Abstract
The health risks to workers in the Florida phosphate industry resulting from chronic inhalation of radionuclide-containing aerosols have not been adequately addressed. The present study establishes a database of information on the particle size distribution, density, shape, chemical composition, and radioactivity concentration for six phosphate facilities in the northern and central regions of the state. A seven-stage cascade impactor was employed to sample aerosols at various processing areas in these plants. Aerosol mass loadings are lowest mainly at shipping areas where they are approximately one order of magnitude less than at granulator areas within equivalent particle size intervals. Aerosol mass concentrations increase as the aerosol size increases for the majority of the plants and operational areas. The aerosol loading at each area varies widely depending on plant, and the variance is largest at the storage areas due to the variability of mechanical operations and patterns of building ventilation. The density of bulk dry product, settled dust, and airborne particles are between 1.6 to 1.7 g/cm 3 . Under electron microscopy, the particles appear as spheroids or rough spherical fragments across all plants, work areas, and sampled size intervals. The main elemental components of large-sized and medium-sized aerosols are similar to those found in the bulk dry product. For small-sized aerosols (0.2–0.4 μ m), the fraction of phosphorus is very small in comparison to elemental impurities such as silicon and sulfur. The 238 U decay series was found in both bulk dry product, settled dust, and airborne particles collected via high-volume samplers. The 238 U, 226 Ra, and 210 Pb radioactivity concentrations in bulk dry product and settled dust from central Florida range from 63–112 pCi/g, 0.8–1.5 pCi/g, and 7–9 pCi/g, respectively. No significant differences in the radioactivity concentrations of 238 U and 226 Ra were found between dry product, settled dust, and sampled aerosols. However, 210 Pb is highly concentrated in aerosols up to 25–87 pCi/g, most likely due to the deposition of ambient airborne radon decay products on workplace aerosols. The database of worker aerosol physicochemical characteristics established in this study for the Florida phosphate industry can be directly used for more realistic assessments of worker inhalation dose, thus providing a more firm basis for assessing the adequacy of existing respiratory and other radiological protection policies.
INTRODUCTION
The phosphate industry produces fertilizer, animal feed, and phosphoric acid using phosphate ore, which contains Naturally Occurring Radioactive Materials or NORM. The industrial practices that utilize these natural resources generate aerosols in the workplace that concentrate NORM to a degree. Risks to workers from aerosol emissions from the phosphate industry include radiation exposures resulting from aerosol inhalation and external gamma-ray exposure. Concentrated radionuclides as a result of human industrial practice are referred to as Technologically Enhanced Naturally Occurring Radioactive Material or TENORM. TENORM issues are mainly focused on waste streams from industrial processes and phosphate fertilizers owing to their widespread use (CitationEPA 1991). The majority of published studies on TENORM in the phosphate industry to date have been conducted for the purpose of environmental protection (CitationBoothe 1977; CitationBurnett and Elzerman 2001; CitationHaridasan et al. 2002; CitationHull and Burnett 1996; CitationParidaens and Vanmarcke 2001).
A comprehensive integrated study on worker exposures from TENORM in the Florida phosphate industry was carried out by CitationBirky et al. (1998). That study collected extensive data on worker exposures at different processing areas including mines and chemical plants. External exposure from airborne and settled particles, as well as internal exposure due to aerosol inhalation and digestion, was assessed for workers in different job classifications. The study demonstrated that aerosol inhalation was a main source of radiation exposure to workers. The study employed high-volume samplers, settled down dust, and effective dose coefficients as given in Publication 61 of the International Commission on Radiological Protection (ICRP) (CitationICRP 1994a). The authors pointed out that in their study “no adjustment for particle size” was made and that “the inhalation doses were conservative” as they tended to be “greater than actual doses.” Other recent studies of note include that by CitationLipsztein et al. (2001) and CitationGafvert et al. (2001). In these studies, detailed information on the particle size distribution and other aerosol characteristics were not available for more accurate and potentially less conservative assessments of worker inhalation dose.
The radioactivity concentrations of phosphate materials, including matrix, products, by-products, and waste have been the subject of many previous studies (CitationBirky et al. 1998; CitationBurnett et al. 1995; CitationEPA 1977, Citation1978; CitationGuimond 1978; CitationGuimond and Windham 1975; CitationHull and Burnett 1996; CitationLaiche and Scott 1991; CitationLardinoye et al. 1982; CitationOwen and Hyder 1980; CitationRoessler et al. 1979; CitationWarren et al. 2001). The principal radionuclides contained in phosphate material are of the 238U decay series. It has been noted that these radionuclides are initially in secular equilibrium within the matrix, yet are localized non-uniformly within product or by-product materials after various process steps.
In 1994, the ICRP issued Publication 66, Human Respiratory Tract Model (HRTM) for Radiological Protection (CitationICRP 1994b). The ICRP 66 HRTM recommends that whenever possible, site-specific information on aerosol physicochemical properties should be measured and then used in the worker dose assessment (CitationICRP 1994b). In the absence of aerosol information, it is assumed that radioactive materials are distributed log-normally in particle size with a geometric mean given by the Activity Median Aerodynamic Diameter (AMAD) and an associated Geometric Standard Deviation (GSD). Although the ICRP recommends using specific default values for routine calculations, these aerosol parameters vary widely in reality. For example, a survey study by CitationDorrian and Bailey (1995) indicated that measured values of AMAD are widely distributed and range from as small as 0.12 μ m to as large as 25 μ m in specific workplace environments. Consequently, use of default values rather than site-specific data can potentially skew dose estimates to unrealistic values in individual exposure cases.
The objectives of the present study were to make direct measurements of the particle size distribution, mass density, shape, chemical composition, and radioactivity concentrations at worker environments within several facilities in the central and north Florida phosphate industry. The database of particle size and physiochemical properties thus established is needed for more worker-specific assessments of radiation inhalation exposures devoid of potentially conservative default assumptions. The data collected will also provide important information to the phosphate industry and to state regulators as they assess the need for any changes in established respiratory and other radiological protection policies.
MATERIALS AND METHODS
Particle Size Distribution
depicts the various industrial processes in the Florida phosphate industry. A previous study has shown that areas of dry product manufacture, dry product storage, and shipping have the highest potential for worker radiation exposure (CitationBirky et al. 1998). Final products are generated in dry product manufacturing areas including those of granulation, screening, and drying. The products are loaded directly for shipping, or are moved to a storage warehouse for later shipment. All processes are associated with aerosol-generating activities either from automated mechanical systems or mobile heavy equipment. In this study, air samples were collected at granulator, storage, and shipping areas within six different phosphate processing plants in either the central or northern regions of Florida.
FIG. 1 Series of processes in the Florida phosphate industry. Rectangular and oval elements of the chart represent, respectively, the type of process and the phosphate material at each process.
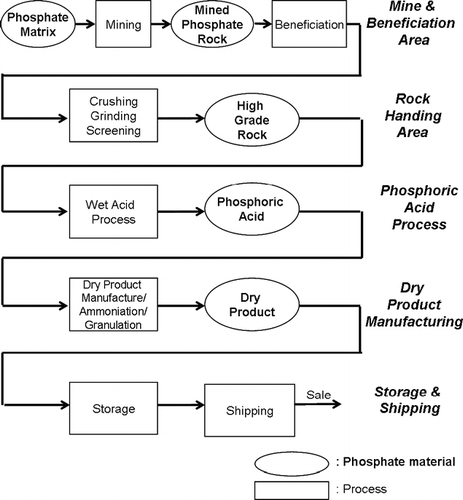
A PM 2.5 dichotomous sampler was employed for pre-sampling studies to estimate particle mass concentrations in the worker environments. These measurements were used in turn to estimate maximum cascade impactor sampling times at each worker area. By so doing, impactor stage overload and subsequent particle-bounce effects could be avoided. A University of Washington (UW) Mark III Cascade Impactor was used to characterize the particle size distribution (CitationMarple 2004; CitationPegnam and Pilat 1992; CitationPilat et al. 1970; CitationPilat and Steig 1983). The UW Mark III impactor consists of seven impactor stages followed by a final collection filter thus partitioning particles into eight different size ranges (CitationPilat 1998). The cutoff size of each stage was calculated by the methods of CitationMarple and Willeke (1976) with a value of 0.49 for the square root of the Stokes number (CitationJohn 1999; CitationMarple and Willeke 1976; CitationRader and Marple 1985; CitationSethi and John 1993). The aerodynamic cutoff sizes were calculated as 21.50, 9.38, 3.57, 1.76, 0.97, 0.51, and 0.26 μ m for the 1st to 7th impactor stages at a flow rate of 15 L/min. The upper particle size limit of the cascade sampler was taken to be 100 μ m following that for the total suspended particulate high-volume air samplers (CitationEPA 1999). The lower limit of particles collected on the final collection filter was taken to be 0.03 μ m, a value typical of those employed in similar studies (CitationDivita et al. 1996; CitationHowell et al. 1998; CitationMarley et al. 2000; CitationWagner and Leith 2001).
A polycarbonate screen membrane film was placed over the cascade impactor substrate to facilitate particle removal as needed for subsequent analyses of particle shape and chemical composition. The samplings were conducted as close as possible to current worker locations, and the height of the inlet nozzle of the cascade impactor was set at a nominal breathing height of 1.5 m. The cascade impactor was operated at a flow rate of 15 L/min for 3 to 27 hours. Duplicate samplings were conducted at all locations. After sampling, impactor substrates containing aerosols were removed from the impactor, desiccated for 24 hours, and then weighed using a microbalance (Satorius, MC210S). Additionally, a PM10 high-volume sampler (Sierra-Andersen, SAUV-10H) was used to collect aerosols with an aerodynamic diameter ≤ 10 μ m as needed for analyses of both particle density and radioactivity content. Samplings without the PM10 orifice were also conducted to collect aerosols at larger sizes (up to 100 μ m).
Particle Density, Shape, and Chemical Composition
The mass density measurements using a pycnometer (Qunatachrome, Ultrapycnometer 1000) were made of (1) bulk dry products (including monoammonium phosphate or MAP, and diammonium phosphate or DAP), (2) settled dust in granulator areas, and (3) aerosols collected by high-volume sampler at granulator areas within the six Florida phosphate processing plants. A limited number of aerosol samples collected by cascade impactor were further analyzed for both particle shape and elemental composition using Scanning Electron Microscopy (SEM) and Energy Dispersive X-ray Spectroscopy (EDXS), respectively. Particles of 3 size ranges from three different areas at two different plants were analyzed for this portion of the study.
Particle Radioactivity
The mass fraction of uranium in phosphate plant dry products ranges from 0.014% to 0.025% (CitationBirky et al. 1998; CitationGuimond 1978; CitationNCRP 1987; CitationOwen and Hyder 1980; CitationRoessler et al. 1979). The small mass of airborne particles collected at each cascade impactor stage resulted in radioactivity loadings that were generally below detectable limits using high-efficiency gamma-ray spectroscopy. Consequently, radioactivity measurements were carried out using bulk dry products, settled dust, and PM10 high-volume aerosol samples at aerodynamic diameters ≤ 10 μ m and ≤ 100 μ m.
A well-type High Purity Germanium (HPGe) detector was employed for radioactive measurement due of its high photon detection efficiency. The gamma-ray spectroscopy system was calibrated with respect to both photon energy and photon detection efficiency using a uranium ore standard from the U.S. Department of Energy New Brunswick Laboratory. Phosphate materials contain radionuclides of the 238U series as well as naturally occurring 40K (CitationBirky et al. 1998). For reference, the entire uranium decay series are depicted in . The contribution of 40K to worker inhalation dose is negligible compared to that due to the various radionuclides in the 238U series. Radioactivity measurements were primarily focused on 238U, 226Ra, and 210Pb as any deviation from natural decay-chain equilibrium would typically occur at the longer-lived daughter radionuclides 226Ra and 210Pb, although disequilibrium for 210Po can also occur.
FIG. 2 The 238U decay series. The numbers in the parenthesis indicate the physical half-life of each radionuclide.
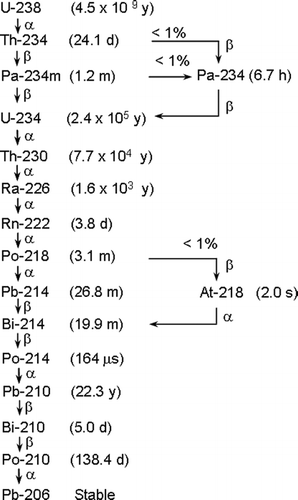
Collected samples were sealed for 30 days in small plastic vials suitable for counting in the HPGe well detector to re-establish secular equilibrium between 226Ra and its 222Rn progeny prior to measurement. In this manner, higher yield gamma-ray photons from 214Pb and 214Bi daughter products can be used to directly infer the radioactivity of the 226Ra parent within the sample. Following the 30-day hold period, the samples were counted for 24 to 48 hours.
Dose Sensitivity to Aerosol Parameters
A sensitivity study was conducted to determine the degree to which various aerosol physicochemical properties contribute to uncertainties in the effective dose to phosphate workers during inhalation exposures of 238U aerosols. Values of the 50-year committed effective dose per unit intake (e.g., effective dose coefficient) to workers under light exertion were computed using the Integrated Modules for Bioassay Analysis (IMBA) code of CitationJames et al. (2003). Worker physiological and activity-related parameters were taken from ICRP 66 HRTM (CitationICRP 1994b). The IMBA code is based upon the ICRP 66 HRTM as well as the more recent elemental biokinetic models published by the ICRP. The program explicitly accounts for the in-growth of decay progeny following inhalation of the 238U parent aerosol particle.
lists the aerosol input parameters considered. Initially, the effective dose coefficient was calculated using ICRP default aerosol parameters. In the ICRP 66 model, the particle size distribution is characterized by a single log-normal distribution with an AMAD of either 5 μ m for workplace exposures or 1 μ m for environmental exposures to members of the general public. In both cases, the distribution is further characterized by a GSD given as a function of the corresponding activity median thermodynamic diameter (AMTD). Other ICRP 66 default parameters include a particle density of 3 g/cm3, a shape factor of 1.5, and a moderate level of solubility (Type M) within the lung fluids following particle deposition. Type M solubility is recommended by the ICRP for all elements except cesium, iodine, and thorium in the absence of site-specific values (CitationICRP 1994b).
TABLE 1 Footnote a ICRP 66 Footnote b HRTM default aerosol parameters and input aerosol parameters for dose sensitivity study
Next, the IMBA code was used to generate 238U effective dose coefficients in which each aerosol parameter was varied over a reasonably broad but realistic span of values. The particle size distribution was allowed to vary from an AMAD of 0.01 μ m to an AMAD of 100 μ m by considering the upper and lower size limits of particles sampled in this study. GSD values were set to unity to provide a narrower particle size distribution and then to the square of the ICRP 66 HRTM default value to show a wider dispersion. In reality, however, GSD values are most likely found between these two values. The particle density was then set to values of either 0.7 or 11 g/cm3, while the particle shape factor was given a value of either 1.0 (spherical) or 2.0 (elongated) (CitationHinds 1998). Last, the particle solubility within the lung fluids was changed from the default Type M absorption class to either Type F (fast) absorption or to Type S (slow) absorption.
RESULTS AND DISCUSSION
Particle Size Distribution
displays aerosol size distributions at granulator, storage, and shipping areas across all six Florida phosphate facilities participating in the study. For clarity, the distributions are depicted as connected scatter plots rather than as histograms. Each data point thus represents the aerosol mass concentration at the geometric mean of the impactor stage. The data points shown are mean values of duplicate samples.
FIG. 3 Aerosol size distribution. Capital letters in legend are plant identifiers. Plant A-a and Plant A-b refer to the same company plant (Plant A), but two different locations within the plant.
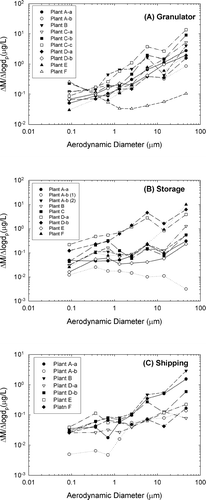
For each worker area, particle size measurements are shown not to follow a normal distributional shape on a logarithmic size scale (i.e., lognormal distribution). Particle mass is increasingly concentrated at larger particle sizes for the majority of the plants and operational areas of the study. Aerosol mass concentrations in the larger size intervals are generally higher at granulator and storage areas, with values dependent upon the operational systems, mechanical activity levels, ventilation, and building structures at the various sampling areas. In general, operational systems in granulator areas include granulators, screeners, dryers, and conveyers that all run during product manufacturing and thus generate aerosols in the work environment. Those in storage areas include conveyors, reclaimers, and heavy equipment such as bobcat tractors or payloaders. Conveyors move products from granulator areas to storage areas or from storage areas to shipping areas for cargo loading. Various types of heavy equipment are used to move products inside storage areas for shipping and they typically generate aerosols to a greater extent than conveyors or reclaimers. Furthermore, ventilation is an important factor in the observed aerosol mass concentration. Dilution of highly concentrated aerosols to the ambient air via opened doors within the storage facility can greatly reduce aerosol mass concentrations.
The gradient of the aerosol mass concentration with aerosol size (slopes in ) is greater in both the granulator areas (except at plant F), and in those storage areas with high levels of operational activity (plants D and F). Within the larger size intervals, aerosol mass concentrations at granulator, storage, and shipping areas vary approximately one order of magnitude. In contrast, variations in concentration within the smallest size intervals generally decrease at all three operational areas.
For granulator areas, aerosol mass concentrations show a similar trend except at plant F where a roughly size-independent distribution is noted. In addition, the concentration variance between plants is less than those seen at storage or shipping areas. Generally, the granulator area is operated mainly under steady-state conditions. Several systems including the granulator, dryer, screen, and conveyor are operated such that all introduce airborne particles to the worker breathing environment. Granulator areas are isolated from ambient air, and thus external conditions such as weather do not influence indoor aerosol mass concentrations. The maximum mass concentration differences among the various plants are 1.5 orders of magnitude at large particle sizes (excluding plant F), and about 1 order of magnitude at smaller particle sizes. The aerosol mass concentrations at plant F are much lower than those at other plants at the larger particle sizes. Unlike the other phosphate plants in this study, Plant F employs a scrubber system, which includes a fan to move particles and fumes through water flowing into plastic packing. The particles and fumes contacting the water are removed from the air-stream. Nonetheless, aerosol mass concentrations at sizes of a few tenths of a micrometer are comparable to those at other plants. In fact, in the smallest particle size range (0.03–0.26 μ m), the aerosol mass concentration at Plant F is noted to be higher than that found at other facilities.
Aerosol mass concentrations at storage areas show several different trends in comparison to those at granulator areas. The variation is wide at a given particle size range. The maximum concentration difference at large particle sizes is more than a factor of 1000. For Plants D and F, aerosols are mainly concentrated at larger sizes, where they achieve magnitudes comparable to those seen at granulator areas. For the other plants, large-sized aerosol mass concentrations are much lower than those seen at their granulator areas.
Air sampling results at storage areas are highly dependent on specific types of work activities and other conditions within the buildings. There are times when only conveyer systems are in operation for the product stack. Alternatively, heavy equipment is sometimes in operation for product loading or moving. The wide differences of activity levels in the warehouses account for the wide variations in aerosol concentrations observed within the storage areas of . During low-level activity, fewer aerosols are generated. Large-sized aerosol concentrations decrease significantly due to rapid settling. If the warehouse is open to ambient air, the mass concentration decrease is more pronounced due to dilution. shows the types of operational systems and ventilation conditions at storage areas during aerosol sampling. The aerosol mass concentration is the highest at Plant D where the warehouse doors were always closed during operation of heavy equipment. For other plants, only conveyor systems were in operation during aerosol sampling, or the doors were open to outside air during heavy equipment operation. For Plants A–B (1), there was no mechanical activity at the time of sampling, and thus the aerosol concentration was extremely low.
TABLE 2 Active operational systems and ventilation conditions during aerosol sampling at the various storage areas
Aerosol mass concentrations are generally the lowest at shipping areas—about one order of magnitude less than found at granulator areas for a given particle size interval. The concentrations show a wide variability depending on the specific plant in question. The maximum mass concentration difference is about a factor of 25 for large particles and about a factor of 10 for small particles. A car-loading system is the only operational device that can generate airborne particles in the shipping area. In addition, the shipping areas are open to the ambient environment where conditions such as wind or rain can strongly influence the aerosol mass concentration. Aerosols generated from the car-loader are then quickly diluted in the atmosphere, and are thus generally lower than at either the storage or granulator areas.
Particle Density, Shape, and Chemical Composition
lists the measured densities of bulk products, settled dust, and sampled aerosols. As there was no discernable density difference between the bulk products MAP and DAP, the data are given only for the different sample types. The densities of bulk products, settled dust, and aerosols sampled under 100 μ m are identical at ∼ 1.7 g cm−3, whereas the densities of aerosols sampled under 10 μ m were found to be slightly less than this value.
TABLE 3 Density of bulk products, settled dusts, and aerosols
Particle shape analysis was conducted on aerosol samples from three different areas within two different chemical processing plants. shows particles of different sizes collected at the granulator area of plant A. Since the particles were compressed during impaction on the filter (e.g., ), it was difficult to differentiate each individual particle from the aggregate particle mass. Single particles can be found at the edges of the impactor stage, and thus images of these edge particles were used for particle shape analysis (e.g., and ). In general, all particles of various sizes, plant facilities, and plant areas appeared as spheroids or as rough spherical fragments. No fiber-like particles were found. Particle shape influences the particle drag force, and is thus characterized by the dynamic shape factor defined as the ratio of the resistance force of the true non-spherical particle to that of a spherical particle of unit density (CitationHinds 1998). Dynamic shape factors for occupational aerosols typically range in value from 1.1 to 1.9 (CitationMercer 1973). In ICRP Publication 66, a reference dynamic shape factor of 1.5 is suggested for occupational radioactive aerosols. CitationJohnson et al. (1987) calculated dynamic shape factors of several non-spherical particles, and noted that they ranged from unity (spherical particles) to values of 1.09 to 1.23 for cylindrical particles having axial ratios of 2 to 5, respectively. Axial ratios of most particles observed in this study did not exceed a value of 2. Consequently, a shape factor of unity can be assumed for the aerosols in the Florida phosphate industry as needed for inhalation dose assessment.
FIG. 4 Shape of particles collected at granulator area in plant A. A: 1st impactor stage particles (scale is 50 μ m), B: 4th impactor stage particles (scale is 10 μ m), and C: 7th impactor stage particles (scale is 1 μ m).
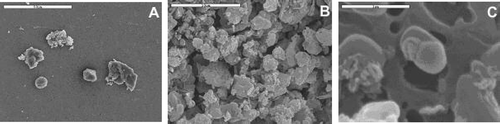
Another benefit of the shape analysis was to verify the cutoff size at each stage of the Mark III cascade impactor. If a particle has a dynamic shape factor of unity, the volume equivalent diameter ranges at the 1st, 4th, and 7th stages are 17.0–79.1 μ m, 1.4–2.8 μ m, and 0.2–0.4 μ m, respectively. The geometric size ranges of particles observed under SEM were found within these calculated values.
shows the chemical composition of dry products which include primarily nitrogen and phosphorus. The dry products also contain other impurities including calcium, iron, aluminum, magnesium, sulfur, and silicon, although the mass fraction of these elements are around 10% (CitationCF 2003a; CitationCF 2003b; CitationMosaic 2003a; CitationMosaic 2003b; CitationPCS 2003a; CitationPCS 2003b). shows EDXS count peaks for sampled airborne particles. Large characteristic X-ray peaks are found at energies corresponding to carbon, oxygen, and phosphorus for large-sized (1st impactor stage) and medium-sized (4th impactor stage) particles. For small-sized (7th impactor stage) particles, the phosphorus peak is significantly depressed, while dominant peaks are noted at X-ray energies corresponding to both sulfur and silicon. The carbon peaks come from the coating material required as part of sample preparation prior to SEM scanning, and can thus be disregarded in the composition analyses.
FIG. 5 Energy Dispersive X-ray Spectroscopy (EDXS) count peak of airborne particles at storage area in plant A. A: large-size particles (1st impactor stage), B: medium-size particles (4th impactor stage), and C: small-size particles (7th impactor stage).
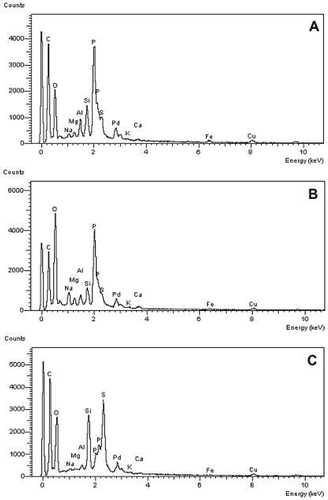
TABLE 4 Footnote a Chemical composition of dry products
Qualitative trends are noted in the data of showing the elemental composition of sampled airborne particles via EDXS for different particle size ranges at plants A and D. The product types in plant A and D are MAP and DAP, respectively. The airborne particles contain various impurities, with silicon and sulfur being the more dominant ones. The particles collected on the 1st and 4th impactor stages contain ∼ 12 to 28% phosphorus. The fraction of silicon and sulfur in these samples is very low in comparison. For small-sized particles, the fraction of phosphorus decreases to between 0.7 and 2.9%, while the fraction of silicon and sulfur increases to ∼ 20% for the majority of samples (except that from the storage area in plant D). The results imply two aerosol sources in this work environment. The first contributes to large-sized aerosols whose main component is the phosphate product. The other contributes to small-sized aerosols whose main components are sulfur and silicon. Nevertheless, the phosphorus fraction of small-sized particles (7th impactor stage) from the storage area at plant D is still more than 20%. As mentioned earlier, the storage area in plant D is operated at a high level of mechanical activity without ventilation, thus showing very high aerosol mass concentrations. The aerosol contribution from phosphate materials due to high levels of mechanical activity exceeds that from sulfur or silicon, and thus the phosphate concentration is still high for this particular facility. Phosphate products are derived from phosphoric acid and ammonium gas, where the phosphoric acid portion contributes the TENORM radioactivity in the product. In contrast, ammonium is not typically associated with natural radioactivity (CitationNCRP 1987). As a result, the radioactivity of the sampled aerosols is thought to be primarily correlated with the amount of phosphorus in the aerosol sample.
TABLE 5 Phosphorus, silicon, and sulfur composition in different size particles. The types of dry products generated in plants A and D are Footnote a MAP and Footnote b DAP, respectively
Particle Radioactivity
shows radioactivity concentrations in bulk products, settled dust, and sampled aerosols. For bulk dry products and settled dust, the radioactivity concentration of 238U is significantly higher than that found for either 226Ra or 210Pb. The radioactivity concentrations of 210Pb are ∼ 5–11 times that of 226Ra. During the chemical process for phosphoric acid generation, a redistribution of the 238U decay series occurs from its originally state of secular equilibrium within the phosphate rock (CitationBirky et al. 1998; CitationBurnett et al. 1995; CitationGuimond and Windham 1975; CitationLaiche and Scott 1991; CitationLardinoye et al. 1982; CitationRoessler et al. 1979). In this redistribution, uranium selectively localizes within product materials, while radium tends to localize in the by-product material known as phosphogypsum. The results given in show a similar trend. For 210Pb, different theories for its localization have been proposed. CitationRoessler (1990) suggested that 210Pb localizes in the bulk dry product, while CitationHorton et al. (1988) indicated that 210Pb localizes in phosphogypsum. In this study, it is seen that 210Pb does not localize in the bulk dry product, but in by-product materials during chemical processing. The radioactivity concentrations of bulk products are in the range of those reported previously (226Ra in DAP and 226Ra and 238U in MAP) or slightly higher than previously reported (238U in MAP) (CitationBirky et al. 1998; CitationGuimond 1978; CitationNCRP 1987; CitationOwen and Hyder 1980; CitationRoessler et al. 1979). For settled dust and sampled aerosols, the radioactivity concentrations of 238U and 226Ra in most samples are within the range of reported data except for 238U in settled DAP dust, which is slightly higher than that previously reported. The radioactivity concentration of 238U in aerosols from northern Florida are lower than those from central Florida, which is likely due to its lower radioactivity concentration in source material or phosphate matrix in that region of the state (CitationRoessler et al. 1979).
TABLE 6 Radioactivity concentrations for 238U, 226Ra, and 210Pb in bulk dry products, settled dust, and sampled aerosols smaller than 10 μ m. Mean and one standard derivation are given for n > 1
Between MAP and DAP, no significant radioactivity differences are found for bulk dry products, settled dust, and sampled aerosols. Although mean 238U radioactivity concentrations in the sampled aerosols are slightly less than those in bulk products and settled dust, the difference is insignificant considering the sample standard deviation. The radioactivity concentrations of 226Ra in aerosols are higher than those in bulk products and settled dusts, but still within one standard deviation.
The radioactivity concentrations of 210Pb in bulk product and settled dust are also similar. However, significantly higher radioactivity concentrations of 210Pb are found in the sampled aerosols. The 210Pb radioactivity of MAP aerosols (86.6 pCi/g) is even higher than the 238U radioactivity concentration (55.1 pCi/g). The increased 210Pb radioactivity is suggested to come from the deposition of ambient airborne radon-decay products in these worker environments. The half-lives of radon progeny, including 218Po, 214Pb, 214Bi, and 214Po, are very short, and thus they decay to 210Pb during aerosol suspension. The attachment of radon progeny to aerosols has been studied extensively (CitationButterweck et al. 1992; CitationKesten et al. 1993; CitationReineking et al. 1992; CitationReineking et al. 1994). These authors report that more than 90% of radon decay products are attached to aerosols in indoor occupational environments, and that the aerosol attached fraction is close to unity.
Dose Sensitivity to Aerosol Parameters
displays the effective dose coefficient of 238U as a function of each aerosol characteristic given in . The single solid circle and solid triangle indicate dose coefficients for ICRP default aerosol values for general public exposures and workplace exposures, respectively. The default effective dose per unit intake for the general public is noted to be 1.53 times that for radiation workers due to the smaller size distribution and thus deeper lung deposition assumed for environmental aerosols within the ICRP 66 inhalation model.
FIG. 6 Effective dose coefficient of 238U for various aerosol parameters. The other parameters except those indicated in the legend follow ICRP 66 human respiratory tract model (HRTM) default values.
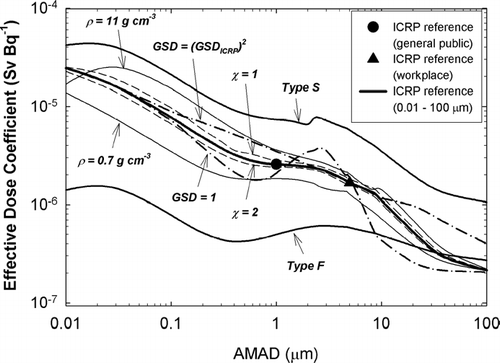
Dose coefficients were further calculated as a function of AMAD with other aerosol parameters held constant at their ICRP 66 default values. In general, the effective dose coefficient decreases with increasing AMAD over the size range 0.01 to 100 μ m. Aerosols with 0.01 and 0.1 μ m AMAD log-normal distributions result in effective dose coefficients that are 9 and 3 times that of a 1 μ m AMAD distribution, respectively. Conversely, effective doses for aerosols with AMADs of 10 and 100 μ m are 1/2 and 1/12 of those for 1 μ m AMAD distributions.
If the aerosol is present as single monodisperse particles (GSD = 1), indicates that the effective dose coefficient decreases with increasing particle size down to a particle size of ∼ 0.6 μ m, and subsequently increases to a local maximum value at a particle size of ∼ 2.5 μ m. After reaching this maximum, the effective dose per unit intake again decreases with increasing particle size. The shape of the effective dose coefficient is related to the regional lung deposition of aerosol particles as a function of particle size. Aerosols deposited deeper within the respiratory tract (e.g., alveolar region) result in longer retention times and thus higher radiation absorbed doses in comparison to those deposited primarily within the upper regions of the respiratory tract (e.g., extra-thoracic region), as the latter is removed to the gastrointestinal (GI) tract faster through mucociliary action. The regional deposition fraction of mono-size aerosols (GSD = 1) shows an initial decrease with particle size, an increase to a maximum value, and then a decrease once again for all sub-regions of respiratory tract, including the extrathoracic airways, the bronchial, bronchiolar, and alveolar-interstitial regions (CitationKim et al. 2005). This local maximum in the effective dose coefficient within the 1 to 10 μ m size region is dampened considerably as the aerosol size distribution widens.
Particle deposition in the respiratory tract depends on two characterizations of the aerosol particles: the aerodynamic diameter d ae and the thermodynamic diameter d th . Their relationship to each other is given the following expression:
The absorption type of an inhaled radionuclide influences the effective dose through changes in its residence time within the respiratory tract. Three different default types—F (fast), M (moderate), and S (slow)—for inhaled materials are given in the ICRP 66 HRTM (CitationICRP 1994b). Type F material is rapidly absorbed to pulmonary blood vessels thus residing in the respiratory tract for short periods of time, while type S material resides in the tract for longer periods due to its slow clearance to blood. Type M material has intermediate rates of blood absorption. Default absorption parameters for type F, M, and S materials, including dissolution fractions and rates, are given in the ICRP 66 HRTM. Deposited particles of Type S 238U remain in the respiratory tract for long periods of time, and thus deliver higher absorbed doses to the lung tissues than do either Type M or Type F 238U particles. Once absorbed into pulmonary blood, the 238U atoms are distributed to the kidney, mineral bone, and other soft tissues of the body. However, their organ dose contributions to the effective dose are small compared to the absorbed dose to the lung tissues. Therefore, Type S, Type M, and Type F materials result in the highest, next highest, and lowest effective dose coefficients for 238U, respectively. Type S 238U aerosols result in an effective dose per unit intake that is 2.9 and 3.4 times that of Type M for 1 μ m AMAD distributions and 5 μ m AMAD distributions, respectively. Conversely, Type F 238U has an effective dose coefficient that is 5.2 (1 μ m AMAD) and 2.9 (5 μ m AMAD) times less than Type M 238U aerosol particles.
This effective dose sensitivity study indicates that the aerosol size distribution and absorption type are the more important input parameters for individual and site-specific dose assessments. Conservative assumptions on the size distribution or absorption type can greatly skew dose assessments to unrealistic values by up to an order of magnitude. The focus of the present study was thus to provide site-specific data on all aerosol characteristics within the Florida phosphate industry as needed for worker dose assessment, with particular emphasis on the particle size distribution. Studies are currently ongoing to measure site-specific values of the lung solubility of Florida phosphate aerosols.
CONCLUSIONS
An accurate assessment of worker risk due to inhalation of TENORM aerosols requires detailed and site-specific knowledge of a range of aerosol properties. In this study, TENORM-containing aerosols in the Florida phosphate industry have been characterized to include the particle size distribution, mass density, shape, chemical composition, and radioactivity concentration. In addition, a sensitivity study was conducted regarding the variations in the effective dose per unit intake of 238U aerosol particles with corresponding variations in each aerosol parameter value. Of particular importance are the aerosol size distribution and lung solubility. The former is reported here, and the later is currently under investigation.
Aerosol size distributions do not follow a lognormal pattern, where the aerosol mass concentration is noted to increase with increasing particle size. The aerosol mass concentration at each plant and area varies widely over 1 to 1.5 orders of magnitude at granulator and shipping areas, and over a factor of a 1000 at storage areas. The shapes of particles appear as spheroids or rough spherical fragments. A high fraction of phosphorus is associated with both large (21–100 μ m) and medium (1.76–3.57 μ m) sized airborne particles. For small-sized airborne particles (0.2–0.4 μ m), sulfur and silicon are found to be dominant over elemental phosphorus. The radioactivity concentrations of 238U are much higher than those of 226Ra in products and particles in their vicinity as 238U selectively localizes on the product from phosphate source material, while 226Ra tends to localize on by-product materials. The radioactivity concentrations of 210Pb are between that of 238U and 226Ra in both bulk dry product and settled dust, and are significantly higher in sampled aerosols due to attachment of radon decay products in the sampled environment. The database established in this study can thus be used for radiation exposure assessments to workers and to members of the general public. In addition, the information is useful to phosphate companies and regulators in their consideration of health protection programs and policies regarding both respiratory and radiological protection.
This work was supported by Grants #00-05-062R and #03-05-064 from the Florida Institute of Phosphate Research (FIPR). This article is dedicated to Professor William Emmett Bolch of the Department of Environmental Engineering Sciences at the University of Florida who passed away on December 26, 2003. The authors greatly appreciate his contributions, support, and devotion to this study and to the field of environmental health physics. The authors gratefully acknowledge Dr. Tony James (ACJ & Associates) and Dr. Alan Birchall (NRPB) for their assistance with the use of IMBA Professional v.3.0 for this research study.
Notes
a International Commission on Radiological Protection;
b Human respiratory tract model;
c Activity median aerodynamic diameter;
d Geometric standard deviation;
e Activity median thermodynamic diameter;
f Moderate absorption into blood;
g Fast absorption into blood;
h Slow absorption into blood.
a Capital letters are plant identifiers. Plant A-a and Plant A-b refer to the same company plant (Plant A), but two different locations within the plant. A-b(1) and A-b(2) are two different sampling sites at the same location.
a Data from material safety data sheet (CitationCF, 2003a; CitationCF, 2003b; CitationMosaic, 2003b; CitationMosaic, 2003a; CitationPCS, 2003b; CitationPCS, 2003a).
a Monoammonium phosphate;
b Diammonium phosphate.
a Data from studies by CitationGuimond (1978), CitationRoessler et al. (1979), CitationOwen and Hyder (1980), CitationNCRP (1987), and CitationBirky et al. (1998).
REFERENCES
- Birky , B. , Tolaymat , T. , Warren , B. , Bolch , W. , Ammons , R. , McNally , T. and Nall , W. 1998 . Evaluation of Exposure to Technologically Enhanced Naturally Occurring Radioactive Materials (TENORM) in the Phosphate Industry , Bartow, FL : Florida Institute of Phosphate Research . FIPR Publication No. 05-046-155.
- Boothe , G. F. 1977 . The Need for Radiation Controls in the Phosphate and Related Industries . Health Phys. , 32 : 285 – 290 . [INFOTRIEVE] [CSA]
- Burnett , W. , Cowart , J. , LaRock , P. and Hull , C. 1995 . Microbiology and Radiochemistry of Phosphogypsum , Bartow, FL : Florida Institute of Phosphate Research . Publication No. 05-035-115
- Burnett , W. C. and Elzerman , A. W. 2001 . Nuclide Migration and the Environmental Radiochemistry of Florida Phosphogypsum . J. Environ. Radioact. , 54 : 27 – 51 . [CSA]
- Butterweck , G. , Porstendorfer , J. , Reineking , A. and Kesten , J. 1992 . Unattached Fraction and the Aerosol Size Distribution of the Radon Progeny in a Natural Cave and Mine Atmospheres . Radiat. Prot. Dosim. , 45 : 167 – 170 . [CSA]
- CF . 2003a . Material Safety Sata Sheet (Diammonium Phosphate) , Long Grove, IL : CF Industries, Inc .
- CF . 2003b . Material Safety Data Sheet (Monoammonium Phosphate) , Long Grove, IL : CF Industries, Inc .
- Divita , F. , Ondov , J. and Suarez , A. 1996 . Size Spectra and Atmospheric Growth of V-Containing Aerosol in Washington, DC . Aerosol Sci. Technol. , 25 : 256 – 273 . [CSA]
- Dorrian , M. and Bailey , M. 1995 . Particle Size Distributions of Radioactive Aerosols Measured in Workplaces . Radiat. Prot. Dosim. , 60 : 119 – 133 . [CSA]
- EPA . 1977 . Radiological Surveys of Idaho Phosphate Ore Processing—The Thermal Process Plant. , Las Vegas, NV : U. S. Environmental Protection Agency . Technical Note ORP/LV-77-3.
- EPA . 1978 . Radiological Surveys of Idaho Phosphate Ore Processing—The Wet Process Plant. , Las Vegas, NV : U.S. Environmental Protection Agency . Technical Note OPR/LV-78-1.
- EPA . 1991 . Diffuse NORM Wastes: Waste Characterization and Risk Assessment. , Washington, DC : U.S. Environmental Protection Agency . Report RAE-9232/1-2.
- EPA . 1999 . Sampling of Ambient Air for Total Suspended Particulate Matter (SPM) and PM10 using High-Volume (HV) Samplers. , Washington, DC : Environmental Protection Agency . Report EPA/625/R-96/101a.
- Gafvert , T. , Holm , E. and Roos , P. 2001 . Radionuclide Fluxes at a Plant Manufacturing Dicalcium Phosphate for Domestic Animals . J. Environ. Radioact. , 54 : 61 – 73 . [INFOTRIEVE] [CSA]
- Guimond , R. 1978 . The Radiological Aspects of Fertilizer Utilization. , pp. 380 – 393 . Washington, DC : U.S. Nuclear Regulatory Agency . NUREG/CP-0001.
- Guimond , R. and Windham , S. 1975 . Radioactivity Distribution in Phosphate Products, By-Products, Effluents, and Wastes. , Washington, DC : U.S. Environmental Protection Agency . Technical Note ORP/CSD-75-3.
- Haridasan , P. P. , Maniyan , C. G. , Pillai , P. M. and Khan , A. H. 2002 . Dissolution Characteristics of 226Ra from Phosphogypsum . J. Environ. Radioact. , 62 : 287 – 294 . [INFOTRIEVE] [CSA]
- Hinds , W. 1998 . Aerosol Technology: Properties, Behavior, and Measurement of Airborne Particles , New York : John Wiley & Sons, Inc .
- Horton , T. , Blanchard , R. and Windham , S. 1988 . A Study of Radon and Airborne Particulates at Phosphogypsum Stacks in Central Florida. , Washington, DC : U.S. Environmental Protection Agency . EPA Report 520/5-88-021.
- Howell , S. , Pszenny , A. A. P. , Quinn , P. and Huebert , B. 1998 . A Field Intercomparison of Three Cascade Impactors . Aerosol Sci. Technol. , 29 : 475 – 492 . [CSA]
- Hull , C. and Burnett , W. 1996 . Radiochemistry of Florida Phosphogypsum . J. Environ. Radioact. , 32 : 213 – 238 . [CSA]
- ICRP . 1994a . Dose Coefficients for Intakes of Radionuclides by Workers (Replacement for ICRP Publication 61). , Oxford, , UK : International Commission on Radiological Protection . ICRP Publication 68.
- ICRP . 1994b . Human Respiratory Tract Model for Radiological Protection. , Oxford : International Commission on Radiological Protection . ICRP Publication 66.
- James , A. , Birchall , A. , Marsh , J. and Puncher , M. 2003 . Users Manual for IMBA Professional Series (Version 3.1). , Richland, WA : ACJ & Associates, Inc .
- John , W. 1999 . A Simple Derivation of the Cutpoint of an Impactor . J. Aerosol Sci. , 30 : 1317 – 1320 . [CSA]
- Johnson , D. , Leith , D and Reist , P . 1987 . Drag on Nonspherical, Orthotropic Aerosol Particles . J. Aerosol Sci. , 18 : 87 – 97 . [CSA]
- Kesten , J. , Butterweck , G. , Postendorfer , J. , Reineking , A. and Heymel , H. 1993 . An Online Alpha-Impactor for Short-Lived Radon Daughters . Aerosol Sci. Technol. , 18 : 156 – 164 . [CSA]
- Kim , K. , Wu , C , Birky , B. and Bolch , W. 2005 . Effective Dose Scaling Factors for Use with Cascade Impactor Sampling Data in TENORM Inhalation Exposures . Health Phys. , 89 : 359 – 374 . [INFOTRIEVE] [CSA]
- Laiche , T. P. and Scott , L. M. 1991 . A Radiological Evaluation of Phosphogypsum . Health Phys. , 60 : 691 – 693 . [INFOTRIEVE] [CSA]
- Lardinoye , M. H. , Weterings , K. and van de Berg , W. B. 1982 . Unexpected 226Ra Build-Up in Wet-Process Phosphoric-Acid Plants . Health Phys. , 42 : 503 – 514 . [INFOTRIEVE] [CSA]
- Lipsztein , J. L. , da Cunha , K. M. , Azeredo , A. M. , Juliao , L. , Santos , M. , Melo , D. R. and Simoes , Filho F. F. 2001 . Exposure of Workers in Mineral Processing Industries in Brazil . J. Environ. Radioact. , 54 : 189 – 199 . [INFOTRIEVE] [CSA]
- Marley , N. , Gaffney , J. , Drayton , P. , Cunningham , M. , Orlandini , K. and Paode , R. 2000 . Measurement of Pb-210, Po-210, and Bi-210 in Size-Fractionated Atmospheric Aerosols: An Estimate of Fine-Aerosol Residence Times . Aerosol Sci. Technol. , 32 : 569 – 583 . [CSA]
- Marple , V. 2004 . History of Impactors—The First 110 Years . Aerosol Sci. Technol. , 38 : 247 – 292 . [CSA]
- Marple , V. and Willeke , K. 1976 . Impactor Design . Atmospheric Environment. , 10 : 891 – 896 . [CSA]
- Mercer , T. 1973 . Aerosol Technology in Hazard Evaluation , New York : Academic Press .
- Mosaic . 2003a . Material Safety Data Sheet (Diammonium Phosphate). , Riverview, FL : Mosaic Company .
- Mosaic . 2003b . Material Safety Data Sheet (Monoammonium Phosphate). , Riverview, FL : Mosaic Company .
- NCRP . 1987 . Radiation Exposure of the U.S. Population from Consumer Products and Miscellaneous Sources. , Bethesda, MD : National Council on Radiation Protection and Measurements . Report No. 95.
- Owen , R. and Hyder , L. 1980 . Technologically Enhanced Naturally Occurring Radioactivity: An Overview. , Oak Ridge, TN : Oak Ridge National Laboratory . ORNL/CF-80/265.
- Paridaens , J. and Vanmarcke , H. 2001 . Radium Concentration of the Banks of the River Laak as a Consequence of the Phosphate Industry in Belgium . J. Environ. Radioact. , 54 : 53 – 60 . [INFOTRIEVE] [CSA]
- PCS . 2003a . Material Safety Data Sheet (Diammonium Phosphate). , Northbrook, IL : PotashCorp .
- PCS . 2003b . Material Safety Data Sheet (Monoammonium Phosphate). , Northbrook, IL : PotashCorp .
- Pegnam , R. and Pilat , M. 1992 . Airborne Particulate Emissions Form a Chromic-Acid Anodizing Process Tank . J. Air. Waste Manag Assoc. , 42 : 303 – 308 . [CSA]
- Pilat , M. 1998 . Operational Manual for the University of Washington Mark III and Mark V Source Test Cascade Impactor. , Seattle, WA : University of Washington .
- Pilat , M. , Ensor , D. and Bosch , J. 1970 . Source Test Cascade Impactor . Atmos. Environ. , 4 : 671 – 674 . [CSA]
- Pilat , M. and Steig , T. 1983 . Size Distribution of Particulate-Emissions from a Pressurized Fluidized-Bed Coal Combustion Facility . Atmos. Environ. , 17 : 2429 – 2433 . [CSA]
- Rader , D. and Marple , V. 1985 . Effect of Ultra-Stokesian Drag and Particle Interception on Impaction Characteristics . Aerosol Sci. Technol. , 4 : 141 – 156 . [CSA]
- Reineking , A. , Butterweck , G. , Kesten , J. and Porstendorfer , J. 1992 . Thoron Gas Concentration and Aerosol Characteristics of Thoron Decay Products . Radiat. Protect. Dosim. , 45 : 353 – 356 . [CSA]
- Reineking , A. , Knutson , E. A. , George , A. C. , Solomon , S. B. , Kesten , J. , Butterweck , G. and Porstendorfer , J. 1994 . Size Distribution of Unattached and Aerosol-Attached Short-Lived Radon Decay Products—Some Results of Intercomparison Measurements . Radiat. Protect. Dosime. , 56 : 113 – 118 . [CSA]
- Roessler , C. 1990 . Control of Radium in Phosphate Mining, Beneficiation, and Chemical Processing. 270 – 279 . Vienna, , Austria International Atomic Energy Agency. IAEA Technical Series No. 310
- Roessler , C. E. , Smith , Z. A. , Bolch , W. E. and Prince , R. J. 1979 . Uranium and Radium-226 in Florida Phosphate Materials . Health Phys. , 37 : 269 – 277 . [INFOTRIEVE] [CSA]
- Sethi , V. and John , W. 1993 . Particle Impaction Patterns from a Circular Jet . Aerosol Sci. Technol. , 18 : 1 – 10 . [CSA]
- Wagner , J. and Leith , D. 2001 . Passive Aerosol Sampler. Part II: Wind Tunnel Experiments . Aerosol Sci. Technol. , 34 : 193 – 201 . [CSA]
- Warren , B. , Harder , G. and Brakefield , K. 2001 . Characterization of Objects Contaminated by Technological Enhanced Naturally Occuring Radioactive Materials (TENORM) within the Phosphate Industry. , Bartow, FL : Florida Institute of Phosphate Research . FIPR Publication No. 05-059-191.