Abstract
An improved method for charging submicron and nano silver particles with uniform charging performance was developed. Monodisperse silver particles were grown into microdroplets through condensation. The aerodynamic diameter and GSD of the condensed droplets were the same regardless of their original diameter. The diameter of the droplets increased from 1.7μm to 2.5 μm as the temperature of the saturator increased from 45°C to 55°C. They were charged by an indirect corona-based charger, in which the ion-generation zone is followed by a particle-charging zone through which the condensed droplets pass. The charges of the droplets were controlled by varying the droplet size, ion concentration, and strength of electric field in the charger. The solvent of the charged droplets was evaporated in an evaporator. The size distribution of the evaporated particles was measured by SMPS spectrometer and compared with their original size distribution. The particles after evaporation were slightly larger than their original particles, due to recondensation. The total charge and number concentration of the particles were measured by aerosol electrometer and CPC, to calculate the average charge. Their electrical mobility distribution was measured by SMPS spectrometer without a neutralizer, to calculate the charge distribution and average charge of the evaporated particles. The results showed the average charges of the particles were similar, regardless of initial diameter and manner of calculation. The charge distributions of the evaporated particles were identical, except for 16.9 nm particles. Ion evaporation phenomenon of particles smaller than 40 nm in diameter was not detected.
INTRODUCTION
Particle-charging techniques have played an important role in aerosol studies, such as the control and measurement of aerosol particles. Much research has focused on improving the charging performance in chargers, with respect to average charge, charging efficiency, and penetration rate. Many chargers based on diffusion charging or field charging have been investigated for these purposes. However, improving the charging performance of ultrafine particles by diffusion charging or field charging has its limits. Particle-charging performance depends greatly on particle diameter, and declines severely as particle diameter decreases; this is especially true for nanoparticles. For instance, when 3 nm particles are charged with a bipolar charger in a Nano-DMA, their average charge is very low (much less than one charge) and the charging efficiency is only 1%, so 99% of such particles are uncharged and wasted during the control and measurement process (CitationReischl et al. 1996).
Several methods have been developed to improve charging performance, for example, minimizing the particle loss in the charger or enhancing the charging efficiency of nanoparticles by adjusting the ion concentration, residence time, and electric field configuration (CitationBüscher et al. 1994; CitationWiedensohler et al. 1994; CitationKruis and Fissan 2001; CitationChen and Pui 1999). Most previous studies have focused only on improving the charging efficiency or penetration rates of nanoparticles in the charger, and not on the average charge. This is because the charge of a particle is highly dependent on the particle diameter.
There has been a certain amount of research on increasing the average charge of nanoparticles. Electrospray and condensation technology have been used to generate highly charged aerosols. Using electrospray, when highly charged micron-sized droplets (or particles) are sprayed in cone-jet mode it is possible to obtain highly charged solid particles (residue particles) after solvent evaporation (CitationLoscertales and Fernandez de la Mora 1995). They founded that when droplets below 40 nm in diameter evaporate, their charges evaporate from their surface, even though the charge density is lower than the Rayleigh limit. This phenomenon is called ion evaporation.
Recently, an enhanced charging method using condensation has been developed. Nano gold and silver particles were grown to micron-sized droplets through condensation, field-charged and dried. After that, highly charged gold and silver nanoparticles were obtained (CitationSuh et al. 2005).
The particle-charging technique using condensation has some advantages over using electrospray. Since the charging procedure is separate from the enlarging procedure, the charge density of droplets could be controlled to prevent the Rayleigh explosion and the ion evaporation.
When controlling and measuring charged particles, the charge characteristics of particles need to be measured over the entire size range. When particles are grown through condensation, the size distribution of the droplets becomes monodisperse, even though the size distribution of the particles before condensation is polydisperse (CitationPark and Lee 2000; CitationPark et al. 2002). It could, therefore, be supposed that the particles are charged uniformly, regardless of particle diameter, thus reducing the effort required to measure the charges of particles.
In the work reported herein, the condensation and evaporation characteristics of silver particles were examined with ethylene glycol vapor and a suitable charger for the condensed droplets was developed. The size distribution of submicron and nano silver particles after condensation and evaporation were measured by using the Aerosizer and the SMPS spectrometer. The average charge of the particles was measured by two manners and each result was compared. The variation of the charge on the droplet by the ion evaporation phenomenon during evaporation was evaluated in the range of 10 nm to 110 nm.
EXPERIMENT
The experimental setup, shown schematically in , consisted of three connecting procedures: condensation, charging, and evaporation. The characteristics of each procedure were evaluated with silver particles. Polydisperse silver particles were generated by the evaporation-condensation method. The particles were classified through a DMA (differential mobility analyzer, model 3071, TSI Inc.) into particles having a monodisperse size distribution based on equivalent electrical mobility, which depends on particle diameter and charge. Silver particles in the range 10 nm to 110 nm were used in the study.
FIG. 1 Experimental setup for measuring (a) the size distribution of classified and evaporated particles and condensed droplets by SMPS spectrometer and Aerosizer (b) and measuring the total charge, number concentration and electrical mobility distribution of highly-charged particles by aerosol electrometer, CPC and SMPS spectrometer.
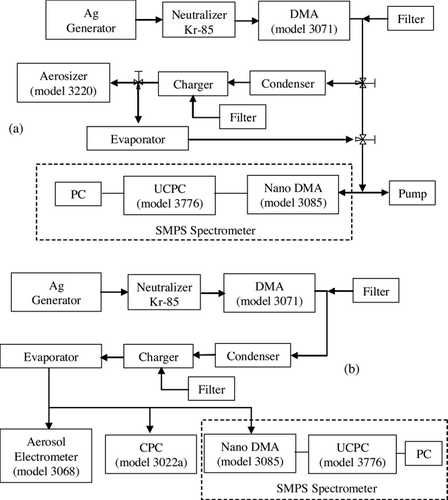
shows a schematic diagram of a condenser, which consisted of a saturator and a cooling pipe. The saturator was partially filled with ethylene glycol liquid and its temperature was kept uniform using a temperature controller. The temperature of the saturator was varied over 45–55°C in increments of 5°C. The particles were saturated with ethylene glycol vapor and grown into micron-sized droplets by condensation while passing through the cooling pipe. The cooling pipe was cooled by four thermoelectric modules and the temperature of the pipe exit was maintained at 10–15°C. The flow rate of the condenser was fixed at 4 lpm.
The condensed droplets were charged by an indirect corona-based charger. A schematic diagram of the charger is shown in . To prevent precipitation or break-up of condensed droplets due to the strong electric field when passing through the charger, a so-called indirect corona charger was developed. It consisted of two zones: an ion-generation zone and a particle-charging zone. In the ion-generation zone, positive ions were generated by corona discharge, some of which were ejected to the particle-charging zone by an external electric field and ion carrier air. In the particle-charging zone, the ejected ions moved to an electrode plate by a weak electric field and some of them adhered to the droplet surface. The corona voltage of the ion-generation zone and the particle-charging zone were varied from 3.5 kV to 5 kV and from 0 kV to 4 kV. The flow rate of the ion carrier air was fixed at 1 lpm.
gives a schematic diagram for measuring the size distribution of classified, condensed and evaporated particles, when the charger was off-state. The size distribution of the condensed droplets was measured by an Aerosizer (TSI model 3220) as varying the temperature of the saturator. The aerodynamic mean diameter and geometric standard deviation (GSD) of the condensed droplets with their initial diameter were calculated.
The solvent of the condensed droplets was evaporated in an evaporator. The evaporator was a stainless steel tube and the flow rate in the evaporator was fixed at 5 lpm, which was identical to the sum of flow rate of the condenser (4 lpm) and the ion carrier air (1 lpm).
The size distribution of classified particles and evaporated particles was measured by SMPS (scanning mobility particle sizer, model 3936, TSI Inc.) spectrometer, which consisted of a Nano DMA (model 3085, TSI Inc.) and UCPC (model 3776, TSI Inc.). The classified and evaporated particles mainly had a charge of +1, so the size distributions of those particles were measured without a neutralizer. The flow rates of the aerosol inlet and sheath air in Nano DMA were fixed at 0.3 and 3 lpm, respectively.
shows a schematic diagram for measuring the charging characteristics of the evaporated particle when the charger was on-state. To evaluate the charging performance of highly-charged silver particles, the electrical mobility distribution, total charge, and number concentration of the evaporated particles were measured by SMPS spectrometer, aerosol electrometer (model 3068, TSI Inc.), and CPC (model 3022a, TSI Inc.).
When using the SMPS spectrometer to measure the charge distribution of highly charged particles, the data obtained should be corrected. The SMPS spectrometer measures the electrical mobility distribution of highly charged particles and converts it to a size distribution of the particles, such as that the particles have one charge. As a result, particles with high charges are considered as smaller particles with a charge of +1 in the SMPS spectrometer and the number concentration is overestimated by considering the charge efficiency in a neutralizer and the counting efficiency in CPC. In this work, a neutralizer was not used and the counting efficiency of the classified particle diameter in UCPC was 100%. So, the number concentration must be changed into the value before the correction, to obtain the exact charge distribution of the highly charged particles.
The ratio of electrical mobility of a highly charged silver particle to that of a classified or evaporated particle with a charge of +1 is the charge on the particle. Hence, the charge distribution and average charge of a particle of known size can easily be calculated from the electrical mobility distribution. However, a classified and evaporated particle is not a perfect monodisperse particle and its GSD is slightly larger than 1, about 1.08. In this work, we assumed, for the sake of convenience, that the classified and evaporated particle is a perfect monodisperse particle of geometric mean diameter. The flow rates of the aerosol inlet and sheath air in Nano DMA were fixed at 1.5 lpm and 15 lpm, respectively.
The average charge of the evaporated particles was also calculated by dividing the total charge by the number concentration. The measured number concentration was corrected by considering the counting efficiency of CPC (model 3022a). The flow rates of the aerosol electrometer and CPC were fixed at 2 lpm and 1.5 lpm, respectively. The results obtained from the two instruments were compared.
RESULTS AND DISCUSSION
The aerodynamic mean diameter and GSD of the condensed droplets are illustrated in . As shown in , particles with different diameters were grown into droplets of a uniform size. The mean diameter of the droplets increased from 1.7 μm to 2.5 μm as the temperature of the saturator increased from 45°C to 55°C. This is because the amount of condensing vapor increases as the temperature of the saturator increases. Since the condensed droplets could evaporate when mixing with ion carrier air in the charger and being measured in the Aerosizer, the measured diameter of a condensed droplet does not yield the exact size of the droplet. However, the result shows that the size distributions of the droplets after the charger are identical with the diameter of the classified particle, even though evaporation occurs. The GSD of the droplets was about 1.07, which means that the condensed droplets were very monodisperse. The difference of GSD with the temperature of the saturator was negligible.
FIG. 4 (a) The aerodynamic mean diameter (b) and GSD of the condensed droplets, as a function of the diameter of classified particles.
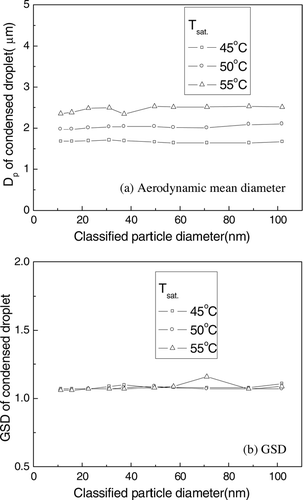
shows the geometric mean diameter and GSD of the evaporated particles as a function of the temperature of an evaporator when the classified particle diameter was 22.4 nm and the temperature of the saturator was 45°C, 50°C, and 55°C, respectively. Since the droplet diameter increases with the temperature of saturator, a higher temperature in the evaporator is necessary to evaporate the solvent of condensed droplets. When the temperature of the evaporator was greater than a critical temperature, the diameter and GSD of particles after evaporation became the same.
FIG. 5 The geometric mean diameter and GSD of evaporated particles with the temperature of the evaporator, when the temperature of the saturator was 45° C, 50° C, and 55° C.
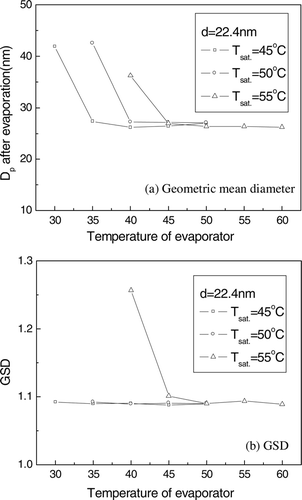
As shown in , the evaporated particles had a very narrow size distribution and their GSD was identical to that of the classified particles. However, the geometric mean diameter of the evaporated particles was larger than that of the original particles; see . If the droplet evaporated incompletely, the error (which is defined as the ratio of the difference in the diameter of evaporated and classified particle to the diameter of classified particle) would decrease as the particle diameter increased. However, as shown in , the error increased with the diameter of particle when the diameter of the particle was less than 60 nm and became similar when it was greater than 60 nm. This suggests that recondensation, rather than incomplete evaporation, occurred.
FIG. 6 (a) Comparison of the geometric mean diameter (b) and GSD of evaporated particles with that of classified particles and (c) the error when the temperature of the saturator was 45° C, 50° C, and 55° C.
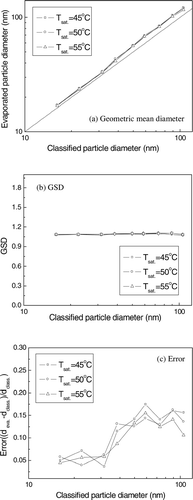
shows the average charge of evaporated silver particles as a function of the charging conditions when the classified particle diameter was 22.4 nm and the temperature of the saturator was 55°C. The total charge and number concentration were measured by using aerosol electrometer and CPC and the average charge was calculated by dividing the total charge by the number concentration. The average charge varied from 30 charges to 60 charges as the corona voltage and external voltage in the charger increased. This is because the ion concentration increases as the corona voltage increases and the electric field is strengthened as the external voltage increases.
FIG. 7 The average charge of particles with corona voltage and external voltage, which was calculated by dividing the total charge by the number concentration (which were measured by using aerosol electrometer and CPC), when the diameter of the classified particle was 22.4 nm and the temperature of the saturator was 55° C.
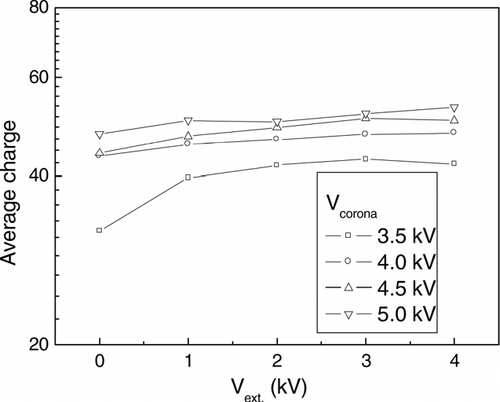
shows the average charge of silver particles as a function of the evaporated particle diameter when the temperature of the saturator was 45°C, 50°C, and 55°C. The average charge increased as the temperature of saturator increased but were uniform with particle diameter, even though the diameter of the particle was less than 40 nm.
FIG. 8 The average charge, which was calculated by dividing the total charge by the number concentration (which were measured by aerosol electrometer and CPC) of the evaporated particles, when the temperature of the saturator was 45° C, 50° C and 55° C.
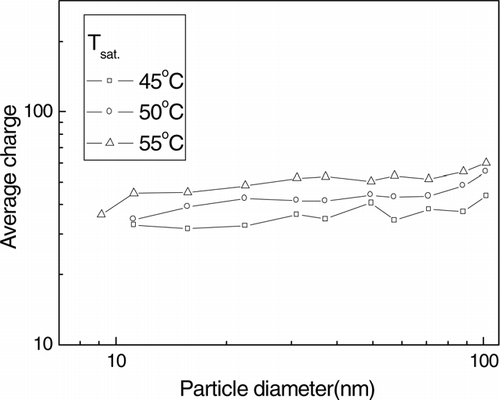
For confirmation, the result was compared with the average charge calculated from the charge distribution which was measured by the SMPS spectrometer. The average charges for several charging conditions calculated by the two methods, when the diameters of the evaporated particle were 16.8 nm, 23.7nm, 33.4 nm, and 51.4 nm, are compared in . The results for the average charges calculated by the two methods agree well. However, when the particle diameter was 23.7 nm and 16.9 nm and the average charge was larger than 30, the average charge calculated by SMPS was slightly smaller than that calculated by aerosol electrometer and CPC. This is because of the measurement limit of SMPS. As illustrated in , the electrical mobility of 56.4 nm particles with high charges lay within the measurement range of SMPS, but that of 16.8 nm particles lay about or over the measurement limit of SMPS; thus, the average charge was underestimated.
FIG. 9 Comparison of the average charges, which was calculated in two ways, SMSP and aerosol electrometer(AE)/CPC, for several charging conditions when the diameter of the particles was 16.9 nm, 23.7 nm, 33.4 nm, and 51.4 nm, when the corona voltage and external voltage were +4 kV and −1 kV, respectively and the temperature of the saturator was 55° C.
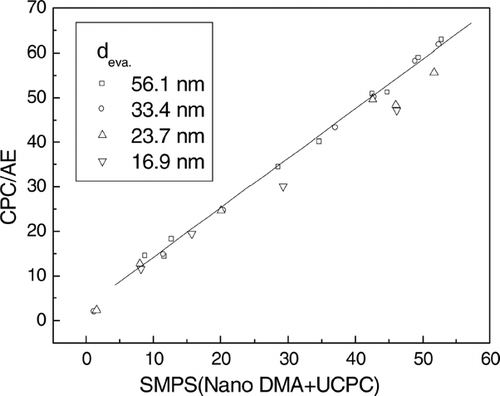
FIG. 10 The shift of the electrical mobility distribution of highly-charged particles compared with evaporated particles with a charge of +1 when the diameter of the evaporated particles was (a) 56.4 nm (b) and 16.9 nm when the corona voltage and external voltage were +4 kV and −1 kV, respectively, and the temperature of the saturator was 55° C.
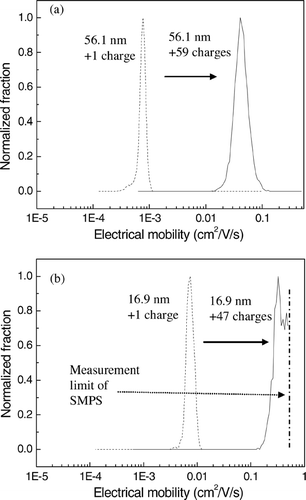
The charge distribution of the evaporated particles, when the temperature of saturator was 55°C and the corona voltage and external voltage were +4 kV and –1 kV, respectively, is illustrated in . The charge distributions of the particles, except for 16.9 nm particles, were similar, even though the initial particle diameter was different. The charge distribution of 16 nm particles was not measured correctly, due to the measurement limit of SMPS. shows that the particles were charged uniformly by using the charging method developed.
FIG. 11 The charge distribution of evaporated particles when the corona voltage and external voltage were +4 kV and −1 kV, respectively, and the temperature of the saturator was 55° C.
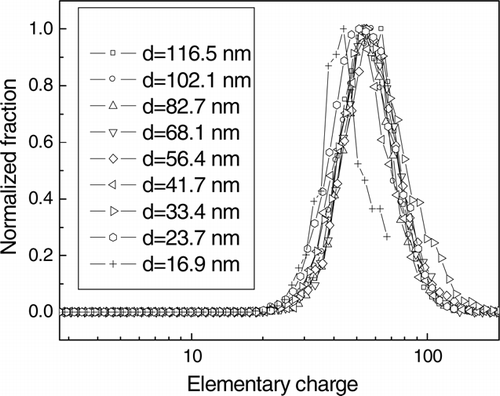
shows a comparison of the average charge of the condensed droplets, classified particles and evaporated particles with the results of previous research. The charging method developed in our study performs much better than conventional corona charging. As mentioned above, the average charges of condensed droplets and of evaporated particle calculated by two manners were similar to one another and independent of particle diameter. The ion evaporation phenomenon was not detected clearly in our study, due to low charge density of particles smaller than 40 nm.
CONCLUSIONS
A uniform charging method for submicron and nano silver particles using condensation was developed and evaluated for various conditions of condensation, charging, and evaporation.
Silver particles were grown into micro droplets of monodisperse size distribution by condensation and their size could be controlled easily by varying the temperature of a saturator. The diameter and GSD of the droplets were the same, even though particle diameter, as classified by DMA, was different.
The condensed droplets evaporated in an evaporator. The GSD of the evaporated particles was identical with that of the classified particles but the diameter of the evaporated particles was greater than that of the original particles, due to recondensation.
The evaporated particles had a uniform charging performance with respect to charge distribution and average charge, regardless of particle diameter. The results agree with our expectations. This is because the particles were charged under the same conditions, due to condensation. The average charge of the particles could be controlled by varying the conditions of condensation (the temperature of saturator) and charging (ion concentration and electric filed strength). The average charge of highly charged silver particles was calculated by two methods: (1) measuring the charge distribution of the particles by SMPS spectrometer, (2) measuring the total charge and number concentration of the particles by aerosol electrometer and CPC. Each result agrees well, but the charge distribution and average charge of particles less than 16.9 nm in diameter cannot be measured exactly, due to the measurement limit of SMPS.
It has been reported widely that when a highly charged droplet whose residue particle is smaller than 40 nm evaporates, the charge on the droplet would evaporate, even though its charge density is lower than the Rayleigh limit. However, in our study, the average charge of the evaporated particles lower than 40 nm changed little and the ion evaporation phenomenon was not detected clearly. This result goes against our expectations. Further, in some cases, the average charge of the particles was greater than that reported in previous research (Citationde la Mora et al. 1995; CitationSuh et al. 2005). It is not yet certain that ion evaporation occurs during the evaporation of highly charged droplets. This is because the charge density of a droplet is not sufficiently high for the charge to evaporate from the surface of the droplet. More detailed study about this is required, and a more precise device for measuring the electric mobility of highly charged particles is needed in order to confirm the occurrence of the ion evaporation.
Acknowledgments
This research was supported partially by the BK21 project of the Korean Ministry of Science and the Eco-Technopia 21 project of the Korean Ministry of the Environment.
REFERENCES
- Büscher , P. , Schmidt-Ott , A. and Wiedensohler , A. 1994 . Performance of a Unipolar Square Wave Diffusion Charger with Variable Nt-Product . J. Aerosol Sci. , 25 : 651 – 663 .
- Chen , D. R. and Pui , D. Y. H. 1999 . A High Efficiency, High Throughput Unipolar Aerosol for Nanoparticles . J. Nanoparticle Research , 1 : 115 – 126 .
- Kruis , F. E. and Fissan , H. 2001 . Nanoparticle Charging in a Twin Hewitt Charger . J. Nanoparticle Research , 3 : 39 – 50 .
- Loscertales , I. G. and Fernández de la Mora , J. 1995 . Experiments on the Kinetics of Field Evaporation of Small Ions from Droplets . J. Chem. Phys. , 103 : 5041 – 5060 .
- Park , S. H. and Lee , K. W. 2000 . Condensational Growth of Polydisperse Aerosol for the Entire Particle Size Range . Aerosol Sci. Technol. , 33 : 222 – 227 .
- Park , S. H. , Lee , K. W. , Shimada , M. and Okuyama , K. 2002 . Change in Particle Size Distribution of Aerosol Undergoing Condensational Growth: Alternative Analytical Solution for the Low Knudsen Number Regime . J. Aerosol Sci. , 33 : 1297 – 1307 .
- Reischl , G. P. , Mäkelä , J. M. , Harch , R. and Necid , J. 1996 . Bipolar Charging of Ultrafine Particles in the Size Range Below 10 nm . J. Aerosol Sci. , 27 : 931 – 949 .
- Suh , J. S. , Han , B. W. , Kim , D. S. and Choi , M. S. 2005 . A Method for Enhanced Charging of Nanoparticles Via Condensation Magnification . J. Aerosol Sci. , 36 : 1183 – 1193 .
- Wiedensohler , A. , Büscher , P. , Hansson , H. C. , Martinsson , B. G. , Stratmann , F. , Ferron , G. and Busch , B. 1994 . A Novel Unipolar Aerosol Charger for Ultrafine Aerosol Particles with Minimal Particle Losses . J. Aerosol Sci. , 34 : 639 – 649 .