. , Matsunaga , T. and Fowkes , F. M. 1983 . The Dispersability and Stability of Carbon Black in Media of Low Dielectric Constant. I: Electrostatic and Steric Contributions to Colloidal Stability . Colloids and Surfaces , 7 : 183 – 207 . [CROSSREF] [CSA]
- Rogueda , P. G. A. 2003 . HPFP, a Model Propellant for pMDIs . Drug Devel. Indust. Pharm , 29 : 39 – 49 . [CROSSREF] [CSA]
- Rogueda , P. G. A. 2003 . Quantification of the Interactions Between Micronised Formoterol and a Selection of Surfaces Found in a Pressurised Metered Dose Inhaler (pMDI) , Salt Lake City, , USA : AAPS .
- Rubio-Hernandez , F. J. 1999 . Is DLVO Theory Valid for Non-Aqueous Suspensions? . J. Non-Equilibrium Thermodynam , 24 : 75 – 79 . [CROSSREF] [CSA]
- Smyth , H. D. C. 2003 . The Influence of Formulation Variables on the Performances of Alternative Propellant-Driven Metered Dose Inhalers . Advance Drug Delivery Rev , 55 : 807 – 828 . [CROSSREF] [CSA]
- Tiwari , D. , Goldman , D. , Dixit , S. , Malick , W. A. and Madan , P. L. 1998 . Compatibility Evaluation of Metered-Dose Inhaler Valve Elastomers with Tetrafluoroethane (P134a), a Non-CFC Propellant . Drug Devel. Indus. Pharm. , 24 ( 4 ) : 345 – 352 . [CSA]
- Traini , D. , Rogueda , P. , Young , P. M. and Price , R. 2005 . Surface Energy and Interparticle Forces Correlations in Model pMDI Formulations . Pharma. Res. , 22 ( 5 ) : 816 – 825 . [CROSSREF] [CSA]
- Van Oss , C. J. , Chaudhury , M. K. and Good , R. J. 1988 . Interfacial Lifshltz-Van Der Waals and Polar Interactions in Macroscopic Systems . Chem. Rev , 88 : 927 – 941 . [CROSSREF] [CSA]
- Van Oss , C. J. 1994 . Interfacial Forces in Aqueous Media , New York : Marcel Dekker .
- Verwey , E. J. W. and Overbeek , J. T. G. 1948 . Theory of the Stability of Lyophobic Colloids , Amsterdam : Elsevier Science Publishers .
- Vervaet , C. and Byron , P. R. 1999 . Drug Surfactant Propellant Interactions in HFA Formulations . Int. J. Pharma , 186 : 13 – 30 . [CROSSREF] [CSA]
- Young , S. A. and Buckton , G. 1990 . Particle Growth in Aqueous Suspensions: the Influence of Surface Energy and Polarity . Int. J. Pharma , 60 : 235 – 241 . [CROSSREF] [CSA]
- Young , P. , Price , R. , Lewis , D. , Edge , S. and Traini , D. 2003 . Under Pressure: Predicting Pressurized Metered Dose Inhaler Interactions Using the Atomic Force Microscope . J. Colloid and Interface Sci. , : 298 – 302 . [CROSSREF] [CSA]
- Young , P. M. , Price , R. , Tobyn , M. J. , Buttrum , M. and Dey , F. 2004 . The Influence of Relative Humidity on the Cohesion Properties of Micronised Drugs Used in Inhalation Therapy . J. Pharma. Sci. , 93 ( 3 ) : 753 – 761 . [CROSSREF] [CSA]
- Wyatt , D. A. and Vincent , B. 1989 . Electrical Effects in Non-Aqueous Systems . J. Biopharma. Sci , 3 : 27 – 31 . [CSA]
- Zisman , W. A. 1963 . Influence of Constitution on Adhesion . Indust. Engineer. Chem , 55 : 10 – 38 . [CROSSREF] [CSA]