Abstract
The Aerodyne aerosol mass spectrometer (AMS) employs flash vaporization (600°C) followed by 70-eV electron impact ionization (EI) to detect organic and inorganic aerosols. The signal at mass-to-charge ratio (m/z) 44 (mainly CO 2 + ) is considered the most reliable marker of oxygenated organic aerosol. This study is the first to evaluate the contribution of selected low molecular weight dicarboxylic acids (diacids) and ω-oxocarboxylic acids (ω-oxoacids) to the particle-phase m/z 44 signal of the AMS mass spectrum. Ambient measurements were conducted at a surface site in Tokyo (35°39 ′ N, 139°40 ′ E) during August 3–8, 2003. Diacids and ω-oxoacids were measured using a filter sampling followed by extraction, derivation, and gas chromatograph-flame ionization detector (GC-FID) analysis. The mass concentrations of diacids and ω-oxoacids show tight correlation with the m/z 44 signal (r 2 = 0.85–0.94) during the measurement period. Laboratory experiments were also performed to determine the fragment patterns of selected diacids (C2–C6 diacids and phthalic acids) and ω-oxoacid (glyoxylic acid) in ambient aerosols. Here, we report for the first time that the selected organic acids could account for 14 ± 5% of the observed m/z 44 signal on average during the measurement period. Oxalic acid (C2) is the largest contributor, accounting for 10 ± 4% of the observed m/z 44 signal. These results would be useful for interpreting the m/z 44 signals obtained from ambient measurements in various locations.
1. INTRODUCTION
Organic compounds are ubiquitous in ambient aerosols and constitute approximately 10–70% of total dry fine particle mass in the troposphere (CitationTurpin et al. 2000, and references therein). The fraction of water-soluble organic carbon (WSOC) is important in estimating direct and indirect radiative forcing of aerosols because they can significantly alter the hygroscopic properties of aerosols (CitationSaxena et al. 1995). Dicarboxylic acids (diacids), which contain two carboxyl groups, are the major constituents of WSOC and can be found in various locations in the troposphere (CitationSaxena and Hildemann 1996; CitationKawamura and Ikushima 1993; CitationKawamura and Yasui 2005; CitationMochida et al. 2003). CitationKawamura and Ikushima (1993) suggested that oxidation of volatile organic compounds (VOCs) is the major source of low molecular weight (C2–C6) diacids observed at an urban site in Tokyo, based on the seasonal variation of these diacids. More recently, CitationErvens et al. (2004) proposed that cloud processing could be an important source of low molecular weight diacids, although there are still many uncertainties in the production pathway of these compounds.
The Aerodyne aerosol mass spectrometer (AMS) can provide size-resolved mass concentrations of submicron non-refractory (NR-PM1) aerosols with a time resolution of the order of minutes (CitationJayne et al. 2000). The AMS employs flash vaporization followed by electron impact (EI) at ionization energy of 70 eV. There are three kinds of AMS, classified by the type of detector employed: quadrupole AMS (Q-AMS), compact time-of-flight AMS (C-ToF-AMS), and high-resolution ToF-AMS (HR-ToF-AMS) (CitationDeCarlo et al. 2006). In this study we use a Q-AMS, which is hereafter referred to simply as the AMS. The mass spectrum of “organics” is defined as the residual fragments after subtracting all the identified signals originating from ambient gas molecules, inorganic compounds, and instrumental artifacts such as sodium (Na) and potassium (K) ions from surface ionization on the vaporizer (CitationAllan et al. 2004). For some organic compounds, the mass spectra of the AMS have been compared to those from the standard reference database provided by the National Institute of Standards and Technology (NIST) (http://webbook.nist.gov/chemistry/) (CitationAlfarra 2004). CitationAlfarra (2004) has found that the fragmentation patterns of the AMS are generally shifted to smaller mass-to-charge ratios (m/z) because of its higher vaporization/ionization temperature (600°C) compared to those obtained by gas chromatograph-mass spectrometer (GC-MS) (100°–200°C). In the AMS, decarboxylation and other thermal degradation often occur prior to ionization.
In spite of this complexity, some m/z peaks can be used as distinct markers of hydrocarbon-like or oxygenated organic aerosols. The signal at m/z 44, which originates mainly from CO2 +, is considered the most reliable marker of oxygenated organic aerosol (CitationZhang et al. 2005b; and references therein). Previous studies showed that the increase in m/z 44 signal was often associated with photochemical processing of air masses (CitationTakegawa et al. 2006a, Citation2006b; CitationZhang et al. 2005a), and that the m/z 44 signal correlated well with the mass concentrations of WSOC (CitationKondo et al. 2007). Diacids are considered the major compounds that can contribute significantly to the m/z 44 signal, as well as ω-oxocarboxylic acids (ω-oxoacids) containing carboxyl and aldehyde groups. However, the contribution of these compounds in ambient aerosols to the m/z 44 signals has not been evaluated. Further, there has been no published comparison between the m/z 44 signal and the mass concentrations of organic acids determined by GC analysis.
The purpose of this paper is to evaluate the contribution of selected low molecular weight diacids and ω-oxoacids to the m/z 44 signal of AMS mass spectra. Here we use ambient measurement data obtained in Tokyo in August 2003 and laboratory experiment data obtained using authentic standards of the selected organic acids. This study will present fundamental information that could be applied to better interpret AMS data obtained in various locations. This information would also be useful for the analysis of ToF-AMS data because ToF-AMS employs the same vaporization/ionization scheme as Q-AMS.
2. EXPERIMENTAL
2.1. Ambient Measurements
2.1.1. Observation Site
Ambient measurements were conducted at a surface site in Tokyo from 21:00LT on August 3 to 20:00LT on August 8, 2003. The observation was made on the top floor of a 5 story building (∼18 m above ground level) on the campus of the Research Center for Advanced Science and Technology (RCAST), University of Tokyo (35°39′N, 139°40′E). RCAST is located near the center of the city and is surrounded by a major highway system (at ∼2 km distance from RCAST). A number of on-line instruments including the AMS were installed in the observation room (CitationTakegawa et al. 2006a). The AMS data were recorded every 10 min.
The mass concentrations of diacids and ω-oxoacids were measured using a filter sampling method followed by GC analysis. The aerosol samples were collected every 3 h during August 4–5, 2003 on a pre-combusted quartz filter using a high-volume air sampler without any size-cut. After August 5, the aerosol samples were collected every 12 h. The high-volume sampler was placed on the rooftop of the observation building.
2.1.2. Quantification Procedure of the Aerodyne AMS for Ambient Data
The AMS quantification procedure for ambient data has been described previously (e.g., CitationJimenez et al. 2003, CitationAlfarra et al. 2004). The key equations for organic aerosol are presented here because the expression used in this paper is slightly different from those in previous publications. Similar expressions can be applied to the mass concentrations of inorganic compounds (equations for inorganics are not shown here).


TABLE 1 Definition of parameters used in this study
The IE
NO3
was routinely determined by supplying mono-disperse ammonium nitrate (NH4NO3) particles from a calibration unit. The uncertainty in determining IE
NO3
was estimated to be 14% (CitationTakegawa et al. 2005). The uncertainty in the RIE values for major inorganic compounds (sulfate, nitrate, chloride, and ammonium) is considered to be small because the mass spectra of these compounds are well defined. The RIE values for organics can vary with functional group: ∼ 2 for hydrocarbons and ∼ 1.4 for oxygenated organic compounds (CitationJimenez et al. 2003). We assume = 1.4 based on the work of CitationAlfarra et al. (2004). The CE values are assumed to be 0.5 both for inorganic and organic compounds.
For the major inorganic compounds, the combined uncertainty (accuracy) due to IE
NO3
, RIE
inorg
, and CE
inorg
(inorg = sulfate, nitrate, chloride, and ammonium) has been evaluated based on intercomparison with a Particle-Into-Liquid Sampler combined with Ion Chromatography (PILS-IC) (CitationTakegawa et al. 2005). The intercomparison was performed on July 23–30 of 2003 (prior to the filter sampling period). A PM1 cyclone (URG Corp., USA) was used for the PILS-IC inlet because the size-cut of the AMS (aerodynamic lens) is similar to the commonly used PM1 cutoff. The linear regression slope of the AMS versus PILS-IC ranged from 0.81 to 1.26 for the major inorganic compounds. Therefore, the combined uncertainty due to IE
NO3, RIE
inorg
, and CE
inorg
(and also to the approximation of the AMS size-cut to PM1) is estimated to be less than 26%. We assume that this error estimate is also applicable to organic compounds: i.e., the combined uncertainties in M
m/z
and OA due to IE
NO3
, , and
are both assumed to be 26%. Although we use a constant value for the contribution of gas-phase CO2 at m/z 44 (equivalent to 0.09 μ g m− 3), the uncertainty of M
44 due to the variability in the gas-phase CO2 is relatively minor (∼ 0.002 μ g m− 3). The overall validity of the quantification of organic aerosol by the AMS has been evaluated based on intercomparison with a Sunset Laboratory semi-continuous elemental and organic carbon (EC/OC) analyzer (CitationTakegawa et al. 2005). The linear regression slope of the AMS OA versus Sunset OC was found to be 1.8, which is consistent with the expected ratio of organic matter (OM) to OC in urban air (CitationTurpin and Lim 2001).
2.1.3. Measurements of Dicarboxylic Acids and ω-Oxocarboxylic Acids in Aerosol Samples
Details of the analytical method have been presented by CitationKawamura and Yasui (2005), and thus only a brief description is given here. The filter samples were extracted with pure water and concentrated using a rotary evaporator in the laboratory. The concentrates were reacted with 14% BF3/n-butanol at 100°C to derive the carbonyl groups to butyl esters. The butyl esters were extracted with n-hexane after adding pure water and then determined using a gas chromatograph-flame ionization detector (GC-FID). Peak identification was performed by comparison with the GC retention times of the samples with those of authentic standards. Identification of the esters was confirmed by GC-MS analysis. The uncertainty in the measurements is given in CitationKawamura and Yasui (2005). Recoveries of authentic standards spiked to a pre-combusted quartz filter were 71% for oxalic (C2) acid and better than 80% for malonic (C3), succinic (C4), and adipic (C6) acids. Procedural blank filter samples showed small peaks of oxalic and phthalic (Ph) acids in the GC chromatograms, although they were small (< 2% for oxalic acid and < 5% for phthalic acid). The data used in this analysis are corrected for the procedural blanks but are not corrected for the recoveries. The analytical errors are within 15% for these major species.
2.2. AMS Laboratory Experiments
Laboratory experiments were performed between July 2005 and April 2006 to estimate the fragment patterns of selected diacids (C2–C6 and Ph) and ω-oxoacid (glyoxylic acid: ω C2) in the AMS vaporization/ionization system. We have selected these organic acids because these compounds were the major constituents of total diacids and ω-oxoacids during the measurement period (see section 3.1.1). The physical parameters of the selected organic acids are given in . These parameters were taken from the International Chemical Safety Cards (ICSC) of the International Labour Organization (ILO) (http://www.ilo.org/) and also from Chemical Dictionary (1994). The selected organic acids can be vaporized and/or decomposed at the temperature of the AMS vaporizer (600°C). The particle generation system used in this study is basically the same as that described in CitationTakegawa et al. (2005). The system consists of a Collison atomizer (Model 3076, TSI, Inc., USA), two diffusion dryers, and a scanning mobility particle sizer (SMPS) (Model 3936, TSI, Inc., USA). The organic acid particles were generated by atomizing water solutions of authentic standards of these compounds. Pure oxygen (O2) was used to atomize the water solutions in order to detect the m/z 28 signal (mainly CO+) originating from the organic acids. The whole system was purged with O2 for a few hours prior to each experiment to eliminate the contribution of N2 + to the m/z 28 signal. The possible dependence of the fragment patterns on carrier gas and mass loadings is discussed in the appendix. We obtained ∼ 10 mass spectra for each compound during the laboratory experiment period. The integration time for each mass spectrum was generally ∼ 10 min.
TABLE 2 Physical parameters of selected organic acids
The “best estimate” of the mass spectrum for each compound is determined using the following procedure (a)–(c): (a) Each mass spectrum is normalized so that the sum of all m/z peaks is equal to unity. For a compound i (= C 2, C 3, etc.), the m/z component of each normalized mass spectrum is expressed as u m/z i (∑ m/z u m/z i = 1). The u m/z i value corresponds to the ratio of the m/z signal to the total signal. (b) The average u m/z i (ū m/z i ) is calculated for each compound (from ∼ 10 mass spectra for each compound). The ū m/z i is referred to as the best-estimate mass spectrum. Note that ∑ m/z ū m/z i = 1 holds without additional normalization. (c) The standard deviation of u m/z i is used as a measure of the uncertainty. Note that the complete table of the best-estimate mass spectra is available as electronic supplemental material.
3. RESULTS
3.1. Ambient Measurements
3.1.1. Mass Concentrations
summarizes the average and median values of OA and M 44 measured by the AMS and those of selected diacids (C2–C6 and Ph) and ω-oxoacid (ω C2) measured by the GC analysis.
TABLE 3 Average mass concentrations of organic compounds on August 3–8, 2003
The AMS data were averaged according to the integration time of the filter samples before calculating the average and median values. The total diacids (or ω-oxoacids) represent the sum of all diacids (or ω-oxoacids) identified by the laboratory analysis. The full list of the identified diacids and ω-oxoacids are given in CitationKawamura and Yasui (2005). As described in section 2.2, only major diacids and ω-oxoacid were selected for the present analysis. On average, the sum of the selected diacids (C2–C6 and Ph) accounts for ∼ 87% of the total diacids (C2–C12), and glyoxylic acid accounts for ∼ 91% of the total ω-oxoacids (C2–C9). Oxalic acid is found as a dominant diacids species. This feature has been observed in various locations in previous studies (CitationKawamura and Ikushima 1993; CitationKawamura and Yasui 2005; CitationMochida et al. 2003). shows the time series of OA and M 44 observed by the AMS and the mass concentrations of oxalic acid (C2), total diacids, and total ω-oxoacids measured by the filter sampling technique. Both M 44 and di-/ω-oxo-acids show a distinct peak at around noon. shows the correlation plots of oxalic acid, total diacids, and total ω-oxoacids versus M 44. The linear regression parameters (slope, intercept, and r 2) for the selected diacids and ω-oxoacids versus M 44 are summarized in . The high correlation coefficients (r 2 = 0.85–0.94) indicate a strong relationship between M 44 and di-/ω-oxo-acids.
FIG. 1 Time series of (a) the mass concentrations of total organics and M 44 observed by the AMS and (b) those of oxalic acid (C2: solid), total dicarboxylic acids (total diacids (C2-C12): open), and total ω-oxocarboxylic acids (total ω-oxoacids (C2-C9): shaded) measured by filter sampling followed by laboratory analysis.
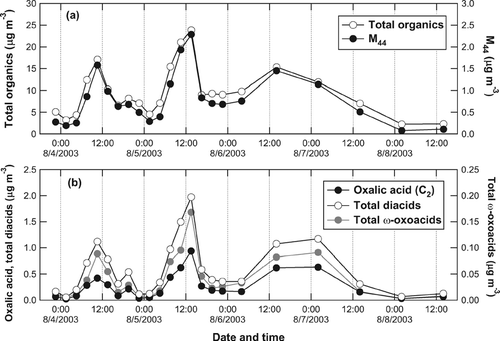
FIG. 2 Correlation plots of oxalic acid (solid), total diacids (open), and total ω-oxoacids (shaded) versus M 44. The lines represent the linear regression results.
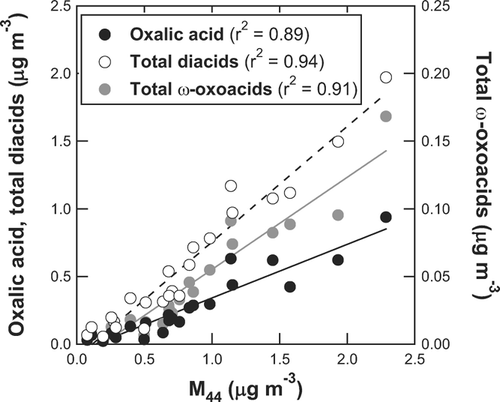
TABLE 4 Linear regression parameters for selected diacids and ω-oxoacids versus M 44
The ozone (O3) mixing ratios measured at the same location also exhibited a distinct peak at around noon during the measurement period (CitationTakegawa et al. 2006a). Specifically, high concentrations of O3 exceeding 100 parts per billion by volume were observed at around noon on August 4–6, 2003. The mass concentrations of M 44, oxalic acid, total diacids, and total ω-oxoacids showed positive correlations with O3 during the daytime (06:00–18:00LT), although the r 2 values were not very high (0.66, 0.51, 0.59, and 0.62, respectively) (correlation plots are not shown here). The positive correlation with O3 suggests that photochemical production could be an important source of these organic acids during the measurement period. This is consistent with the findings by CitationKawamura and Ikushima (1993).
3.1.2. AMS Mass Spectrum
represents the average mass spectrum of organics obtained at 12:00–15:00LT on August 5, 2003. Based on laboratory experiments that have previously been performed, the standard AMS analysis procedure assumes that the m/z 18 signal (H2O+) from organics is equal to the m/z 44 signal (CitationAllan et al. 2004). This is because the high background level at m/z 18 generally makes it difficult to estimate the m/z 18 signal originating from ambient particles and also because H2O molecules produced from thermal decomposition of organics are not distinguishable from those originating from particle-phase H2O. On the other hand, the AMS standard analysis procedure does not include the m/z 28 signal originating from organics (mainly CO+) because the observed m/z 28 signal is dominated by nitrogen (N2 +) in air. In this analysis, we assume that the m/z 28 signal from organics is also equal to the m/z 44 signal during the measurement period. The basis for this assumption is described below.
CitationZhang et al. (2005b) first evaluated the m/z 28 signal from organics using particle-time-of-flight (PToF) data. They used an exponential fit that has the same function form as the one-dimensional Boltzmann velocity distribution functional in order to represent the N2 + signal in the PToF mode. They have found that the m/z 28 signal from organics could be ∼ 30% larger than m/z 44 during the Pittsburgh Air Quality Study. Here we use a simpler method to estimate the contribution of organics to the m/z 28 signal. shows the plots of signal at m/z 28 versus PToF observed during the daytime (high SOA) and nighttime (low SOA). The broad peak at PToF = 0–2 ms corresponds to N2 + in ambient air. The small difference between the daytime and nighttime at the tail of the N2 + signal (PToF = 2–8 ms) corresponds to the organic fragment. The N2 + peak height of the high SOA data agreed with that of the low SOA data to within 0.3%. represents the size distributions of Δ S 28 and Δ S 44, where Δ denotes the difference between the daytime and nighttime data. The conversion of PToF to vacuum aerodynamic diameter (d va ) is based on polystyrene latex (PSL) calibrations. Although Δ S 28 is very noisy because of the large contribution of N2 + to the baseline, it exhibits a very similar size distribution to Δ S 44. The signals were integrated over the size range of d va = 30–2000 nm. The ratio of the integrated Δ S 28 to the integrated Δ S 44 is found to be 1.0, suggesting the importance of m/z 28 for the analysis of organics. Based on this result, we assume that the m/z 28 signal from ambient organic aerosol is equal to m/z 44 during the measurement period.
3.2. AMS Laboratory Experiments
3.2.1. Mass Spectra of Oxalic Acid and Its Salts
The best-estimate mass spectrum of oxalic acid obtained in the laboratory is shown in . The major peaks of the selected organic acids are summarized in . The mass spectrum from the NIST standard reference database is also shown for comparison. In the AMS mass spectrum, m/z 18 (H2O+), 28 (CO+), and 44 (CO2 +) are the three major peaks. The m/z 44 peak is the largest, accounting for ∼ 34% of the sum of all m/z peaks. The signals at m/z 16 (O+) and 17 (OH+) originating from oxalic acid were estimated from the m/z 18 signal, based on the fragment pattern of H2O in the AMS ionizer (CitationAllan et al. 2004). In the NIST mass spectrum, m/z 45 (COOH+) and 46 (CH2O2 +) are the two major peaks. The similarity between the AMS and NIST mass spectra is the positions of the significant peaks such as m/z 18, 28, 44, 45, and 46, while the major difference is the relative intensities of those peaks: the contributions of the m/z 18, 28, and 44 peaks in the AMS mass spectrum are much larger than those in the NIST spectrum. Thermal decomposition of oxalic acid could result in the formation of H2O (dehydration), CO, CO2 (decarboxylation), and formic acid (HCOOH) (CitationChemical Dictionary 1994). It is suggested that dehydration and decarboxylation of oxalic acid could be more significant in AMS spectra than those of NIST due to the higher vaporizer temperature of the AMS (600°C compared to 100°–200°C).
FIG. 5 Mass spectrum of oxalic acid obtained in the laboratory (solid bars). Mass spectrum from the NIST standard reference database is also shown as shaded bars. Each mass spectrum has been normalized so that the sum of all m/z peaks is equal to unity.
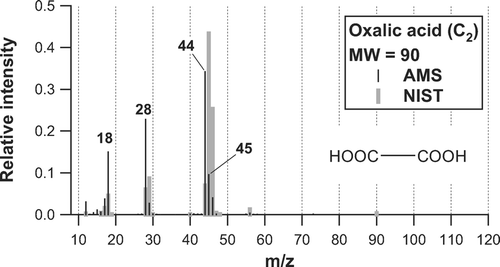
TABLE 5 Major mass fragment ions derived from various organic acids
It should be noted that the standard AMS analysis procedure does not assign organic fragments for m/z 46 (only nitrate fragment) (CitationAllan et al. 2004). Considering the fragment patterns and RIE of nitrate and organics, the m/z 46 signal from oxalic acid could be comparable to that from nitrate if the mass concentration of oxalic acid is 6–7 times greater than nitrate. Note that the interference of the m/z 46 signal may not be a problem for the newly developed HR-ToF-AMS (CitationDeCarlo et al. 2006) because it can distinguish NO2 + from CH2O2 +.
shows the mass spectra of sodium oxalate ((COONa)2) and ammonium oxalate ((COONH4)2) obtained in the laboratory, illustrating the possible dependence of the fragment pattern of oxalate on its counter ions. The NIST mass spectrum is not available for these compounds. The m/z 45 and 46 peaks do not show up in the mass spectrum of (COONa)2, which is expected from its elemental composition. This result indicates that the mass spectrum of (COONa)2 could be significantly different from (COOH)2. On the other hand, the major peaks in the mass spectrum of (COONH4)2 are similar to those of (COOH)2. shows the comparison of the individual m/z signals between (COONH4)2 and (COOH)2. Each m/z signal has been normalized by the m/z 44 signal (base peak). The relative intensities of the m/z 18, 28, 44, 45, and 46 signals for (COONH4)2 agree well with those for (COOH)2. However, the mass spectrum of (COONH4)2 cannot be explained by the sum of (COOH)2 and NH3: i.e., the observed m/z 16 (NH2 +) and 17 (NH3 +) peaks, which are the major fragments of NH3, appears smaller by a factor of ∼ 3 than those expected from the RIE NH3 value for ammonium sulfate/nitrate (RIE NH3 = 3.5). There are two possible explanations for this feature. The first possibility is that (COONH4)2 is mainly decomposed to (COOH)2 and NH3 but the RIE NH3 value for (COONH4)2 is significantly smaller than that for ammonium sulfate/nitrate. The second possibility is that (COONH4)2 is not simply decomposed to (COOH)2 and NH3. In fact, (COONH4)2 could be converted to oxalic diamide, (CONH2)2 by a dehydration process at high temperatures (CitationChemical Dictionary 1994). In order to test the second possibility, we examined the mass spectrum of (CONH2)2 in the laboratory. shows the comparison of the individual m/z signals between (COONH4)2 and (CONH2)2. Again, each m/z signal has been normalized by the m/z 44 signal. The relative intensities of the m/z 18, 28, 44, 45, and 46 signals for (COONH4)2are significantly different from those for (CONH2)2, indicating that the second possibility is unlikely. It is interesting to note that the base peak of (CONH2)2 is m/z 44 (CONH2 +), which implies that amide compounds could be an important source of m/z 44 signal in some cases.
FIG. 6 Mass spectra of sodium oxalate ((COONa)2) and ammonium oxalate ((COONH4)2) obtained in the laboratory.
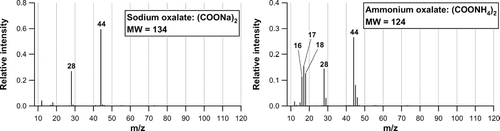
FIG. 7 (a) Comparison of the individual m/z signals between (COONH4)2 and (COOH)2 and (b) between (COONH4)2 and (CONH2)2. Each m/z signal has been normalized by the m/z 44 signal (base peak). The m/z numbers are shown beside the data points.
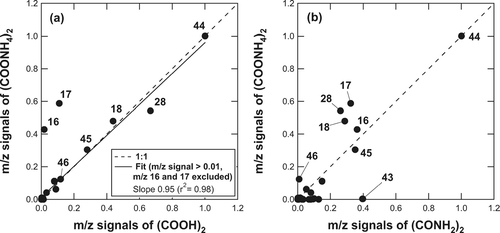
The filter sampling/GC analysis detects both (COONH4)2 and (COONa)2 as (COOH)2. In the case of this study, the contribution of (COONa)2 in the AMS mass spectrum is likely negligible because of the low concentration of Na+ in the PM1 mode during the measurement period (CitationTakegawa et al. 2005). The contribution of (COONH4)2 may not be negligible because there was enough NH3 to fully neutralize sulfate, nitrate, and chloride during this period (CitationMorino et al. 2006). However, we can use the mass spectrum of (COOH)2 for the present analysis assuming that (COONH4)2 is mainly decomposed to (COOH)2 and NH3 on the AMS vaporizer. Same assumption is applied to the other selected organic acids. Note that the RIE NH3 issue remains uncertain and requires additional laboratory experiments in future studies.
3.2.2. Mass Spectra of Other Selected Organic Acids
Mass spectra of the other selected diacids (C3–C6 and Ph) and ω-oxoacid (ω C2) obtained in the laboratory are shown in . The positions of the significant peaks are similar to the NIST database, although the major fragments of the AMS mass spectrum are shifted to smaller m/z numbers as compared to NIST. The difference can be attributed to the higher vaporization temperature of the AMS than used in the NIST experiments, as mentioned earlier. These similarities and differences between the NIST and AMS mass spectra have been noted in CitationAlfarra (2004), although m/z 28 is not included in the mass spectra of organic compounds given in CitationAlfarra (2004).
It should be noted that the m/z 39 signal (mainly C3H3 +) is present in the mass spectra of relatively higher molecular weight diacids (C4–C6 and Ph). The m/z 39 signal is also detected in the NIST mass spectra. The AMS standard analysis procedure does not include the m/z 39 signal originating from organics because there is an artifact of K+ produced by surface ionization on the vaporizer. The artifact of K+, which varies with each instrument, depends on the condition of the vaporizer and perhaps on the loadings of ambient aerosol, which generally makes it difficult to extract the m/z 39 signal originating from organic compounds. shows the correlation plots of S 39 versus ∑S m/z for C2 and C5 diacids. No significant S 39 was observed for C2 diacid, which is expected from its elemental composition. On the other hand, S 39 shows tight correlation with ∑S m/z for C5 diacid (∼ 6% of the total), indicating that m/z 39 is one of the significant peaks for this compound. The m/z 39 signal was also found in the mass spectra for C4, C6, and Ph diacids (). The r 2 values of S 39 versus ∑S m/z were 0.88, 0.99, and 0.99 for C4, C6, and Ph diacids, respectively. The m/z 39 signals from the selected organic acids are negligibly small as compared to the signals from total organics during the measurement period, given the low ambient concentrations of C4–C6 and Ph. However, there may be some other cases where the m/z 39 signal from organics could become important. Again, this may not be a problem for the HR-ToF-AMS because it can distinguish C3H3 + from K+.
FIG. 9 Correlation plots of the m/z 39 signal (S 39) versus the sum of all m/z signals (∑S m/z ) for C2 and C5 diacids. The line represents the linear regression for the C5 diacid data.
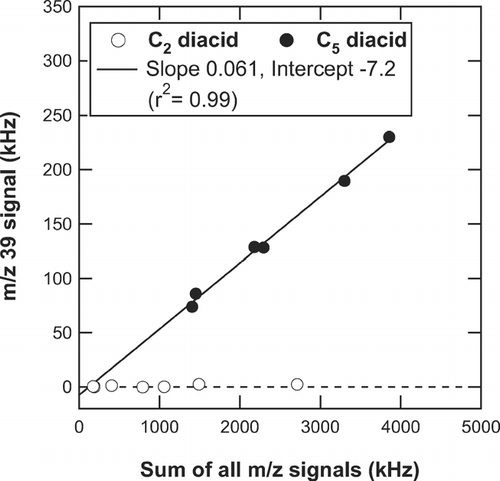
gives the summary of the m/z 44 fraction and the relationship between m/z 44 and the other m/z signals (m/z 18, 28, 39, and 46) for the selected organic acids. In ambient data, the m/z 18, 28, 39, and 46 signals originating from organic aerosol are generally difficult to extract from the observed mass spectra. This information provides the basis for estimating those signals using the observed m/z 44 signal.
TABLE 6 Fraction of m/z 44 signal and relationship between m/z 44 and other m/z signals for selected organic acids
4. DISCUSSION
4.1. Estimate of m/z 44 Signals from Selected Organic Acids in Ambient Aerosol
Here we estimate the mass spectra of the selected diacids and ω-oxoacid in ambient aerosol in a bottom-up manner. The estimate is based on the ambient mass concentrations obtained from the filter sampling and the fragment patterns characterized in the laboratory. The signal at an m/z peak originating from a compound i (= C 2, C 3, etc.) in ambient aerosol, s m/z i , is estimated as follows (reverse of Equation [Equation1]):
From Equation (Equation1) and Equation (Equation3), the contribution ratio of compound i to the observed m/z signal, R m/z i , is estimated as follows:
Although we assume CE
i
/ = 1, RIE
i
/
= 1, and f
i
/ϵ
i
= 1 in the following discussion, these factors are explicitly included in Equation (Equation4) to evaluate the possible uncertainties (see next section).
shows the estimated mass spectra of oxalic acid (C2) and the sum of the other selected acids (C3–C6, Ph, and ω C2) in ambient aerosol at 12:00–15:00LT on August 5, 2003, together with the observed mass spectrum of total organics. It can be seen that m/z 28 and m/z 44 are the largest two peaks from the selected acids and have significant contributions to the mass spectrum of total organics. shows the time series of R 44 C2, R 44 selected , and M 44, illustrating the contribution ratios of oxalic acid and the selected acids to the m/z 44 signal during the measurement period. The 10-min average of M 44 is also shown for comparison. Both R 44 C2 and R 44 selected tend to be higher during the daytime, suggesting that these ratios were largely controlled by photochemical activity. It should be noted that R 44 selected reaches as high as 24% during the midnight of August 7 (8/6/2006 20:00-8/7/2006 8:00). The 10-min M 44 data indicates that there was a significant enhancement of M 44 during this time period. Possible explanations for the nighttime peak of SOA (i.e., formation of SOA by O3 and NO3 and/or shift in gas/particle partitioning of SOA) were discussed in CitationTakegawa et al. (2006a). Note that the last two data points obtained on August 8 are not used for calculating the R 44 C2 and R 44 selected values because the relative errors of these data points may be large considering the low concentrations of M 44 and possible artifacts in the diacid measurements at low concentrations (CitationRay and McDow 2005).
FIG. 10 Mass spectra of total organics (grey), oxalic acid (C2: orange), and the sum of the other selected acids (C3–C6, Ph, and ω C2: blue) in ambient aerosol at 12:00–15:00LT on August 5, 2003. Note that the blue bars are stuck on the orange bars.
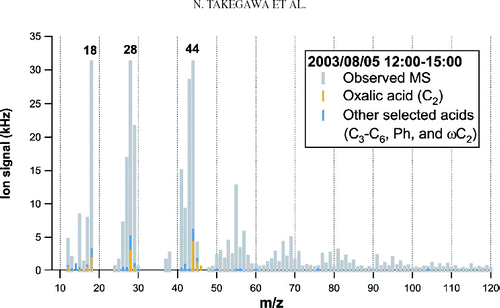
FIG. 11 Time series of M 44 (3-12-h average: thick shaded line; 10-min average: thin shaded line) and the contribution ratios of oxalic acid (solid circles) and the sum of the selected acids (open circles) to S 44.
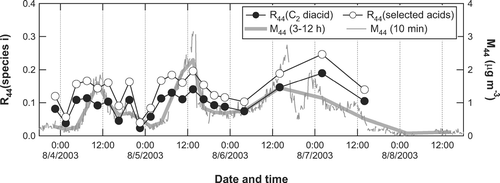
On average, the selected acids account for 14 ± 5% of S 44. The difference in the integration time of the filter samples (3–12 h) is not taken into account in calculating the average. The error represents the standard deviation and does not include the uncertainties in the ambient measurements and laboratory experiments. These uncertainties will be discussed in the next section. Oxalic acid is the largest contributor, accounting for 10 ± 4% of S 44 on average. It should be noted that seven low molecular weight compounds alone could explain a substantial fraction (∼ 14%) of M 44 observed during the measurement period. The rest of M 44 may have originated from mono-/poly-carboxylic acids and perhaps from peroxides because these compounds could produce CO2 + in the vaporization and ionization processes of the AMS. In fact, significant amounts of mono-/poly-carboxylic acids have been found in urban aerosol (e.g., CitationAlves et al. 2002; CitationFuzzi et al. 2001; CitationRogge et al. 1993).
4.2. Possible Uncertainties
Possible uncertainties in estimating the contributions of oxalic acid to the m/z 44 signal are now evaluated using Equation (Equation4). The uncertainty in u
44
C2 is estimated to be 8% based on . The uncertainty in m
C2 is estimated to be 15%, as described earlier. The uncertainty in M
44 is assumed to be 26%, as described in section 2.1.2. The uncertainties in RIE
C2/ and CE
C2/
depend on how the RIE and CE values of oxalic acid are similar to or different from those of total organic aerosol. Because the assumed
(= 1.4) is close to the RIE values for oxygenated organic compounds, the uncertainty in RIE
C2/
would be small. CitationAlfarra (2004) showed that CE values of pure C4 diacid particles generated in the laboratory were smaller than 0.5. However, it has been demonstrated that solid compounds generated in the laboratory (e.g., (NH4)2SO4) generally show lower CE values than those in ambient air. Assuming that oxalic acid in ambient aerosol is internally mixed with most of the organic compounds in ambient air, the uncertainty in CE
C2/
would also be small. The uncertainty in f
C2/ϵ
C2 is estimated as follows. The recoveries are estimated to be ∼ 0.7 for oxalic acid, as described earlier. During the summer of 2004, we conducted measurements of diacids using a high-volume sampler and also using a PM1 low-volume sampler at the RCAST observatory. The analysis and evaluation of the filter measurements are now being performed (Kawamura et al., manuscript in preparation). The preliminary results show that the PM1 diacids account for 40–80% of the high-volume diacids. Based on these results, the f
i
/ϵ
i
values are estimated to be 0.6–1.1 for oxalic acid. Therefore, the uncertainty in f
C2/ϵ
C2 is estimated to be < 40%. In summary, the overall uncertainty in R
44
C2 is estimated to be ∼ 50%.
5. CONCLUSIONS
The relationship between low molecular weight diacids and ω-oxoacids in ambient aerosol and the AMS m/z signal has, for the first time, been investigated based on ambient measurements and laboratory experiments. Oxalic acid was found to be the most abundant compounds of total diacids identified in the filter samples. The mass concentrations of oxalic acid, total diacids, and total ω-oxoacids correlated well with the m/z 44 signal (r 2 = 0.85–0.94), indicating a strong link between m/z 44 and these compounds.
Detailed analysis of ambient mass spectra and particle time-of-flight data suggests that m/z 28 from organics could be comparable to m/z 44 during the measurement period, although the AMS standard analysis procedure does not include m/z 28 for the mass spectrum of organics. Mass spectra of selected diacids (C2–C6, and Ph) and ω-oxoacid (ω C2) were investigated in the laboratory. For the selected compounds, m/z 28 and 44 were found to be the major fragments. Both ambient and laboratory data suggest the importance of m/z 28 for the analysis of organics.
Mass spectra of the selected diacids and ω-oxoacid in ambient aerosol were estimated based on the mass concentrations of these compounds obtained from the filter sampling and the fragment patterns of these compounds characterized in the laboratory. On average, the selected compounds accounted for 14 ± 5% of the observed m/z 44 signal during the measurement period. Oxalic acid was the largest contributor, accounting for 10 ± 4% of the observed m/z 44 signal. These results could be useful for the interpretation of m/z 44 data obtained from ambient measurements.
APPENDIX. DEPENDENCE OF FRAGMENT PATTERNS ON CARRIER GAS AND MASS LOADINGS
In our laboratory experiments, pure O2 was used as the carrier gas (i.e., compressed gas to atomize the water solutions of the selected organic acids) in order to detect the m/z 28 signal originating from the organic acids. Synthetic air (N2+ O2) and argon (Ar) were also used for oxalic acid to examine possible effects of the carrier gas on the mass spectra. In addition, the mass concentrations of oxalic acid were varied by adjusting a dilution flow of O2, in order to examine possible dependence of the fragment patterns on the mass loadings.
shows the correlation of S
44 versus ∑S
m/z
for oxalic acid particles generated with O2, synthetic air, and Ar. Note that the S
28 signal for synthetic air was estimated based on the PToF data (see section 3.1.2). Only data obtained with O2 were used for the linear regression analysis. The S
44 < eqid5 > ∑S
m/z
ratios for the synthetic air and Ar data agreed with the average S
44 < eqid6 > ∑S
m/z
ratio calculated from the O2 data to within 9%, suggesting that the dependence of the fragment pattern on the carrier gas is small. The dynamic range of ∑S
m/z
corresponds to mass concentrations of 3-300 μ g m− 3 if we assume = 0.5 and
= 1.4 in equation [Equation1]. The correlation of S
44 versus ∑S
m/z
exhibits very good linearity (r
2 = 0.99) over the whole mass concentration range considered here. Similar results were obtained for m/z 28 (r
2 = 0.99 for S
28 versus ∑S
m/z
). This result suggests that the dependence of the fragment pattern on the mass concentration is also small.
FIG. A1 Correlation of the m/z 44 signal (S 44) versus the sum of all m/z signals (∑S m/z ) for oxalic acid. The solid, open, and shaded circles represent the data obtained using O2, synthetic air, and Ar, respectively, as the carrier gas of the particle generation system. The line represents the linear regression for the O2 data.
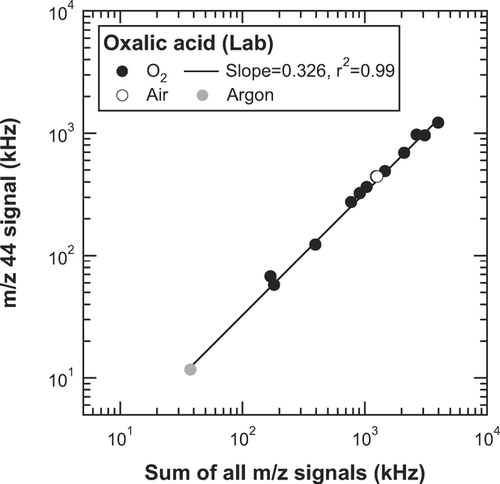
TABLE S1 ELECTRONIC SUPPLEMENTAL MATERIAL
Best-estimate mass spectra (std: standard deviation)
TABLE S2 Best-estimate mass spectra (std: standard deviation)
Acknowledgments
The authors thank M. Koike and K. Asano for their support in the filter sampling. They also thank J. D. Allan for providing the AMS analysis software and M. R. Alfarra, J. L. Jimenez, and D. R. Worsnop for useful information on the fragment patterns of some organic compounds. This study was funded by the Japanese Ministry of Education, Culture, Sports, Science and Technology (MEXT) and the Japanese Science and Technology Agency (JST).
Notes
a The parameters were taken from the International Chemical Safety Cards (ICSC) of the International Labour Organization (ILO) (http://www.ilo.org/).
b The parameters were taken from CitationChemical Dictionary (1994).
a Total organics and M 44 were measured by AMS. Dicarboxylic acids (diacids) and ω-oxocarboxylic acids (ω-oxoacids) were measured using a filter sampling followed by GC-FID analysis. The AMS data were averaged over the integration time of the filter sampling.
b Selected diacids represent the sum of the selected diacids listed in this table.
c Total diacids and ω-oxoacids represent the sum of all diacids (C2–C12) and ω-oxoacids (C2–C9), respectively, identified by the GC analysis. These are listed by CitationKawamura and Yasui (2005).
* Base peak.
a Assuming that one of the carboxyl groups is detected as the m/z 44 signal.
b Values in parenthesis are the relative uncertainties (i.e., ratio of the standard deviation to the average). N/A means that the m/z 39 signal is not assigned as a fragment of the compound.
REFERENCES
- Alfarra , M. R. 2004 . Insights into Atmospheric Organic Aerosols using an Aerosol Mass Spectrometer , Ph.D. thesis UK : The University of Manchester .
- Alfarra , M. R. , Coe , H. , Allan , J. D. , Bower , K. N. , Boudries , H. , Canagaratna , M. R. , Jimenez , J. L. , Jayne , J. T. , Garforth , A. A. , Li , S.-M. and Worsnop , D. R. 2004 . Characteristics of Urban and Rural Organic Particulate in the Lower Fraser Valley using Two Aerodyne Aerosol Mass Spectrometers . Atmos. Environ. , 38 : 5745 – 5758 .
- Allan , J. D. , Delia , A. E. , Coe , H. , Bower , K. N. , Alfarra , M. R. , Jimenez , J. L. , Middlebrook , A. M. , Drewnick , F. , Onasch , T. B. , Canagaratna , M. R. , Jayne , J. T. and Worsnop , D. R. 2004 . A Generalised Method for the Extraction of Chemically Resolved Mass Spectra from Aerodyne Aerosol Mass Spectrometer Data (Technical Note) . J. Aerosol Sci. , 35 : 909 – 922 .
- Alves , C. , Carvalho , A. and Pio , C . 2002 . Mass Balance of Organic Carbon Fractions in Atmospheric Aerosols . J. Geophys. Res. , 107 : 8345 doi:10.1029/2001JD000616
- DeCarlo , P. F. , Kimmel , J. R. , Trimborn , A. , Northway , M. J. , Jayne , J. T. , Aiken , A. C. , Gonin , M. , Fuhrer , K. , Horvath , T. , Docherty , K. S. , Worsnop , D. R. and Jimenez , J. L. 2006 . Field-Deployable, High-Resolution, Time-of-Flight Aerosol Mass Spectrometer . Anal. Chem. , Article 10.1021/ac061249n
- Ervens , B. , Feingold , G. , Frost , G. J. and Kreidenweis , S. M. 2004 . A Modeling Study of Aqueous Production of Dicarboxylic Acids: 1. Chemical Pathways and Speciated Organic Mass Production . J. Geophys. Res. , 109 : D15205 doi:10.1029/2003JD004387
- Fuzzi , S. , Decesari , S. , Facchini , M. C. , Matta , E. , Mircea , M. and Tagliavini , E. 2001 . A Simplified Model of the Water Soluble Organic Component of Atmospheric Aerosols . Geophys. Res. Lett. , 28 : 4079 – 4082 .
- Jayne , J. T. , Leard , D. C. , Zhang , X. F. , Davidovits , P. , Smith , K. A. , Kolb , C. E. and Worsnop , D. R. 2000 . Development of an Aerosol Mass Spectrometer for Size and Composition Analysis of Submicron Particles . Aerosol Sci. Technol. , 33 ( 1–2 ) : 49 – 70 .
- Jimenez , J. L. , Jayne , J. T. , Shi , Q. , Kolb , C. E. , Worsnop , D. R. , Yourshaw , I. , Seinfeld , J. H. , Flagan , R. C. , Zhang , X. , Smith , K. A. , Morris , J. W. and Davidovits , P. 2003 . Ambient Aerosol Sampling using the Aerodyne Aerosol Mass Spectrometer . J. Geophys. Res. , 108 : 8425 doi:10.1029/2001JD001213
- Kawamura , K. and Ikushima , K. 1993 . Seasonal Changes in the Distribution of Dicarboxylic Acids in the Urban Atmosphere . Environ. Sci. Technol. , 27 : 2227 – 2235 .
- Kawamura , K. and Yasui , O. 2005 . Diurnal Changes in the Distribution of Dicarboxylic Acids, Ketocarboxylic Acids and Dicarbonyls in the Urban Tokyo Atmosphere . Atmos. Environ. , 39 : 1945 – 1960 .
- Kondo , Y. , Miyazaki , Y. , Takegawa , N. , Miyakawa , T. , Weber , R. J. , Jimenez , J. L. , Zhang , Q. and Worsnop , D. R. 2007 . Oxygenated and water-soluble organic aerosols in Tokyo . J. Geophys. Res. , 112 : D01203 doi:10.1029/2006JD007056
- Mochida , M. , Kawabata , A. , Kawamura , K. , Hatsushika , H. and Yamazaki , K. 2003 . Seasonal Variation and Origins of Dicarboxylic Acids in the Marine Atmosphere over the Western North Pacific . J. Geophys. Res. , 108 ( D6 ) : 4193 doi:10.1029/2002JD002355
- Morino , Y. , Kondo , Y. , Takegawa , N. , Miyazaki , Y. , Kita , K. , Komazaki , Y. , Fukuda , M. , Miyakawa , T. , Moteki , N. and Worsnop , D. R. 2006 . Partitioning of HNO3 and Particulate Nitrate over Tokyo: Effect of Vertical Mixing . J. Geophys. Res. , 111 : D15215 doi:10.1029/2005JD006887
- Ooki , M. , Oosawa , T. , Tanaka , M. and Chihara , H. , eds. 1994 . Chemical Dictionary , Tokyo , , Japan : Tokyo Kagaku Dozin, Co., Ltd. . in Japanese
- Ray , J and McDow , S. R. 2005 . Dicarboxylic Acid Concentration Trends and Sampling Artifacts . Atmos. Environ. , 39 : 7906 – 7919 .
- Rogge , W. F. , Hildemann , L. M. , Mauzrek , M. A. , Cass , G. R. and Simoneit , B. R. T. 1993 . Sources of Fine Organic Aerosol. 2. Noncatalyst and Catalyst-Equipped Automobiles and Heavy-Duty Diesel Trucks . Environ. Sci. Technol. , 27 : 636 – 651 .
- Saxena , P. and Hildemann , L. M. 1996 . Water-Soluble Organics in Atmospheric Particles: A Critical Review of the Literature and Application of Thermodynamics to Identify Candidate Compounds . J. Atmos. Chem. , 24 : 57 – 109 .
- Saxena , P. , Hildemann , L. M. , McMurry , P. H. and Seinfeld , J. H. 1995 . Organics Alter Hygroscopic Behavior of Atmospheric Particles . J. Geophys. Res. , 100 : 18,755 – 18,770 .
- Takegawa , N. , Miyazaki , Y. , Kondo , Y. , Komazaki , Y. , Miyakawa , T. , Jimenez , J. L. , Jayne , J. T. , Worsnop , D. R. , Allan , J. D. and Weber , R. J. 2005 . Characterization of an Aerodyne Aerosol Mass Spectrometer (AMS): Intercomparison with Other Aerosol Instruments . Aerosol Sci. Technol. , 39 : 760 – 770 .
- Takegawa , N. , Miyakawa , T. , Kondo , Y. , Jimenez , J. L. , Zhang , Q. , Worsnop , D. R. and Fukuda , M. 2006a . Seasonal and Diurnal Variations of Submicron Organic Aerosol in Tokyo Observed using the Aerodyne Aerosol Mass Spectrometer . J. Geophys. Res. , 111 : D11206 doi:10.1029/2005JD006515
- Takegawa , N. , Miyakawa , T. , Kondo , Y. , Blake , D. R. , Kanaya , Y. , Koike , M. , Fukuda , M. , Komazaki , Y. , Miyazaki , Y. , Shimono , A. and Takeuchi , T. 2006b . Evolution of Submicron Organic Aerosol in Polluted Air Exported from Tokyo . Geophys. Res. Lett. , 33 : L15814 doi:10.1029/2006GL025815
- Turpin , B. J. and Lim , H. J. 2001 . Species Contributions to PM2.5 Mass Concentrations: Revisiting Common Assumptions for Estimating Organic Mass . Aerosol Sci. Technol. , 35 : 602 – 610 .
- Turpin , B. J. , Saxena , P. and Andrews , E. 2000 . Measuring and Simulating Particulate Organics in the Atmosphere: Problems and Prospects . Atmos. Environ. , 34 : 2983 – 3013 .
- Zhang , Q. , Canagaratna , M. R. , Jayne , J. T. , Worsnop , D. R. and Jimenez , J. L. 2005a . Time-and Size-Resolved Chemical Composition of Submicron Particles in Pittsburgh: Implications for Aerosol Sources and Processes . J. Geophys. Res. , 110 : D07S09 doi:10.1029/2004JD004649
- Zhang , Q. , Alfarra , M. R. , Worsnop , D. R. , Allan , J. D. , Coe , H. , Canagaratna , M. R. and Jimenez , J. L. 2005b . Deconvolution and Quantification of Hydrocarbon-Like and Oxygenated Organic Aerosols Based on Aerosol Mass Spectrometry . Environ. Sci. Technol. , 39 : 4938 – 4952 . doi:10.1021/ es048568l