Abstract
Raman microspectroscopy and mapping have been applied for the analysis of soot, humic-like substances (HULIS) and inorganic compounds in size resolved samples of air particulate matter collected with an electrical low pressure impactor (ELPI). Using several reference materials, we found that spectral parameters determined by curve fitting with five bands (G, D1-D4) enable a discrimination of soot and HULIS and provide information about the relative abundance and structural order of graphite-like carbon. In particular, the D1 band width exhibited a near-linear negative correlation with the ratio of apparent elemental carbon to total carbon in different types of soot.
The ELPI samples of sub-micrometer atmospheric particles exhibited essentially the same Raman spectra and parameters as standard diesel soot. In winter samples this was also the case for larger particles with aerodynamic diameters up to 4 μm. Spring and autumn samples, however, exhibited increased D1 band widths and D3 band intensities, indicating a high prevalence of HULIS in the size range of 2–4 μm. In addition, various nitrates and sulfates (mostly NaNO3 and (NH4)2SO4; some NH4NO3, Ca(NO3)2, Na2SO4, and CaSO4) and small amounts of CaCO3 were detected. Different single- and multi-component spectra indicated the presence of externally and internally mixed particles. The relative abundance of different chemical components in different particle size ranges was quantified in mapping experiments (0–55% NaNO3, 1–15% (NH4)2SO4, 10–45% soot/HULIS, 30–60% highly fluorescent organics). Overall, the results of this study demonstrate that Raman microspectroscopy and mapping can provide qualitative and quantitative information about the composition of ELPI aerosol samples.
INTRODUCTION
Aerosols are of central importance for atmospheric chemistry and physics, climate, and public health. Atmospheric particles scatter and absorb solar and terrestrial radiation, they are involved in the formation of clouds and precipitation, and they affect the abundance and distribution of trace gases by heterogeneous chemical reactions and other multiphase processes. Moreover, airborne particles play an important role for the spread of biological organisms, reproductive materials, and pathogens (pollen, bacteria, spores, viruses, etc.), and they can cause or enhance respiratory, cardiovascular, infectious, and allergic diseases (CitationSeinfeld and Pandis 1998; CitationFinlayson-Pitts and Pitts 1999; CitationBernstein et al. 2004; CitationKanakidou et al. 2005; CitationOberdorster et al. 2005; CitationLohmann and Feichter 2005; CitationPöschl 2005; CitationFuzzi et al. 2006; CitationMcFiggans et al. 2006).
Among the predominant chemical components determining the properties and effects of air particulate matter (PM) are sulfates, nitrates, sea salt, organic compounds, and so-called black or elemental carbon, respectively. Each of these substance classes typically accounts for about 10–30% of total PM mass concentrations in the lower atmosphere, which are usually in the range of 1–100 μ g m−3. For different locations, times, meteorological conditions, and particle size fractions, however, the relative abundance of different chemical components can vary by an order of magnitude or more, and their identification and quantification are demanding analytical challenges (CitationNiessner 1991; CitationPöschl 2003, Citation2005).
Traditionally the total carbon (TC) content of air particulate matter is defined as the sum of all carbon contained in the particles, except in the form of inorganic carbonates. TC is usually determined by thermochemical oxidation and evolved gas analysis (CO2 detection), and divided into an organic carbon (OC) fraction and a black carbon (BC) or elemental carbon (EC) fraction, respectively. Measurements of BC and EC are generally based on optical and thermochemical techniques, and OC is operationally defined as the difference between TC and BC or EC, respectively (CitationGelencser 2004). In practice, however, there is no sharp cut but a continuous decrease of thermochemical refractiveness and specific optical absorption going from graphite-like structures to nonrefractive and colorless organic compounds, respectively (CitationPöschl 2003, Citation2005). Both, BC and EC, comprise the carbon content of the graphite-like material usually contained in soot (technically defined as the black product of incomplete hydrocarbon combustion or pyrolysis) and other combustion aerosol particles, which can be pictured as more or less disordered stacks of graphene layers or large polycyclic aromatics (CitationHomann 1998; CitationSadezky et al. 2005). Depending on the applied optical or thermochemical methods (absorption wavelength, temperature gradient, etc.), however, BC and EC measurements also include the carbon content of colored and refractory organic compounds, such as humic-like substances (CitationGraber and Rudich 2006). Therefore, optically determined BC values have to be considered as mass equivalent values but not as absolute mass or concentration values, and thermochemically determined EC values can only be considered as apparent elemental carbon (CitationPöschl 2003, Citation2005). Accordingly, CitationAndreae and Gelencser (2006) have recently proposed to use the symbols BCe, with subscript e for equivalent, and ECa, with subscript a for apparent, when reporting BC or EC measurement values. Several studies have compared different optical and thermochemical methods for the determination of BC and EC in atmospheric aerosol samples and found strong deviations (up to factors of 2 or more) (CitationHitzenberger et al. 1999; CitationSchmid et al. 2001; CitationWittmaack 2005). Among the few methods available for the characterization of the molecular and crystalline structures of BC and EC are high-resolution electron microscopy, X-ray diffraction, and Raman spectroscopy (CitationSu et al. 2004; CitationSadezky et al. 2005). So far, however, these methods have been too labor-intensive for routine investigations of atmospheric aerosol samples, and their applicability and reliability for quantitative analyses remains to be proven (CitationSadezky et al. 2005).
Raman spectroscopy provides fingerprint spectra which allow the distinction of a wide range of chemical substances, including soot, related carbonaceous materials, and inorganic salts in aerosol samples (CitationRosen and Novakov 1977; CitationMcCreery 2000; CitationEscribano et al. 2001; CitationSze et al. 2001; CitationMertes et al. 2004; CitationIvleva et al. 2005; CitationSadezky et al. 2005). Raman microspectroscopy (RM), which combines the analytical capabilities of Raman spectroscopy with the spatial resolution of an optical microscope, enables investigation of individual aerosol particles (CitationBatonneau et al. 2006; CitationPotgieter-Vermaak and Van Grieken 2006). CitationPotgieter-Vermaak and Van Grieken (2006) have shown that inorganic particles can be efficiently analyzed by RM, but organic particles and heterogeneous agglomerates proved to be challenging. CitationBatonneau et al. (2006) found that the results of Raman microscopic mapping of major chemical components in aerosol particles are in good agreement with elemental images obtained by wavelength-dispersive X-ray spectrometry.
In recent studies we have shown that spectral parameters obtained by RM and curve fitting with five bands (G, D1-D4) provide information about the chemical structure and reactivity of different types of soot and related carbonaceous materials (CitationSadezky et al. 2005; CitationIvleva et al. 2007). Here we apply RM for the investigation of atmospheric aerosol particles in size-resolved samples collected with an electrical low-pressure impactor. We explore the potential of this technique for qualitative and quantitative analysis of soot, humic-like substances, nitrates, and sulfates in air particulate matter.
METHODS
Atmospheric Aerosol Sampling with an Electrical Low Pressure Impactor
Atmospheric aerosol samples were collected at the Meteorological Observatory Hohenpeissenberg (MOHp), situated in a rural region at the foothills of the Alps in Southern Germany (47° 48′ N, 11° 07′ E, 980 m a.s.l.). The observatory is operated by the German Weather Service (DWD) and serves as a regional station in the Global Atmospheric Watch (GAW) program. The aerosol particles were sampled with an electrical low pressure impactor (ELPI, Model EFS1 90 Nr. 90114, Dekati Ltd., Tampere, Finland (CitationELPI 2003)). The ELPI was operated with an aerosol flow of 30 lpm and equipped with a pre-impactor (cut-off diameter 10 μ m), 11 impaction stages (cut-off diameters 28 nm to 4 μ m), and a filter stage (7–28 nm) as detailed in . The aluminum foils used as particle impaction and sample substrates on the impactor stages were cut from regular household aluminum and cleaned by rinsing with high-purity isopropanol and drying at 373 K for 3 hours. Prior to use and after sample collection they were stored in clean filter sample holders in a refrigerator. To minimize particle bounce effects, the aerosol flow was humidified to about 75% relative humidity in a moisturizing chamber installed between the sample inlet and the ELPI. The moisturizing chamber was developed at the Institute of Hydrochemistry (TU Munich, Germany [CitationZerrath 2005]). The aerosol sample inlet was positioned about 1 m from the outside wall of the observatory and 10 m above ground. It consisted of an aluminum funnel with downward-pointing aperture connected to a steel liner (1 cm diameter, 3 m length). The steel liner, moisturizing chamber and ELPI were connected by short pieces of antistatic rubber tubing (Tygon, Norton). The aerosol flow and low pressure required to operate the ELPI were maintained by a scroll pump (GVSP30, BocEdwards, West Sussex, Great Britain).
The three sets of impaction substrates investigated in this study have been collected over the periods of 5–14 May, 16–24 September, and 22–30 December 2003. RM analyses were performed on impaction substrates from stages 1–10. The substrates from impaction stage 11 and the filter stage were not suitable for RM analysis (insufficient homogeneity of deposition spots on stage 11; continuous operation of filter stage). Exemplary aerosol particle mass size distribution data are given in for the sampling period of 5–14 Mai 2003: mean mass concentration in the sampled air and mass of particulate matter deposited on the ELPI stages. These values have been calculated on the basis of the electrical charge carried by the deposited particles and corresponding electrical current measured by the ELPI electrometers, assuming an average particle density of 1.5 g/cm3 (ELPIVI software, version 4.0 rev. 615). The mass size distribution exhibited a pronounced primary maximum in the fine particle range at ∼ 0.5 μ m (stage 6, 0.39–0.62 μ m) and a secondary maximum in the coarse particle range at about 5–10 μ m (stage 11, 4–10 μ m), which are typical for rural continental aerosols (CitationSeinfeld and Pandis 1998). Over 90% of the total mass of collected air particulate matter resided in the size range of 0.15–10 μ m (stages 4–11).
TABLE 1 ELPI stage cut-off diameters and exemplary mass size distribution of air particulate matter collected on 5–14 May 2003: Da,50% is the aerodynamic diameter of particles that are deposited with 50% efficiency on a given stage, i.e., the lower cut-off diameter of the size range of particles deposited on this stage (CitationELPI 2003)
Reference Samples for Soot and Humic-Like Substances
Five reference materials for soot and humic-like substances have been investigated: graphite powder (C > 99.0%, particle size 1–2 μ m; Fluka Chemie GmbH, Steinheim, Germany), Printex XE-2 soot (Degussa AG, GB FP, Frankfurt/Main, Germany), NIST 1650 diesel particulate matter (National Institute of Standards & Technology; Gaithersburg, MD, USA), GfG spark discharge soot (Palas GFG1000 aerosol generator; CitationMesserer et al. 2006), and humic acid (humic acid depur; Carl Roth GmbH, Karlsruhe, Germany). The total carbon (TC) and apparent elemental carbon (ECa) contents of the reference materials have been determined with a thermochemical carbon analyzer (Coulomat 702, Stroehlein, Kaarst, Germany) following the VDI 2465 standard method. TC was measured by combustion of sample aliquots under O2 at 873 K and detection of the evolved CO2 by coulometric detection. For the determination of ECa, the sample aliquots were pre-conditioned by solvent extraction and thermal desorption prior to combustion (VDI, 1996). For soot, the thermochemically determined ratio of ECa/TC can be regarded as a proxy for the proportion of graphite-like carbon in the sample (graphite: ECa/TC ≈ 100%). For humic acids and humic-like substances, the ECa/TC ratio indicates proportion of carbonaceous material which has similar thermochemical refractiveness as the graphite-like material in soot (CitationPöschl 2005; CitationAndreae and Gelencser 2006; CitationGraber and Rudich 2006). Samples for Raman microspectroscopy were prepared by pressing the powdered materials in a dense layer of about one millimeter thickness on silicon wafer substrates.
Acquisition and Analysis of Raman Spectra
Spectra were recorded with a Raman microscope system (Renishaw 2000, Renishaw, UK), which consisted of an argon ion excitation laser (17 mW laser at 514 nm), an optical microscope with × 5, × 20, × 50, and × 100 magnification objectives, a holographic notch filter, and a spectrograph in combination with a thermoelectric cooled CCD detector (578 × 385 pixel). Wavelength calibrations were performed with a silicon wafer (1 s integration time, ×50 objective) by utilizing the first order phonon band of Si at 520 cm−1. The aluminum foils, used as aerosol particle collection substrates, showed no significant Raman signals in the relevant spectroscopic range (800–2000 cm−1). Spectra of the aerosol samples were recorded over the range of 800–2000 cm−1 (Stokes shift) with a spectral resolution of ∼6 cm−1, 30 s or 50 s integration time, and two different laser-microscope configurations: (a) 50× magnification objective, fully focused laser beam (diameter ≈ 1–2 μ m), and 10% of the source power; (b) 50× magnification objective, fully focused laser beam, and 100% of the source power.
In the computer-controlled Raman mapping procedure spectra were recorded in an automated point-by-point scanning mode: static mode centered at 1250 cm−1 (spectroscopic range 970–1500 cm−1) with 3 s dwell time (photo-bleaching for fluorescence reduction prior to data collection) and 2 s integration time, 50× magnification objective, fully focused laser beam, and 100% source power. The instrument's autofocus option was applied to automatically adjust the laser focus on the sample surface. The mapping was performed in 50 μ m steps over a square area around the center of the particle deposition spots on the impaction substrates (different sizes of particle deposition spots on different impaction stages): 1300 × 1300 μ m on stage 10; 1000 × 1000 μ m on stage 9; 800 × 800 μ m on stage 8; and 700 × 700 μ m on stage 7.
All spectra were processed via WiRE 1.2 software (Renishaw, UK) running under GRAMS/32 (Thermo Galactic, USA), that were used to control the Raman microscope system and data acquisition as well as the processing and analysis (curve fitting) of Raman spectra. Spectral analyses were performed by comparison with spectra from an in-house library as well as commercially available spectral libraries (CitationDegen and Newman 1993; CitationNyguist et al. 1997). Spectra with fluorescence background signals of 30000 counts or more were affected by partial or complete saturation of the CCD detector and categorized as “high fluorescence” spectra.
Spectral parameters of soot, related carbonaceous materials, and humic-like substances were determined by curve fitting after multi-point baseline correction with the GRAMS software. The spectra were fitted with a combination of four Lorentzian-shaped G, D1, D2, and D4 bands at about 1580, 1350, 1620, and 1200 cm−1, respectively, with Gaussian-shaped D3 band at about 1500 cm−1 (CitationSadezky et al. 2005). The goodness-of-fit was indicated by the χ2 value (which is equal to unity for ideal fit). Values between 1 and 3 imply that the fit converges towards the observed spectrum. For the analysis presented here, we used the results with χ2 < 2. For each Raman spectrum, the fitting procedure was repeated at least six times to ensure the reproducibility of fit. The standard deviations of parameters from repeated fits were typically less than 3%. The mean values and standard deviations of the spectral parameters presented and discussed below were obtained from 5–10 spectra recorded at different positions on the sample.
Interpretation of Raman Spectra for Soot and Related Carbonaceous Materials
The first-order Raman spectra of soot and related carbonaceous materials are generally characterized by two broad and strongly overlapping peaks with intensity maxima near 1580 (G or “Graphite” peak) and 1350 cm−1 (D or “Defect” peak). Based on experimental observations and theoretical calculations, up to five bands corresponding to different vibration modes of crystalline or molecular structures in the sample material have been suggested to account for the observed spectra (CitationSze et al. 2001; CitationDippel et al. 1999; CitationSadezky et al. 2005). The G band at ∼1580 cm−1 has been attributed to ideal graphitic lattice (E2g symmetry) stretching mode (CitationTuinstra and König 1970; CitationFerrari and Robertson 2000; CitationSadezky et al. 2005). Other bands contributing to the observed spectrum, are typical for disordered graphitic lattices (D bands). The D1 band at ∼1350 cm−1 has been associated with a vibration mode involving graphene layer edges (A1g symmetry) (CitationTuinstra and König 1970; CitationFerrari and Robertson 2000; CitationSadezky et al. 2005), and the D2 band at ∼1620 cm−1 has been attributed to a vibration mode involving surface graphene layers (E2g symmetry) (CitationFerrari and Robertson 2000; CitationSadezky et al. 2005). High signal intensities between the two main peaks of the observed spectra have been assigned to a D3 band at ∼ 1500 cm−1 that is associated with the amorphous carbon content of soot (organic molecules, fragments, and functional groups [CitationCuesta et al. 1994; CitationJawhari et al. 1995; CitationBeyssac et al. 2003; CitationDippel et al. 1999; CitationSadezky et al. 2005]). Moreover, weak bands at ∼ 1200 cm−1 (D4) have been assigned to vibrations of disordered graphite lattices (A1g symmetry), sp2- and sp3-hybridized carbon bonds, C–C and C = C stretching vibrations of polyenes, and ionic impurities (CitationCuesta et al. 1994; CitationDippel et al. 1999; CitationSadezky et al. 2005).
For structural characterization of soot and related carbonaceous materials, a range of different spectroscopic parameters and curve fitting procedures have been proposed (CitationJawhari et al. 1995; CitationSze et al. 2001; CitationBeyssac et al. 2003; CitationDippel et al. 1999; CitationSadezky et al. 2005). Jawhary et al. (1995) have used two Lorentzian (G′ and D1) and one Gaussian band (D3) for several carbon black materials and reported that the G′ band intensity is related to the abundance of the crystalline component, that the D1 band width reflects the degree of structural order in the samples, and that I D1 /I G′ is inversely proportional to the lateral extension of microcrystalline domains. CitationSze et al. (2001) have used three Lorentzian bands (G, D1, and D2) and reported that the integrated band intensity ratios I D1 /I G (or R1) and I D2 /I G provide information about the abundance of the graphitic component in carbonaceous materials. CitationDippel et al. (1999) have used an alternative combination of four Lorentzian bands for the characterization of flame soot: G′ (a single band combining G and D2 band), D1, D3, and D4. They suggested that the G′ band intensity, the D1 band width, and the I D1 /I G′ intensity ratio are related to the graphitic order. CitationMertes et al. (2004) have proposed to quantify the amount of graphitic carbon in atmospheric aerosol samples by integrating the total G peak intensity from 1510 to 1736 cm−1 (including all potential contributions of the G, D2, and D3 bands). They used industrial carbon black (Monarch 71) as a reference substance and obtained good correlations with optical absorption measurements. CitationBeyssac et al. (2003) used four Voigt-function bands (G, D1, D2, and D3) and proposed to characterize the degree of organization of heterogeneous carbonaceous materials (natural coal, cokes and anthracite) by the integrated band intensity ratio I D1 /(I G + I D1 + I D2 ) (or R2). Recently, CitationSadezky et al. (2005) have tested and compared nine different band combinations and demonstrated that the best fit to the Raman spectra of a wide range of soot samples and related carbonaceous materials was obtained with four Lorentzian bands (G, D1, D2, and D4) and one Gaussian band (D3). They reported that the D1 band width allow to discriminate an industrial soot sample with high degree of graphitic order (Degussa Printex XE2) from seven other types of industrial soot, spark discharge soot, and diesel soot.
RESULTS AND DISCUSSION
Raman Spectra and Spectral Parameters of Soot and Humic-Like Substance Reference Materials
shows characteristic Raman spectra of the investigated reference materials for soot and humic-like substances (Printex XE2 industrial soot, SRM 1650 diesel soot, GfG spark discharge soot, graphite, humic acid). They all show pronounced peaks at ∼ 1350 cm−1 (D peak) and ∼ 1580 cm−1 (G peak), but the D and G peaks exhibit greatly varying relative intensities and widths. In accordance with (CitationYang and Wang 1997), the Raman spectra of humic acid were similar to those of soot, but the fluorescence background signals were more than an order of magnitude higher than for the soot samples.
FIG. 1 Raman spectra (λ0 = 514 nm) of different soot samples and related carbonaceous materials. The spectra are offset for clarity.
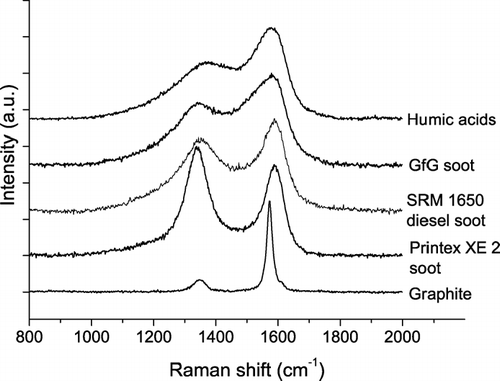
For quantitative spectral analysis we applied the five band combination and fitting procedures proposed by CitationSadezky et al. (2005) and determined the band positions (Stokes Raman shift, cm−1), full width at half maximum (FWHM, cm−1), integrated band intensities relative to the G band (I/I G ) for four Lorentzian bands (G, D1, D2, D4) and one Gaussian band (D3) as detailed above. Mean values and standard deviations of all parameters are listed in Appendix . Overall, the results obtained for graphite and different types of soot are in good agreement with those reported by CitationSadezky et al. (2005), except for substantially higher relative intensities of the D3 band (I D3 /I G ) in GfG soot.
Recently we have demonstrated that the D1 band width and the D3 band intensity are the Raman spectroscopic parameters which provide most information about the chemical structure and reactivity of different types of soot (CitationIvleva et al. 2007). This is reconfirmed by the measurement and fitting results of the present study, because only for D1 FWHM and I D3 /I G the differences between the investigated soot and humic acid reference materials were substantially lager than the standard deviations of repeated measurements (Appendix, ). Thus the subsequent discussions of Raman spectra from soot and humic-like substances are focused primarily on these spectroscopic parameters.
shows a pronounced decrease of I D3 /I G with increasing ratio of apparent elemental carbon to total carbon content (ECa/TC) of the investigated samples, which is consistent with a decrease of the amorphous organic carbon fraction and increase of graphitic structural order from GfG soot (highly disordered) to graphite (highly ordered [CitationSadezky et al. 2005; Ivleva et al. 2006; CitationMesserer et al. 2006]). A similar but less pronounced trend was observed for the relative intensity of the D1 band (I D1 /I G ). For comparison with earlier studies (CitationBeyssac et al. 2003; CitationSadezky et al. 2005; Ivleva et al. 2006), we have also calculated the parameters R2 = I D1 / (I G + I D1 + I D2 ) and R3 = I D3 / (I G + I D2 + I D3 ) and listed them in Appendix . Compared to the relative intensities of individual bands, these parameters exhibit similar correlations with ECa/TC.
FIG. 2 Integrated band intensity ratio I D3 /I G vs. apparent elemental carbon (ECa) fraction of reference materials for soot and humic-like substances (mean values ± standard deviations).
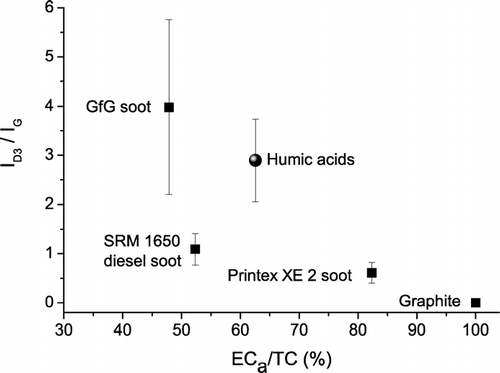
Moreover, we found that the D1 band width exhibits a near-linear negative correlation with the ECa/TC ratio of the soot and graphite samples (), which is also consistent with an increase of structural order from GfG soot to graphite (CitationSadezky et al. 2005; CitationIvleva et al. 2007; CitationMesserer et al. 2006). The close correlation between D1 FWHM and ECa/TC ratio indicates that Raman spectroscopy may serve as an efficient tool for the characterization of ECa/TC ratios and the degree of graphitic structural order in soot and related carbonaceous materials.
FIG. 3 Full widths at half maximum (FWHM) of D1 band vs. apparent elemental carbon (ECa) fraction of reference materials for soot and humic-like substances (mean values ± standard deviations; linear fit to graphite and soot samples).
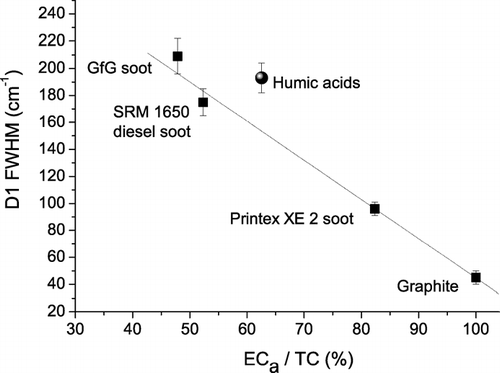
The Raman spectroscopic parameters of the humic acid reference material, including D1 FWHM and I D3 /I G , were similar to those of GfG soot, although the ECa/TC ratio was significantly higher ( and ). The observation of higher ECa/TC ratios for humic acid than for GfG and standard diesel soot can be attributed to charring effects, which convert organic molecules of high molecular mass into disordered graphite-like structures (charcoal) and represent a major problem in the thermochemical analysis of atmospheric aerosol samples containing humic-like substances and biopolymers (artificial overestimation of the “graphite-like,” “black,” or “elemental” carbon content (CitationPöschl 2005; CitationAndreae and Gelencser 2006).
In any case, and illustrate that both the D1 band width and the relative intensity of the D3 band of humic acid are on average higher than those of standard diesel soot and may thus be applicable for the distinction of different types of soot and humic-like substances in air particulate matter by Raman microspectroscopy.
Raman Spectra and Spectral Parameters of Soot and Humic-Like Substances in ELPI Samples of Air Particulate Matter
The Raman spectra of samples of air particulate matter usually exhibited varying fluorescence background and signal-to-noise levels, but after baseline correction they could be systematically analyzed by curve-fitting and compared to the spectra of reference materials.
shows exemplary Raman spectra of soot or humic-like substances from different ELPI stages and particle size ranges (stages 2–10; cut-off diameters 56 nm to 2.4 μ m) of the atmospheric aerosol samples collected on 5–14 May 2003. At first sight the base-line corrected spectra look all very similar. Nevertheless, close inspection reveals that the shape of the spectra from stages 2–8 is nearly identical to that of SRM 1650 diesel soot, whereas the shape of the spectrum from stage 10 resembles more closely to that of humic acid (see ).
FIG. 4 Exemplary Raman spectra (λ0 = 514 nm) of soot or humic-like substances in air particulate matter collected in May 2003 on ELPI stages 2, 4, 6, 8, and 10. The spectra are offset for clarity.
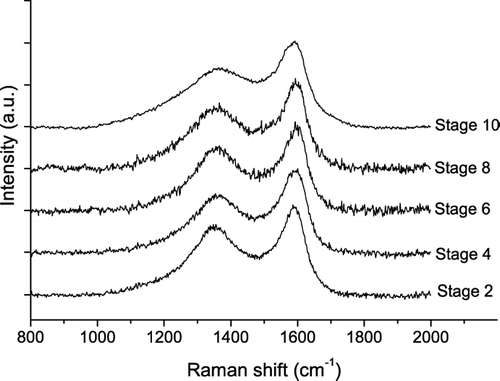
illustrates the results of curve fitting for the exemplary spectra of stages 4 and 10 from : it shows a higher width of the D1 band and a higher relative intensity of the D3 band for stage 10. Indeed D1 FWHM and I D3 /I G (203 ± 3 cm−1; 1.9 ± 0.1) of the spectrum from stage 10 are in the range of values typical for humic acid (193 ± 11 cm−1; 2.9 ± 0.8), whereas the values obtained for stage 4 (183 ± 6 cm−1; 1.0 ± 0.1) are close to the mean values of diesel soot (175 ± 10 cm−1; 1.1 ± 0.3). Thus the exemplary spectra appear to originate from humic-like substances on stage 10 and from diesel soot on stage 4.
FIG. 5 Exemplary Raman spectra (λ0 = 514 nm) of soot or humic-like substances in air particulate matter collected in May 2003 on ELPI stages 10 (a, humic-like) and 4 (b, soot-like) with five band fits.
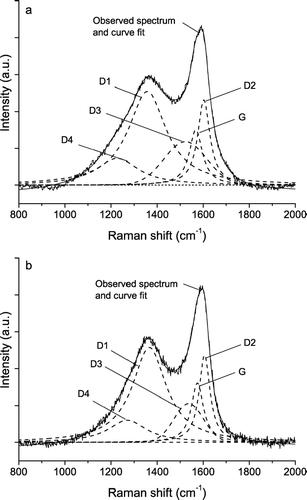
and display the mean values and standard deviations of D1 FWHM and I D3 /I G obtained from 7–10 different spectra recorded at randomly varying positions on the aerosol particle samples collected on ELPI stages 1–10 in May 2003 (complete data set: Appendix ). For stage 10 the mean values of D1 FWHM and I D3 /I G are clearly outside the range of variability observed for standard diesel soot and close to the mean values observed for humic acid, indicating a prevalence of humic-like substances in the size range of 2.4–4 μ m. In contrast, the range of spectral parameters for stages 1–7 is largely overlapping with that of standard diesel soot, consistent with a prevalence of diesel soot throughout the sub-micrometer size range (28–957 nm). In the intermediate size range of 1–2.4 μ m (stages 8 and 9), the spectral parameters of air particulate matter vary between those of diesel soot and humic acid reference materials.
FIG. 6 Full widths at half maximum (FWHM) of D1 band vs. ELPI stage number of aerosol particle samples collected in May (a), September (b), and December 2003 (c) (mean values ± standard deviations).
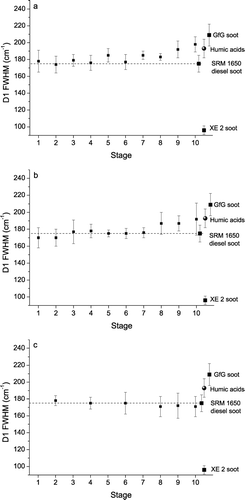
FIG. 7 Integrated band intensity ratio I D3 /I G vs. the ELPI stage number for aerosol particles collected in May (a), September (b), and December 2003 (c) (mean values ± standard deviations).
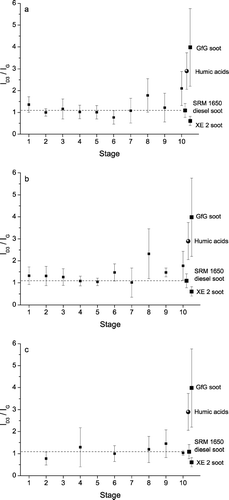
and show similar pictures for the ELPI samples collected in September 2003 (complete data set: Appendix ). In contrast, the spectral parameters from the ELPI samples collected in December 2003 ( and ) exhibit no dependence on particle size and are all in good agreement with the standard diesel soot values (complete data set: Appendix ), indicating that humic-like substances are much less abundant in the sampled winter-time aerosols.
Overall, , , , demonstrate that the shape of Raman spectra and the D1 band widths and D3 band intensities derived by curve fitting can indeed be used to distinguish soot and humic-like substances in size-resolved ELPI samples of air particulate matter by Raman microspectroscopy.
Raman Spectra of Inorganic Compounds in ELPI Samples of Air Particulate Matter
shows exemplary Raman spectra of nitrates and sulfates from different ELPI stages and particle size ranges (stages 6–10; cut-off diameters 0.39–2.4 μ m) of the atmospheric aerosol samples collected on 5–14 May 2003. On stage 10 (2.4–4 μ m; ) most of the detected Raman signals are characteristic for nitrates: sodium nitrate at 1067 cm−1 (dry) and 1052 cm−1 (wet), calcium nitrate at 1049 cm−1, and ammonium nitrate at 1042 cm−1. On stage 9 (1.6–2.4 μ m; ) we could identify a range of different sulfates in addition to calcium and sodium nitrate: ammonium sulfate (wet) at 974 cm−1 (CitationBatonneau et al. 2006); contributions of ammonium and potassium sulfites at 973 cm−1 and 975 cm−1 possible), sodium sulfate at 995 cm−1, and calcium sulfate (gypsum) at 1006 cm−1. On stage 8 (0.96–1.6 μ m; ) we found no signals of nitrates but characteristic bands of calcium carbonate (calcite, 1086 cm−1), calcium sulfate (gypsum, 1006 cm−1), sodium sulfate (995 cm−1), and ammonium sulfate at 974 cm−1 (wet) and 982 cm−1 (dry, [CitationBatonneau et al. 2006]; contributions of potassium and lead sulfates at 980 cm−1 and 983 cm−1 possible). On stage 7 (0.62–0.96 μ m; ) we found ammonium and sodium sulfate signals (974 cm−1, 982 cm−1, 995 cm−1), and on stage 6 (0.39–0.62 μ m; ) as well as on lower stages we found only the ammonium sulfate band at 974 cm−1 ().
FIG. 8 Exemplary Raman spectra (λ0 = 514 nm) of inorganic compounds in air particulate matter collected in May 2003 on ELPI stages 10 (a), 9 (b), 8 (c), 7 (d), and 6 (e).
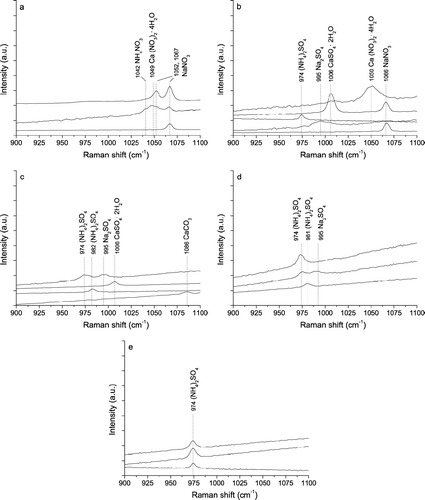
The strong prevalence of nitrate signals in spectra from stages 10 and 9 confirms that nitrates are highly abundant in micrometer-sized particles (diameter range 1.6–4 μ m) of rural aerosols (CitationSeinfeld and Pandis 1998; CitationFinlayson-Pitts and Pitts 1999). Their absence from lower stages may be due not only to a lower abundance in sub-micrometer atmospheric particles but also to evaporative losses (CitationZhang and McMurry 1992) in the low-pressure impactor (pressure decrease with decreasing stage number and cut-off diameter).
The enhanced prevalence of signals related to calcium and sodium salts on stages 10–8 (minor signals of sodium sulfate on stage 7, no calcium- or sodium-related signals detected at lower stages) confirms that the abundance of these elements in rural air particulate matter is dominated by micrometer-sized dust particles or aged sea-salt particles (CitationSeinfeld and Pandis 1998; CitationFinlayson-Pitts and Pitts 1999).
The dominance of ammonium sulfate signals on stage 7 and lower stages confirms that ammonium sulfate in rural aerosols is concentrated in sub-micrometer particles and mostly of secondary origin (oxidation of SO2 to H2SO4, neutralization by NH3, and condensation on pre-existing or newly formed fine particles (CitationSeinfeld and Pandis 1998; CitationFinlayson-Pitts and Pitts 1999]).
As some of the exemplary spectra exhibit signals from multiple compounds whereas others are characteristic for only one compound, the results of Raman microspectroscopy also provide information about the mixing state of air particulate matter (internally vs. externally mixed aerosols). The observation of different spectra generally indicates the presence of particles with different composition (external mixture). Signals from multiple compounds in one spectrum suggest that the investigated particles are composed of multiple components (internal mixture). Depending on the dimensions of the laser focal spot and investigated particles, however, different spectra may also arise from probing different regions on heterogeneous multi-component particles, and multi-component spectra may also arise from the probing of multiple adjacent particles (internally or externally mixed). Thus the number of single- and multi-component spectra can be regarded as an upper limit for the number of different types of externally mixed particles in the investigated aerosol sample, and the proportion of multi-component spectra can be regarded as an upper limit for the relative abundance of internally mixed particles.
Overall, demonstrates that Raman microspectroscopy can be applied to identify a range of three different nitrates (ammonium, calcium, and sodium nitrate), three different sulfates (ammonium sulfate, calcium, and sodium sulfate), and calcium carbonate in size-resolved ELPI samples of air particulate matter, and that it provides information about the aerosol particle mixing state (internal vs. external mixtures).
Raman Mapping for Quantitative Analysis of ELPI Samples of Air Particulate Matter
To obtain quantitative information about the composition of the investigated samples, we have applied computer-controlled Raman mapping as detailed in a previous section. and summarize the results obtained upon investigation of stages 7–10 of the ELPI samples of rural air particulate matter collected in May 2003. On each stage 3–5 particle deposition spots have been mapped, and the total number of spectra recorded per spot ranged from 225 to 729. The sum of all spectra with signals characteristic for nitrates, sulfates, soot/HULIS, or high fluorescence (“characteristic spectra”) and their proportions were determined. The relative standard deviations between the different spots investigated on a given stage were mostly less than 30%, indicating fairly uniform composition and reasonable counting statistics. The ratio of the total number of characteristic spectra over recorded spectra increased from about 10% for the particle deposition spots on stage 10 to 50% on stage 7. This is consistent with an increase of particle number concentration with decreasing particle size that is typical for atmospheric aerosols and with microscopic images of the deposition spots.
FIG. 9 The proportion of the Raman spectra (λ0 = 514 nm) with signals characteristic for nitrates, sulfates, soot/HULIS, and high fluorescence in air particulate matter collected in May 2003 on ELPI stages 10–7.
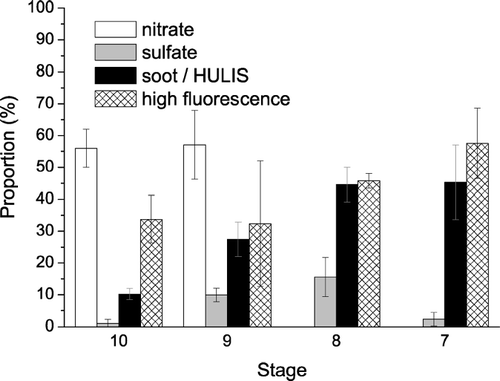
TABLE 2 Raman mapping of ELPI samples collected on 5–14 May 2003 (stages 7–10): investigated area, number of particle deposition spots investigated; number of spectra recorded; ratio of the number of all spectra with signals characteristic for nitrates, sulfates, soot/HULIS, or high fluorescence (“characteristic spectra”) to the number of recorded spectra; proportion of different types of characteristic spectra relative to the number of all characteristic spectra (arithmetic mean values ± standard deviations from averaging over different particle deposition spots)
The proportion of spectra with signals of soot/HULIS relative to the total number of characteristic spectra increased from ∼ 10% on stage 10 to ∼ 45% on stage 7, which is consistent with increasingly darker color of the deposition spots. The observed proportions are in good agreement with the results of CitationPotgieter-Vermaak and Van Grieken (2006) who reported that 50% of atmospheric particles with ∼ 1 μ m diameter exhibited Raman signals characteristic for soot, but only 10% of particles with ∼ 4 μ m diameter.
The proportion of spectra with sulfate signals was lowest on stage 10 (∼ 1%, calcium sulfate only) and highest on stage 8 (∼ 15%, mostly ammonium sulfate). The relatively low proportion of sulfates observed upon Raman mapping of stage 7 (∼ 2%) is not consistent with the results of recording individual spectra; it is most likely due to a masking of sulfate signals by high fluorescence as discussed below.
The proportion of spectra with nitrate signals on stages 9 and 10 was ∼ 55%, and most of these were from sodium nitrate. The high proportion of sodium nitrate can be attributed to the uptake of nitric acid and replacement of chloride by nitrate in aged sea salt particles (CitationGard et al. 1998; CitationLaskin et al. 2002; CitationKrüger et al. 2003). No nitrates were found on stages 7 and 8, which is consistent with the results of CitationBatonneau et al. (2006). They also found nitrates in size-selected aerosol filter samples with aerodynamic diameters of 1–10 μ m but not in sub-micrometer samples, which were dominated by soot and sulfate. Spectra with carbonate (calcite) signals were recorded on stages 10 and 8, but their proportions were much less than 1%.
Fluorescence signals of air particulate matter are generally due to organic components, including biological materials but also the organic components of soot (polycyclic aromatic compounds) and HULIS. They can mask characteristic fingerprint signals of other substances and limit the applicability of Raman spectroscopy for quantitative analysis.
The proportion of high fluorescence spectra increased from ∼ 30% on stage 10 to ∼ 60% on stage 7, indicating an increase of the organic particle fraction with decreasing particle size. At lower stages the proportion of high fluorescence spectra was even higher, preventing further statistical analysis. For future Raman mapping experiments we are planning to reduce fluorescence by application of longer laser excitation wavelengths (e.g., 633 nm) or longer photo-bleaching prior to the recording of Raman spectra (> 3 s). The proportion of spectra with characteristic signals for more than one component (inorganic salts, soot/HULIS, fluorescent organics) varied around 5–20%. It indicates that a major fraction of the investigated chemical components of the sampled rural air particulate matter was externally mixed.
Overall, the results of our Raman mapping experiments with ELPI samples are consistent with the results of recent RM investigations of other types of aerosol samples (CitationBatonneau et al. 2006; CitationPotgieter-Vermaak and Van Grieken 2006) and with the results of ELPI sample analysis by other techniques (CitationTemesi et al. 2001; CitationPöschl 2005; CitationZerrath 2005). They demonstrate that quantitative or at least semi-quantitative information about the composition and mixing state of size-resolved ELPI samples of air particulate matter can be obtained by Raman mapping. Thus we suggest and plan to explore the precision, accuracy, and reliability of Raman mapping for quantitative analysis of air particulate matter by further comprehensive and systematic studies.
These future studies should also address potential sampling artifacts caused by reactive trace gases like ozone and nitrogen oxides, which can be generated by the ELPI corona charger and may change the chemical composition of the aerosol particles collected on the ELPI substrates. First insights may be obtained by test experiments in which the corona charger is sequentially turned on and off. To obtain reliable and representative information about the actual occurrence and extent of ambient aerosol sampling artifacts caused by the corona charger, however, it appears necessary to operate multiple ELPI instruments in parallel with the corona charger turned on or off.
Such investigations go beyond the scope of the present manuscript, which is meant to demonstrate the applicability of Raman microspectroscopy and mapping for the analysis of aerosol particles collected on ELPI substrates. Moreover, this study was focused on the identification and distinction of soot and humic-like substances, which are generally characterized by high thermochemical refractiveness and relatively low susceptibility for oxidation by gaseous reactants. Exposure of these substances to ozone and nitrogen oxides at ambient temperatures is likely to change their surface composition and content of organic trace substances but not their bulk composition and spectral parameters determined by Raman microspectroscopy (CitationPöschl et al. 2001; CitationSchauer et al. 2003; CitationSchauer et al. 2004; CitationPöschl, 2005; CitationMesserer et al. 2006; CitationIvleva et al. 2007).
SUMMARY AND CONCLUSIONS
In this study we have investigated and demonstrated the applicability of Raman microspectroscopy and Raman mapping for the identification and semi-quantitative analysis of soot, humic-like substances, nitrates, sulfates, and carbonate in size resolved samples of air particulate matter collected with an electrical low-pressure impactor.
The characterization of five reference materials for soot and humic-like substances (graphite, Printex XE-2 soot, NIST 1650 diesel particulate matter, GfG spark discharge soot, humic acid) showed that spectral parameters determined with a five band fitting procedure—in particular the D1 band width and the D3 band intensity—provide information about the relative abundance and structural order of graphite-like and amorphous carbon in the samples. We found that the D1 bandwidth exhibits a near-linear negative correlation with the ECa/TC ratio of the investigated soot and graphite samples, which is consistent with the increase of structural order from GfG soot to graphite and demonstrates that Raman microspectroscopy can serve as an efficient tool for the characterization of ECa/TC ratios. Both the D1 bandwidth and the relative intensity of the D3 band of humic acid were on average higher than those of standard diesel soot.
The spectral parameters of the ELPI samples of air particulate matter varied between those of standard diesel soot and humic acid. The sub-micrometer particles (30–960 nm, ELPI stages 1–7) exhibited essentially the same Raman spectra and parameters as standard diesel soot, which confirms that the ECa and BCe content of fine particulate matter in continental air of Central Europe is dominated by soot from fossil fuel combustion or similar carbonaceous particles from other pyrogenic sources (e.g., biomass burning). The Raman spectra of ELPI samples collected in winter exhibited no dependence on particle size, i.e., also the larger particles with diameters up to 4 μ m showed the same spectral parameters as standard diesel soot. In contrast, the large particles collected in spring on ELPI stage 10 (2–4 μ m) exhibited D1 band widths and D3 band intensities which were on average clearly outside the range of variability observed for standard diesel soot. Instead, they were close to the mean values observed for humic acid, indicating a high prevalence of humic-like substances in the size range of 2–4 μ m. In the intermediate size range of 1–2 μ m (ELPI stages 8–9), the spectral parameters varied between those of standard diesel soot and humic acid, indicating a fairly even mix of soot and HULIS. The spectral characteristics of ELPI samples collected in autumn were essentially the same as those of the samples collected in spring. Overall, the results demonstrate that Raman spectra and the D1 band widths and D3 band intensities derived by curve fitting can be used to distinguish soot and HULIS in size-resolved samples of air particulate matter.
In addition to soot and humic-like substances, three different nitrates (ammonium, calcium, and sodium nitrate), three different sulfates (ammonium sulfate, calcium, and sodium sulfate), and calcium carbonate were detected and identified in the ELPI samples. The observation of single- and multicomponent spectra indicated the presence of both externally and internally mixed particles. The results of automated Raman mapping experiments were consistent with the manually recorded spectra, with recent Raman microspectroscopic investigations of other types of aerosol samples, and with the results of ELPI sample analysis by other analytical techniques. The proportion of spectra with signals characteristic for soot or HULIS increased from ∼10% for coarse particles to ∼45% for fine particles, and a similar trend was observed for sulfates (mostly (NH4)2SO4; ∼1% of coarse and ∼15% of fine particle spectra). In contrast, the proportion of spectra with nitrate signals (mostly NaNO3) was as high as ∼55% for coarse particles and dropped to zero for fine particles. Less than 1% of the spectra exhibited signals from calcite. The proportion of spectra with high fluorescence signals from organic components, including biological materials as well as HULIS and organic components of soot (polycyclic aromatic compounds), increased from ∼30% on ELPI stage 10 (2–4 μm) to ∼60% on stage 7 (0.6–1 μm), indicating an increase of the organic fraction of air particulate matter with decreasing particle size. At lower stages the proportion of high fluorescence spectra was even higher and prevented further statistical analysis, because fluorescence signals can mask characteristic fingerprint signals of other substances and limit the applicability of Raman spectroscopy for quantitative analysis. For future Raman mapping experiments we suggest and are planning to reduce fluorescence by application of longer laser excitation wavelengths or longer photo-bleaching prior to the recording of Raman spectra. The proportion of spectra with characteristic signals for more than one component (inorganic salts, soot/HULIS, fluorescent organics) varied around 5–20%. It indicates that a major fraction of the investigated chemical components of the sampled rural air particulate matter was externally mixed.
Overall, the results of this study demonstrate that Raman microspectroscopy and mapping provide qualitative and quantitative (or at least semi-quantitative) information about the composition and mixing state of size-resolved samples of air particulate matter. Thus we suggest and plan to determine the precision, accuracy, and reliability of Raman microspectroscopy and mapping for quantitative analysis of atmospheric aerosols by further comprehensive and systematic studies with a larger number of samples from different locations and seasons.
APPENDIX
TABLE A1 Spectral parameters for the first-order Raman bands of different soot samples and related carbonaceous materials (λ0 = 514 nm): number of analyzed spectra (n), band position (Stokes Raman Shift, cm−1), full width at half maximum (FWHM, cm−1), peak intensity ratios relative to the G band (arithmetic mean values ± standard deviations)
TABLE A2 Spectral parameters for the first-order Raman bands of soot aerosol particles deposited on ELPI stages in May 2003 (λ0 = 514 nm): particle cut-off diameter (Da,50%, μ m), number of analyzed spectra (n), band position (Stokes Raman Shift, cm−1), full width at half maximum (FWHM, cm−1), peak intensity ratios relative to the G band (arithmetic mean values ± standard deviations)
TABLE A3 Spectral parameters for the first-order Raman bands of soot aerosol particles deposited on ELPI stages in September 2003 (λ0 = 514 nm): particle cut-off diameter (Da,50%, μ m), number of analyzed spectra (n), band position (Stokes Raman Shift, cm−1), full width at half maximum (FWHM, cm−1), peak intensity ratios relative to the G band (arithmetic mean values ± standard deviations)
TABLE A4 Spectral parameters for the first-order Raman bands of soot aerosol particles deposited on ELPI stages in December 2003 (λ0 = 514 nm): particle cut-off diameter (Da,50%, μ m), number of analyzed spectra (n), band position (Stokes Raman Shift, cm−1), full width at half maximum (FWHM, cm−1), peak intensity ratios relative to the G band (arithmetic mean values ± standard deviations)
This work has been funded by the German Federal Ministry of Education and Research (BMBF, AFO2000 Project 07ATC05, CARBAERO). The authors thank H. Berresheim and colleagues from the German Weather Service (DWD) for providing infrastructure and support at the MOHp sampling site. Technical assistance by R. Leube and S. Mahler, supply of samples by A. Messerer and A. Zerrath, and helpful comments from two anonymous reviewers are gratefully acknowledged.
REFERENCES
- Andreae , M. O. and Gelencser , A. 2006 . Black carbon or brown carbon? The nature of light-absorbing carbonaceous aerosols . Atmos. Chem. Phys. , 6 : 3131 – 3148 .
- Batonneau , Y. , Sobanska , S. , Laureyns , J. and Bremard , C. 2006 . Confocal Microprobe Raman Imaging of Urban Tropospheric Aerosol Particles . Environ. Sci. Technol. , 40 : 1300 – 1306 .
- Bernstein , J. A. , Alexis , N. , Barnes , C. , Bernstein , I. L. , Bernstein , J. A. , Nel , A. , Peden , D. , Diaz-Sanchez , D. , Tarlo , S. M. and Williams , P. B. 2004 . Health Effects of Air Pollution . J. Allergy Clin. Immunol. , 114 : 1116 – 1123 .
- Beyssac , O. , Goffe , B. , Petitet , J.-P. , Froigneux , E. , Moreau , M. and Rouzaud , J.-N. 2003 . On the Characterization of Disordered and Heterogeneous Carbonaceous Materials by Raman Spectroscopy . Spectrochim. Acta, Part A , 59A : 2267 – 2276 .
- Cuesta , A. , Dhamelincourt , P. , Laureyns , J. , Martinez-Alonso , A. and Tascon , J. M. D. 1994 . Raman Microprobe Studies on Carbon Materials . Carbon , 32 : 1523 – 1532 .
- Degen , I. A. and Newman , G. A. 1993 . Raman Spectra of Inorganic Ions . Spectrochim. Acta, Part A , 49A : 859 – 887 .
- Dippel , B. , Jander , H. and Heintzenberg , J. 1999 . NIR FT Raman Spectroscopic Study of Flame Soot . Phys. Chem. Chem. Phys. , 1 : 4707 – 4712 .
- ELPI . 2003 . ELPI User Manual , Dekati Ltd .
- Escribano , R. , Sloan , J. J. , Siddique , N. , Sze , N. and Dudev , T. 2001 . Raman Spectroscopy of Carbon-Containing Particles . Vib. Spectrosc. , 26 : 179 – 186 .
- Ferrari , A. C. and Robertson , J. 2000 . Interpretation of Raman Spectra of Disordered and Amorphous Carbon . Phys. Rev. B: Condens. Matter Mater. Phys. , 61 : 14095 – 14107 .
- Finlayson-Pitts , B. J. and Pitts , J. N. 1999 . Chemistry of the Upper and Lower Atmosphere: Theory, Experiments, and Applications , 349 – 423 . San Diego : Academic .
- Fuzzi , S. , Andreae , M. O. , Huebert , B. J. , Kulmala , M. , Bond , T. C. , Boy , M. , Doherty , S. J. , Guenther , A. , Kanakidou , M. , Kawamura , K. , Kerminen , V. M. , Lohmann , U. , Russell , L. M. and Pöschl , U. 2006 . Critical Assessment of the Current State of Scientific Knowledge, Terminology, and Research Needs Concerning the Role of Organic Aerosols in the Atmosphere, Climate, and Global Change . Atmos. Chem. Phys. , 6 : 2017 – 2038 .
- Gard , E. E. , Kleeman , M. J. , Gross , D. S. , Hughes , L. S. , Allen , J. O. , Morrical , B. D. , Fergenson , D. P. , Dienes , T. , Gälli , M. E. , Johnson , R. J. , Cass , G. R. and Prather , K. A. 1998 . Direct Observation of Heterogeneous Chemistry in the Atmosphere . Science , 279 : 1184 – 1187 .
- Gelencser , A. 2004 . Carbonaceous aerosol , 22 – 82 . Dordrecht : Springer .
- Graber , E. R. and Rudich , Y. 2006 . Atmospheric HULIS: How Humic-Like Are They? A Comprehensive and Critical Review . Atmos. Chem. Phys. , 6 : 729 – 753 .
- Hitzenberger , R. , Jennings , S. G. , Larson , S. M. , Dillner , A. , Cachier , H. , Galambos , Z. , Rouc , A. and Spain , T. G. 1999 . Intercomparison of Measurement Methods for Black Carbon Aerosols . Atmos. Environ. , 33 : 2823 – 2833 .
- Homann , K.-H. 1998 . Fullerenes and Soot Formation—New Pathways to Large Particles in Flames . Angew. Chem., Int. Ed. , 37 : 2435 – 2451 .
- Ivleva , N. P. , Messerer , A. , Yang , X. , Niessner , R. and Pöschl , U. 2007 . Raman Microspectroscopic Analysis of Changes in the Chemical Structure and Reactivity of Soot in a Diesel Exhaust Aftertreatment Model System . Environ. Sci. Technol. , 41 : 3702 – 3707 .
- Ivleva , N. P. , Niessner , R. and Panne , U. 2005 . Characterization and Discrimination of Pollen by Raman Microscopy . Anal. Bioanal. Chem. , 381 : 261 – 267 .
- Jawhari , T. , Roid , A. and Casado , J. 1995 . Raman Spectroscopic Characterization of Some Commercially Available Carbon Black Materials . Carbon , 33 : 1561 – 1565 .
- Kanakidou , M. , Seinfeld , J. H. , Pandis , S. N. , Barnes , I. , Dentener , F. J. , Facchini , M. C. , Van Dingenen , R. , Ervens , B. , Nenes , A. , Nielsen , C. J. , Swietlicki , E. , Putaud , J. P. , Balkanski , Y. , Fuzzi , S. , Horth , J. , Moortgat , G. K. , Winterhalter , R. , Myhre , C. E. L. , Tsigaridis , K. , Vignati , E. , Stephanou , E. G. and Wilson , J. 2005 . Organic Aerosol and Global Climate Modelling: A Review . Atmos. Chem. Phys. , 5 : 1053 – 1123 .
- Krüger , B. J. , Grassian , V. H. , Iedema , M. J. , Cowin , J. P. and Laskin , A. 2003 . Probing Heterogeneous Chemistry of Individual Atmospheric Particles Using Scanning Electron Microscopy and Energy-Dispersive X-ray Analysis . Anal. Chem. , 75 : 5170 – 5179 .
- Laskin , A. , Iedema , M. J. and Cowin , J. P. 2002 . Quantitative Time-Resolved Monitoring of Nitrate Formation in Sea Salt Particles Using a CCSEM/EDX Single Particle Analysis . Environ. Sci. Technol. , 36 : 4948 – 4955 .
- Lohmann , U. and Feichter , J. 2005 . Global Indirect Aerosol Effects: A Review . Atmos. Chem. Phys. , 5 : 715 – 737 .
- McCreery , R. L. 2000 . Raman Spectroscopy for Chemical Analysis , 15 – 35 . New York : John Wiley & Sons .
- McFiggans , G. , Artaxo , P. , Baltensperger , U. , Coe , H. , Facchini , M. C. , Feingold , G. , Fuzzi , S. , Gysel , M. , Laaksonen , A. , Lohmann , U. , Mentel , T. F. , Murphy , D. M. , O'Dowd , C. D. , Snider , J. R. and Weingartner , E. 2006 . The Effect of Physical and Chemical Aerosol Properties on Warm Cloud Droplet Activation . Atmos. Chem. Phys. , 6 : 2593 – 2649 .
- Mertes , S. , Dippel , B. and Schwarzenbock , A. 2004 . Quantification of Graphitic Carbon in Atmospheric Aerosol Particles by Raman Spectroscopy and First Application for the Determination of Mass Absorption Efficiencies . J. Aerosol Sci. , 35 : 347 – 361 .
- Messerer , A. , Niessner , R. and Pöschl , U. 2006 . Comprehensive Kinetic Characterization of the Oxidation and Gasification of Model and Real Diesel Soot by Nitrogen Oxides and Oxygen Under Engine Exhaust Conditions: Measurement, Langmuir-Hinshelwood, and Arrhenius Parameters . Carbon , 44 : 307 – 324 .
- Niessner , R. 1991 . Chemical Characterizations of Aerosols: On Line and In Situ . Angew. Chem., Int. Ed. , 103 : 542 – 552 .
- Nyguist , R. A. , Putzig , C. L. and Leugers , M. A. 1997 . The Handbook of Infrared and Raman spectra of Inorganic Compounds and Organic Salts, Four Volume Set , Vol. 1 , 77 – 224 . San Diego : Academic Press .
- Oberdorster , G. , Oberdorster , E. and Oberdorster , J. 2005 . Nanotoxicology: An Emerging Discipline Evolving from Studies of Ultrafine Particles . Environ. Health Perspect. , 113 : 823 – 839 .
- Pöschl , U. , Letzel , T. , Schauer , C. and Niessner , R. 2001 . Interaction of Ozone and Water Vapor with Spark Discharge Soot Aerosol Particles Coated with Benzo[a]pyrene: O3 and H2O Adsorption, Benzo[a]pyrene Degradation and Atmospheric Implications . J. Phys. Chem. A , 105 : 4029 – 4041 .
- Pöschl , U. 2003 . Aerosol Particle Analysis: Challenges and Progress . Anal. Bioanal. Chem. , 375 : 30 – 32 .
- Pöschl , U. 2005 . Atmospheric Aerosols: Composition, Transformation, Climate and Health Effects . Angew. Chem., Int. Ed. , 44 : 7520 – 7540 .
- Potgieter-Vermaak , S. S. and Van Grieken , R. 2006 . Preliminary Evaluation of Micro-Raman Spectrometry for the Characterization of Individual Aerosol Particles . Appl. Spectrosc. , 60 : 39 – 47 .
- Rosen , N. and Novakov , T. 1977 . Raman Scattering and Characterisation of Atmospheric Aerosol Particles . Nature , 266 : 708 – 710 .
- Sadezky , A. , Muckenhuber , H. , Grothe , H. , Niessner , R. and Pöschl , U. 2005 . Raman Spectra of Soot and Related Carbonaceous Materials: Spectral Analysis and Structural Information . Carbon , 43 : 1731 – 1742 .
- Schauer , C. , Niessner , R. and Pöschl , U. 2003 . Polycyclic Aromatic Hydrocarbons in Urban Air Particulate Matter: Decadal and Seasonal Trends, Chemical Degradation, and Sampling Artifacts . Environ. Sci. Technol. , 37 : 2861 – 2868 .
- Schauer , C. , Niessner , R. and Pöschl , U. 2004 . Analysis of Nitrated Polycyclic Aromatic Hydrocarbons by Liquid Chromatography with Fluorescence and Mass Spectrometry Detection: Air Particulate Matter, Soot, and Reaction Product Studies . Anal. Bioanal. Chem. , 378 : 725 – 736 .
- Schmid , H. , Laskus , L. , Jurgen Abraham , H. , Baltensperger , U. , Lavanchy , V. , Bizjak , M. , Burba , P. , Cachier , H. , Crow , D. , Chow , J. , Gnauk , T. , Even , A. , ten Brink , H. M. , Giesen , K. P. , Hitzenberger , R. , Hueglin , C. , Maenhaut , W. , Pio , C. , Carvalho , A. , Putaud , J. P. , Toom-Sauntry , D. and Puxbaum , H. 2001 . Results of the “Carbon Conference” International Aerosol Carbon Round Robin Test Stage I . Atmos. Environ. , 35 : 2111 – 2121 .
- Seinfeld , J. H. and Pandis , S. N. 1998 . Atmospheric Chemistry and Physics: From Air Pollution to Climate Change , 408 – 763 . New York : Wiley .
- Su , D. S. , Jentoft , R. E. , Müller , J. O. , Rothe , D. , Jacob , E. , Simpson , C. D. , Tomovic , Z. , Müllen , K. , Messerer , A. , Pöschl , U. , Niessner , R. and Schlögl , R. 2004 . Microstructure and Oxidation Behaviour of Euro IV Diesel Engine Soot: A Comparative Study with Synthetic Model Soot Substances . Catal. Today , 90 : 127 – 132 .
- Sze , S.-K. , Siddique , N. , Sloan , J. J. and Escribano , R. 2001 . Raman Spectroscopic Characterization of Carbonaceous Aerosols . Atmos. Environ. , 35 : 561 – 568 .
- Temesi , D. , Molnar , A. , Meszaros , E. , Feczko , T. , Gelencser , A. , Kiss , G. and Krivacsy , Z. 2001 . Size Resolved Chemical Mass Balance of Aerosol Particles Over Rural Hungary . Atmos. Environ. , 35 : 4347 – 4355 .
- Tuinstra , F. and König , J. L. 1970 . Raman Spectrum of Graphite . J. Chem. Phys. , 53 : 1126 – 1130 . VDI (1996). Measurement of Soot (immission)—Chemical Analysis of Elemental Carbon by Extraction and Thermal Desorption of the Organic Carbon, VDI guideline 12 (2465 Part 1)
- Wittmaack , K. 2005 . Combustion Characteristics of Water-Insoluble Elemental and Organic Carbon in Size Selected Ambient Aerosol Particles . Atmos. Chem. Phys. , 5 : 1905 – 1913 .
- Yang , Y.-h. and Wang , T. 1997 . Fourier Transform Raman Spectroscopic Characterization of Humic Substances . Vib. Spectrosc. , 14 : 105 – 112 .
- Zerrath , A. 2005 . Analysis of Cellulose und Glucose in Atmospheric Aerosol Samples and Physical Aerosol-Characterization with an Electric Low Pressure Impactor: Dissertation , Technical University Munich .
- Zhang , X. and McMurry , P. H. 1992 . Evaporative Losses of Fine Particulate Nitrates During Sampling . Atmos. Environ., Part A , 26A : 3305 – 3312 .