Abstract
Iron oxide nanoparticles, to be used in a health effects study, were synthesized in a H2/air diffusion flame and characterized by transmission electron microscopy, X-ray diffraction, surface area measurement, inductively coupled plasma mass spectrometry, and a spectrophotometric speciation method. The nanoparticles exhibited the maghemite (γ -Fe2O3) crystal structure and contained only trivalent iron. There were two size modes in the particles. The large size mode contained crystalline, non-agglomerated particles with a median diameter of approximately 45 nm; the small size mode contained particles that were in the size range of 3–8 nm and were mostly amorphous. Depending on the value taken for the small particle size, the small mode accounted for 73–82% of the particle surface area. The particles in the small size mode were likely formed from the vapor of FeO and Fe.
INTRODUCTION
The inhalation of airborne particles has been linked to adverse health effects by epidemiological studies (CitationAnalitis et al. 2006; CitationDockery et al. 1993; CitationSamet et al. 2000; CitationSamoli et al. 2005). Considerable research effort has been expended in the assessment of the factors that contribute to the health effects of atmospheric aerosols (CitationKaiser 2005). Some studies have shown that smaller particles are more potent than larger ones in causing adverse health effects (CitationHauser et al. 2001; CitationOberdorster et al. 1996; CitationSchwartz and Neas 2000), but it is also suggested that data on the health effects of ultrafine (< 100 nm) particles are still inadequate to draw an unequivocal conclusion (CitationHarrison and Yin 2000). It is recognized that there is a major gap in knowledge about the relationship between toxicity and particle physico-chemical properties (CitationSchlesinger et al. 2006). This highlights the importance of particle characterization when conducting health effects studies (CitationSingh et al. 2004).
A few epidemiological studies have investigated the correlation between the components (or the sources) of particulate matter and health effects in terms of clinic diagnosis or mortality rate (CitationLanki et al. 2006; CitationMar et al. 2000). These studies provide statistical data of the correlation between health effects (e.g., mortality or cardiovascular abnormality) and the different fractions of the particulate matter, such as elemental carbon, sulfate, and the various metals. Besides epidemiological studies, intensive efforts have been invested in toxicological research (CitationOberdorster 2001). Several studies have used synthesized particles to investigate the mechanism of toxicity of metals (CitationCheng 2004; CitationGojova et al. 2007; CitationZhou et al. 2003a). One of the benefits of using synthesized particles is that thorough characterization of the particles can be achieved at reasonable cost, due to the possibility to control the chemical composition and particle size. Although it is difficult for synthesized particles to provide the exact mixture of components found in atmospheric particles, they do provide an opportunity for researchers to examine the correlation between a biological response and a specific type of particles.
Epidemiological and toxicological studies have consistently shown that metals can be involved in the development of pulmonary and cardiovascular diseases (CitationSchwarze et al. 2006). As one of the most abundant transition metals in atmospheric particulate matter (CitationHughes et al. 1998), iron is believed to cause oxidative stress in biological systems (CitationLund and Aust 1991). Previous toxicity studies (CitationZhou et al. 2003a, Citation2003b) have used flame synthesized iron-bearing particles. The synthesis and characterization of these particles were well-characterized (CitationYang et al. 2001); such documentation is obviously necessary for finding the correlation between toxicity and particle properties.
We report in this article details about the flame synthesis and characterization of iron oxide nanoparticles that were used in a health effects study (CitationGojova et al. 2007). That study was designed to assess the health effects of different metal oxides in cultured vascular endothelial cells. A H2 flame was used to prevent carbon contamination of the nanoparticles that is likely with a hydrocarbon flame (CitationZhou et al. 2003a). Although the formation of iron oxide nanoparticles in flames has been previously investigated in a number of studies (CitationJanzen et al. 2002; CitationJossen et al. 2005; CitationMcMillin et al. 1996; CitationRumminger and Linteris 2000; CitationYang et al. 2001), this article reports a significant feature of the iron oxide nanoparticles synthesized in this study: ultrafine nanoparticles of iron oxide (3–8 nm in diameter). Such small iron oxide particles have been briefly discussed in the recently published health effects study (CitationGojova et al. 2007), but no detailed characterization and discussion of the small particles' origin were given in that work. These small particles may account for a large fraction of the particle surface area, which is an important factor in determining health effects. In addition, the hypothesized formation mechanism of these small iron oxide particles may be found in other combustion processes and hence is relevant to the understanding of their particulate emissions.
EXPERIMENTAL
The Fe2O3 nanoparticles were synthesized in a H2/air diffusion flame. A schematic of the experimental apparatus is shown in . A tubular burner made of 3/8-inch stainless steel tubing (inner diameter 7.5 mm) was situated in the center of a vertical wind tunnel that has a cross section of 300 mm by 300 mm. HEPA filtered, particle-free air flowed through the wind tunnel to form a uniform laminar flow in the working section. The wind tunnel was operated at atmospheric pressure and room temperature, with an air speed of 1.9 ± 0.1 m s−1. The vapor of iron pentacarbonyl (Alfa Aesar, chemical formula Fe(CO)5), which decomposes at 200°C, was doped in the H2 fuel gas so as to provide the precursor for iron. To achieve the desired Fe(CO)5 concentration, the fuel gas flow was split into two streams, only one of them used as the Fe(CO)5 carrier gas. The pure H2 stream was well-mixed with the Fe(CO)5-laden stream before entering the burner. The Fe(CO)5 carrier H2 gas flowed through the headspace of an airtight Fe(CO)5 container, which was maintained in an ice-water bath to insure a steady Fe(CO)5 concentration. The concentration of Fe(CO)5 in the fuel gas was estimated from the mixing ratio of the Fe-laden H2 and the pure H2, assuming that the carrier gas was saturated with Fe(CO)5 vapor. A stable, self-sustaining laminar diffusion flame was established on the tubular burner. The iron oxide nanoparticles for the health effects study were synthesized using a total H2 flow rate of 0.2 liter min− 1, of which 0.1 liter min− 1 was the Fe(CO)5 carrier gas. Other H2 fuel gas flow rates and Fe(CO)5 concentrations were also used for studying the formation mechanism of the iron oxide nanoparticles. A 4-channel MKS 647C (MKS Instruments Inc., MA) mass flow controller was used to control the H2 gas flows.
The flame synthesized iron oxide nanoparticles were collected and analyzed by transmission electron microscopy (TEM), X-ray diffraction (XRD), inductively coupled plasma mass spectrometry (ICP-MS), the Brunauer-Emmett-Teller (BET) surface area measurement method, and a spectrophotometry method. The post-flame iron oxide aerosol was drawn into a sampling tube positioned above the burner on-axis, and either collected on a Cole-Parmer TEFLONTM 47 mm membrane filters with a nominal pore size of 200 nm, or analyzed on-line with a TSI Scanning Mobility Particle Sizer (SMPS), which consisted of a 3071A Differential Mobility Analyzer (DMA) and a 3025A Condensation Particles Counter (CPC). An in-house vacuum system provided the suction for the sampling apparatus and the SMPS. Thermophoretic sampling was also used to extract particle samples from the flame interior in the same manner as used in a previous study (CitationGuo and Kennedy 2004). Aerosol particles in the flame were deposited onto a TEM grid mounted on the sampling probe, when the probe was quickly inserted into the flame and retracted. The particles deposited on the TEM grid were then analyzed by TEM.
Particles collected on filters were analyzed by TEM, XRD, ICP-MS, and BET. For the TEM and XRD analyses, the nanoparticles were first carefully scraped off the membrane filter and dispersed in ethanol with the assistance of ultrasound sonication. To prepare a XRD sample, the ethanol suspension containing iron oxide nanoparticles was deposited drop-wise on a single crystal Si substrate and dried to obtain a thin layer of particles. Drops of the ethanol suspension were put on copper TEM grids with carbon film support. After evaporation of the solvent, the particles were deposited on the grids and TEM samples were thus obtained. TEM imaging was carried out on a Philips CM-12 transmission electron microscope (FEI, Hillsboro, OR) operated at 100 kV. High-resolution transmission electron microscopy (HRTEM) and selected area electron diffraction (SAED) were conducted on a JEOL2010 microscope (JEOL Ltd., Tokyo, Japan) operated at 200 kV. The XRD analysis was carried out in a Scintag PAD V X-ray diffractometer (Thermo Optek, Franklin, MA) with Cu Kα radiation operated at 45 kV and 40 mA. The XRD patterns were analyzed using the MDI JADE 6.0 program. For the BET analysis, about 30 mg of particle sample was gently pressed into a pellet and the specific surface area of the sample was measured in a Gemini 2360 instrument (Micromeritics, Nocross, GA). The ICP-MS analysis was done on an Agilent Technologies 7500c inductively coupled plasma mass spectrometer (Agilent Technologies, Santa Clara, CA). Germanium (Ge) solution was added to all solutions as an internal standard in order to correct for instrumental drift during acquisition; the Ge concentration in the spiked solutions was adjusted to 50 ppb. Signals for the isotopes 57Fe (or 56Fe) and 72Ge were used in determining the concentration of Fe and other elements. In 56Fe measurements, hydrogen gas was introduced into the octopole cell as a reaction gas (3.0 ml/min) to minimize isobaric interference from the oxide of argon carrier gas (40Ar16O+). The flow rate of argon gas for plasma was 1.08 liter min−1. Samples were introduced at a rate of ∼ 2 ml min−1 and clean 3% nitric acid was used for washing between samples. The acquisition time was 0.3 sec per isotope, repeated 10 times. The precision of the analysis was better than ± 3.8%.
The iron oxide particles collected on filters were also analyzed for iron oxidation state by a spectrophotometric method following acid digestion. The particles were carefully scraped off the filter, weighed and digested in sulfuric acid. The concentration of Fe2 + and Fe3 + ions in the digestion solution was measured using the spectrophotometer (CitationShirakashi 1993; CitationWeinberg et al. 2002). The detailed procedure of this method is provided in the Appendix. Iron(II) sulfate heptahydrate (chemical formula FeSO4 7H2O, Alfa Aesar, > 99%) was used to prepare the standard solution for calibration. Fe(II), Fe(III) and Fe(II, III) oxides (chemical formula FeO, Fe2O3, and Fe3O4, Alfa Aesar, > 99%) were used for validation of this iron speciation measurement procedure.
RESULTS
A TEM image of flame-synthesized iron oxide nanoparticles is shown in . These particles were produced at a total H2 flow rate of 0.2 SLM (liter per minute at standard temperature and pressure), of which 0.01 SLM was the Fe(CO)5 carrier gas. The estimated molar concentration of Fe(CO)5 vapor in the fuel gas was approximately 500 ppm. Typically 5 mg of sample was collected on the filter in 1 hour. This synthesis condition was selected because it produced a median particle diameter close to 50 nm, which was the target particle size for the health effects study. The particle size distribution of the iron oxide particles generated in this synthesis condition was measured by SMPS and is shown in . The TEM results indicated that the iron oxide particles contained two size modes. Particles in the larger size mode were non-agglomerated and appeared to have a log-normal size distribution, as indicated by TEM survey. The count median diameter of the log-normal size distribution was approximately 45 nm, with a geometric standard deviation of approximately 1.2. The high-resolution TEM image of a Fe2O3 particle of the large size mode and many small particles is shown in . Particles in the small size mode were approximately 3–8 nm, some of which appeared to have formed aggregates. The bimodal size distribution existed at higher Fe(CO)5 molar concentrations as well. The median particle diameter of the larger size mode increased with higher Fe(CO)5, but the primary diameter of the small size mode did not change significantly as the Fe(CO)5 concentration increased. At Fe(CO)5 concentrations lower than 20 ppm, only particles of the small size mode were formed.
FIG. 2 TEM image of flame-synthesized iron oxide nanoparticles. Two distinct size modes are present. The large size mode is composed of crystalline particles with a median diameter of approximately 45 nm. The small size mode contained very small particles (3–8 nm) that were mostly amorphous. Arrows point to particles in the small size mode. 88 × 66 mm (400 × 400 DPI).
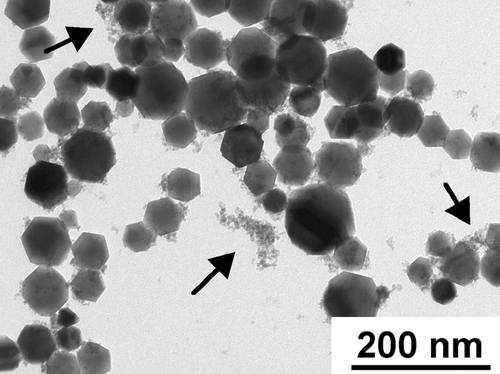
FIG. 3 Particle size distribution measured by Scanning Mobility Particle Sizer. 88 × 84 mm (300 × 300 DPI).
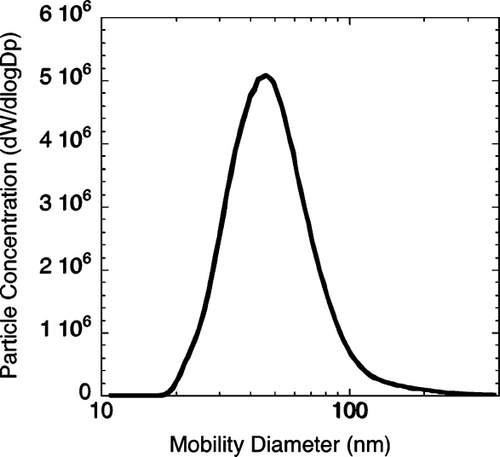
FIG. 4 High-resolution TEM image of particles of the small size mode and a large particle. 177 × 177 mm (400 × 400 DPI).
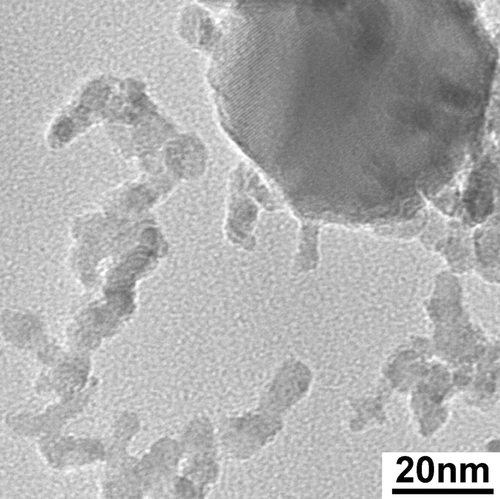
X-ray diffraction and transmission electron microscopy were used to determine the crystal structure of the iron oxide particles collected on filters. shows the XRD pattern for the iron oxide particles that were used in the health effects study. The XRD result showed that the crystalline phase was γ -Fe2O3. The grain size calculated from the XRD data was within about 10% of the median particle diameter of the large size mode determined by SMPS and TEM. Selected area electron diffraction (SAED) results (not shown) indicated that the particles in the large size mode were crystalline and the diffraction patterns were assigned to γ -Fe2O3. The particles in the small size mode did not produce distinct electron diffraction signal. Therefore the valence state of iron in the small size mode could not be readily determined based on crystallographic information. The spectrophotometric method was thus used to measure the valence state of the iron in the particles. No Fe2 + was detected by this method after the iron oxide particles were dissolved with sulfuric acid. Tests using commercial FeO, Fe3O4, and Fe2O3 powders showed that this method accurately reported the original Fe2+/Fe3 + ratios in the samples. The method was able to detect Fe2 +/Fe3 + ratios below 1%.
FIG. 5 XRD result of the iron oxide nanoparticles. The pattern is from flame-synthesized particles used for the health effects study (with bimodal size distribution). The vertical lines are the major lines from the JCPDS standard PDF #39-1346. 177 × 88 mm (300 × 300 DPI).
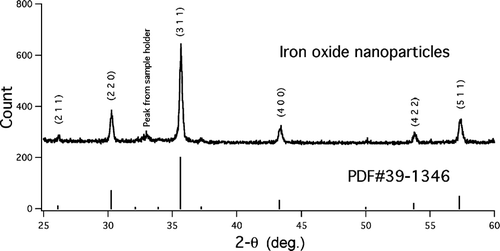
The BET measurement indicated that the iron oxide nanoparticles used in the health effects study had a specific surface area of 81 ± 1 m2 g− 1. The ICP-MS results showed that the iron oxide nanoparticles were of very high purity (> 99.99%, mass ratio of Fe to total metal). The only detectable impurities were trace amounts of Cu, Mn, and Se.
Particles were taken from different reaction stages (different heights above the burner) within the flame by thermophoretic sampling and viewed by TEM. This technique was used to study the history of particle evolution in flames at various Fe(CO)5 concentrations. The trend of particle evolution in the flame was found to be similar for the different flame conditions. shows the results for a flame with H2 flow rate of 1 SLM and an estimated Fe(CO)5 molar concentration of 70 ppm. In the early stages of the flame (5 mm above burner), where the temperature is low and the atmosphere is reducing (CitationGuo et al. 2006; CitationGuo and Kennedy 2004), particles with a mean diameter of approximately 20 nm were formed. No particles of the small size mode were found until at a stage where the flame temperature reached a maximum (25 mm above burner). The number of particles in the small size mode apparently continued increasing from this stage onward (35 mm above burner), as indicated by the number ratio of the small size mode to the large size mode.
FIG. 6 Photograph of a H2 synthesis flame and TEM micrographs of iron oxide (or iron) particles taken from flame interior. The particles were sampled thermophoretically at 15 mm (A), 25 mm (B) and 35 mm (C) above the burner, respectively. For reference, the highest luminous tip of the flame is about 45 mm above the burner mouth. The micrographs indicate that particles of the small size mode started forming in the maximum temperature region in the flame. 88 × 104 mm (300 × 300 DPI).
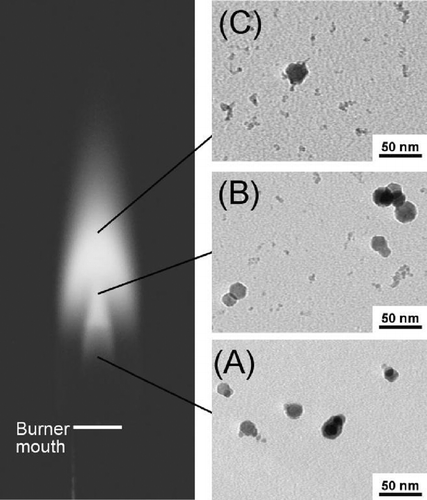
DISCUSSION
In studying the health effects of particles, particle size and specific surface area are of great interest since chemical reactions involving the particles take place on the surface. Because the Fe2O3 particles synthesized for the heath effects study exhibited a bimodal size distribution, it became desirable to identify the contribution of each size mode to the particle mass and surface area. The SMPS measurement failed to reveal the bimodal nature of this size distribution. This is possibly due to two reasons. First, the small particles appeared to have formed aggregates, and/or diffused onto the surface of the large particles. Second and more obviously, the primary particle size of the small size mode was smaller than the lower detection limit of the model of SMPS used in the study. However, the number concentration ratio of two size modes, RS/L, may be calculated using the following equation:


ρ is the density of maghemite, 4.88 g cm−3 (CitationLide 2001);
θ is the specific surface area, large and small modes both included, measured by the BET method, 81 ± 1 m2 g−1;
d
_Large and d
_Small are the diameters of particles with average surface area for the large mode and the small size mode;
Assuming the large size mode has a log-normal distribution, d_Large and d
_Large can be calculated from the count median diameter (CMD) and geometric standard deviation (GSD) (CitationHinds 1999). Based on TEM survey, the CMD and the GSD of the large size mode were approximately 45 nm and 1.2, respectively. The calculated values of d
_Large and d
_Large were both approximately 47 nm. The characteristic diameters d
_Small and d
_Small for the small mode were assumed to have the same value because of the narrow size range. Using several estimated values for d
_Small (d
_Small), Equation (Equation1) was solved to yield a value of for RS/L, which was in turn used to calculate the mass and surface area fractions of the small particles. The results based on different d
_Small (d
_Small) values are shown in . Depending on the value taken for the small particle size, the small mode might account for 73–82% of the total particle surface area of the iron oxide nanoparticles generated in this study. It should be noted that the number ratio of “small” and “large” particles visible in the TEM image cannot be used to verify the value of RS/L, mainly because of overlapping of particles in the TEM sample.
TABLE 1 Contributions from the small size mode at various values of dm_Small or (ds_Small)
The oxidation state of iron is an important factor that influences the health effects of iron-containing aerosol particles. One hypothesized mechanism of iron toxicity is through the role of Fe(II) in the Fenton reaction to produce hydroxyl radicals:
The hydroxyl radicals are highly reactive and can cause damage to biomolecules. An animal study has attributed the adverse health effects to the Fe(II) fraction in a soot/Fe aerosol that contained mostly γ -Fe2O3 (CitationZhou et al. 2003a). Since the particles in the small size mode in this study were largely amorphous and did not produce distinct X-ray or electron diffraction signal, the spectrophotometric method served as a simple and reliable complementary method for determining the oxidation state of iron. The small size mode accounted for 28% of the total particle mass. Thus, within its detection limit, the spectrophotometry result indicated that all the iron in the flame-synthesized nanoparticles was trivalent iron (Fe3 +).
The TEM results on the particle samples taken by thermophoretic sampling indicated that the small size mode formation occurred in the region of the flame where the temperature reached a maximum. To help explain this observation, the computer program STANJAN (CitationReynolds 1986) was used to calculate the equilibrium species at different combustion stages in the flame. Similar calculations have proved useful in studying chromium oxide aerosol formation in diffusion flames (CitationGuo and Kennedy 2004). The scheme and results of the equilibrium calculation are shown in . As the iron species of interest are almost all in the aerosol form, and the aerosol particles are confined to the vicinity of the flame axis due to relatively slow particle diffusion, the following discussion is applied to the conditions along the flame centerline. Measured flame temperatures at representative points (CitationGuo and Kennedy 2004) on the flame axis were used in STANJAN to calculate the fuel/oxygen mixing ratio assuming adiabatic conditions. The fuel/oxygen mixing ratio and temperature were then used to calculate the equilibrium concentration of the product species at these points. The initial concentration of Fe(CO)5 in the fuel gas was assumed to be 100 ppm. The major iron species included in the equilibrium calculations were Fe, FeO, Fe2O3, and Fe3O4. The results show that there is a peak in the equilibrium concentration of Fe vapor and FeO vapor near the maximum temperature region, accompanied by a drastic decrease in liquid FeO and an increase of Fe2O3 and Fe3O4 (Fe3O4/Fe2O3 ratio ∼ 1%).
FIG. 7 Scheme of chemical equilibrium calculation and results for representative points on flame axis. The flame temperature at each point was measured. The mixture fraction was back calculated from the measured flame temperature. The molar concentration of Fe(CO)5 in the fuel gas used in the calculation was 100 ppm. Letters in the parentheses indicate the phase of the species, g being gas-phase, and c condensates. Fe2O3 and Fe3O4 are always condensates. 88 × 158 mm (300 × 300 DPI).
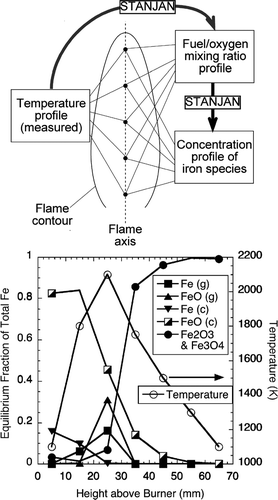
A formation mechanism for the small mode iron oxide particles may be proposed based on the experimental results, the chemical equilibrium calculation, and literature data. The mechanism is schematically shown in . In the lower part of the flame (on the flame centerline) and the reducing atmosphere on the fuel side, Fe(CO)5 pyrolyzes to produce elemental Fe (CitationZhao et al. 1994). As the iron moves along the flame axis, the oxygen concentration increases due to diffusion from the oxidizer side, and so does the temperature. Iron is then oxidized into FeO. Next, in the maximum temperature region liquid FeO converts to vapor-phase FeO. After this region, the flame temperature decreases due to mixing of the hot flame gases with the cool ambient air. The FeO and Fe vapors nucleate to form very small particles, and convert to Fe2O3 due to the super-stoichiometric oxygen concentration. In the mean time, the remaining liquid FeO particles convert to Fe2O3 particles due to the increased oxygen partial pressure. Experimental results by other researchers appear to support this qualitative description of iron oxide aerosol formation in the flame, particularly the role of FeO or Fe vapor plays. CitationMcMillin et al. (1996) used laser induced fluorescence and light scattering to measure the gas-phase FeO concentration and particle formation in a premixed flame seeded with Fe(CO)5. They found a rapid rise of FeO concentration immediately downstream of the flame front, where the temperature was high. They also noted particle formation with a concurrent decrease in FeO vapor concentration.
The formation of small iron oxide particles from FeO and Fe vapor is of significance to the understanding of the nature of real-world combustion emissions. Diesel exhaust particulate matter is known to contain significant concentrations of Fe and other metals (CitationHuggins et al. 2000), but so far little has been reported about the physio-chemical state of the metals in the carbonaceous matrix. Current literature data suggest that FeO vapor exists in hydrocarbon flames at significant concentrations (CitationMcMillin et al. 1996; CitationZachariah et al. 1996), hence it is reasonable to believe that the formation mechanism of small (3–8 nm) iron oxide particles that we have hypothesized in this study may also play a role in hydrocarbon flames that contain iron.
CONCLUSION
A gas-phase flame process was used for the synthesis of iron oxide nanoparticles for a particle health effects study. The flame-synthesized iron oxide particles were characterized for their size distribution, specific surface area, metal impurity, crystal structure, and the oxidation state of iron, using SMPS, XRD, TEM, HRTEM, BET, ICP-MS, and an acid-digestion-spectrophotometric method. The iron oxide particles had a γ -Fe2O3 crystal structure and contained only trivalent Fe, with only trace amounts of metal impurity. The iron oxide particles had two distinct size modes: the small size mode was composed of 3–8 nm primary particles. Depending on the value taken for the small particle size, the small size mode might account for 73–82% of the total particle surface area. TEM of thermophoretic particle samples, chemical equilibrium calculation, and literature data suggested that the iron oxide particles in the small size mode were probably formed from the vapor of FeO and/or Fe. This hypothesized formation mechanism may play a role in hydrocarbon combustion processes in which Fe is present.
Appendix: Procedure for the Acid Digestion and Spectrophotometry Method for Measurement of Fe2 + and Fe3 + Concentrations
1. Spectrophotometer: Cole-Parmer, set at 512 nm, read in the absorbance mode.
2. Chemicals: All chemicals were from Alfa Aesar, with the highest purity.
3. Standard Solution and Calibration Curve:
1. | Prepare a stock Fe solution by accurately weighing to the nearest 0.1 mg approximately 0.05 g of FeSO4· 7H2O and quantitatively transferring to a 1-liter volumetric flask. | ||||
2. | Add 200 ml water and shake to dissolve any remaining solid. | ||||
3. | Add 20 mL of 20% sulfuric acid. | ||||
4. | Dilute to the mark with distilled water and homogenize thoroughly. | ||||
5. | Calculate the concentration of the solution in mg Fe/litter solution. (10 mg/l) | ||||
6. | Prepare a series of standards by pipetting into each of five 100 ml volumetric flasks, 1.00, 5.00, 10.00, 25.00, and 50.00 ml aliquots of the stock Fe(II) solution (a buret can be used for this addition). | ||||
7. | Into a sixth 100 ml volumetric flask pipet 50 ml of distilled water to serve as a blank. | ||||
8. | To all of the solutions add, in sequence, 1 ml of 1% hydroquinone water solution (freshly prepared in amber bottle), 10 ml of 0.3% 1,10-phenanthroline solution (in water with 10% ethanol, freshly prepared in amber bottle), and 20 ml of 5% potassium acetate buffer. | ||||
9. | Dilute to the mark, mix thoroughly, and allow to stand for 10 minutes. | ||||
10. | Read absorbance and plot calibration curve. |
4. Digestion of Iron Oxide Particles:
1. | Weigh flame-synthesized iron oxide particles or commercial powders (mass range approximately 2–10 mg) and put in a beaker, add 20 mL 20% sulfuric acid, add DI water to 100 mL, heat at 80°C for 1 hour. Then transfer solution into a 1-liter volumetric flask. | ||||
2. | Dilute with DI water to mark. | ||||
3. | Pipet 50.00 ml aliquots of the sample into two 100 mL volumetric flask A and B. | ||||
4. | To flask A add 1 ml of 1% hydroquinone water solution (freshly prepared in amber bottle), shake well and allow to sit for 1 minute. Then add to both flasks 10 ml 0.3% 1,10-phenanthroline solution (in water with 10% ethanol, freshly prepared in amber bottle). | ||||
5. | Dilute to the mark, mix thoroughly, and allow to stand for 10 minutes. | ||||
6. | Read absorbance for solutions in both flasks A and B. | ||||
7. | Compare absorbance with calibration curve to obtain concentration of Fe(total) and Fe(II). Absorbance of solution A yields concentration of Fe(total), while B yields Fe(II). |
Acknowledgments
Recieved 14 March 2007; accepted 31 July 2007. The authors thank Dr. Alexandra Navrotsky, Mr. John M. Neil, and Dr. Sergey Ushakov of UC Davis for the XRD and BET analyses, Drs. Valerie Leppert and Jacek Jasinski of UC Merced for part of the TEM analysis, and Dr. Ryoji Shiraki of UC Davis for the ICP-MS analysis. The Microscopy and Imaging Center of Texas A&M University is acknowledged for the use of the JEOL2010 microscope. The authors thank two anonymous reviewers for useful suggestions. The authors acknowledge the support of the Superfund Basic Research Program with Grant 5P42ES04699 from the National Institute of Environmental Health Sciences, NIH. Financial support was also received from the Texas Engineering Experiment Station and Texas A&M University.
REFERENCES
- Analitis , A. , Katsouyanni , K. , Dimakopoulou , K. , Samoli , E. , Nikoloulopoulos , A. K. , Petasakis , Y. , Touloumi , G. , Schwartz , J. , Anderson , H. R. , Cambra , K. , Forastiere , F. , Zmirou , D. , Vonk , J. M. , Clancy , L. , Kriz , B. , Bobvos , J. and Pekkanen , J. 2006 . Short-Term Effects of Ambient Particles on Cardiovascular and Respiratory Mortality . Epidemiology , 17 : 230 – 233 .
- Cheng , M. D. 2004 . Effects of Nanophase Materials (< = 20 nm) on Biological Responses . Journal of Environmental Science and Health Part a-Toxic/Hazardous Substances & Environmental Engineering , 39 : 2691 – 2705 .
- Dockery , D. W. , Pope , C. A. , Xu , X. P. , Spengler , J. D. , Ware , J. H. , Fay , M. E. , Ferris , B. G. and Speizer , F. E. 1993 . An Association between Air-Pollution and Mortality in 6 United-States Cities . New England Journal of Medicine , 329 : 1753 – 1759 .
- Gojova , A. , Guo , B. , Kota , R. S. , Rutledge , J. C. , Kennedy , I. M. and Barakat , A. I. 2007 . Induction of Inflammation in Vascular Endothelial Cells by Metal Oxide Nanoparticles: Effect of Particle Composition . Environmental Health Perspectives , 115 : 403 – 409 .
- Guo , B. , Harvey , A. , Risbud , S. H. and Kennedy , I. M. 2006 . The Formation of Cubic and Monoclinic Y2o3 Nanoparticles in a Gas-Phase Flame Process . Philosophical Magazine Letters , 86 : 457 – 467 .
- Guo , B. and Kennedy , I. M. 2004 . The Speciation and Morphology of Chromium Oxide Nanoparticles in a Diffusion Flame . Aerosol Science and Technology , 38 : 424 – 436 .
- Harrison , R. M. and Yin , J. X. 2000 . Particulate Matter in the Atmosphere: Which Particle Properties Are Important for Its Effects on Health? . Science of the Total Environment , 249 : 85 – 101 .
- Hauser , R. , Godleski , J. J. , Hatch , V. and Christiani , D. C. 2001 . Ultrafine Particles in Human Lung Macrophages . Archives of Environmental Health , 56 : 150 – 156 .
- Hinds , W. C. 1999 . Aerosol Technology: Properties, Behavior, and Measuremen of Airborne Particles , New York : Wiley .
- Huggins , F. E. , Huffman , G. P. and Robertson , J. D. 2000 . Speciation of Elements in Nist Particulate Matter Srms 1648 and 1650 . Journal Of Hazardous Materials , 74 : 1 – 23 .
- Hughes , L. S. , Cass , G. R. , Gone , J. , Ames , M. and Olmez , I. 1998 . Physical and Chemical Characterization of Atmospheric Ultrafine Particles in the Los Angeles Area . Environmental Science & Technology , 32 : 1153 – 1161 .
- Janzen , C. , Kleinwechter , H. , Knipping , J. , Wiggers , H. and Roth , P. 2002 . Size Analysis in Low-Pressure Nanoparticle Reactors: Comparison of Particle Mass Spectrometry with In Situ Probing Transmission Electron Microscopy . Journal Of Aerosol Science , 33 : 833 – 841 .
- Jossen , R. , Pratsinis , S. E. , Stark , W. J. and Madler , L. 2005 . Criteria for Flame-Spray Synthesis of Hollow, Shell-Like, or Inhomogeneous Oxides . J. Am. Ceram. Soc , 88 : 1388 – 1393 .
- Kaiser , J. 2005 . Mounting Evidence Indicts Fine-Particle Pollution . Science , 307 : 1858 – 1861 .
- Lanki , T. , de Hartog , J. J. , Heinrich , J. , Hoek , G. , Janssen , N. A. H. , Peters , A. , Stolzel , M. , Timonen , K. L. , Vallius , M. , Vanninen , E. and Pekkanen , J. 2006 . Can We Identify Sources of Fine Particles Responsible for Exercise-Induced Ischemia on Days with Elevated Air Pollution? The Ultra Study . Environmental Health Perspectives , 114 : 655 – 660 .
- Lide , D. R. 2001 . CRC Handbook of Chemistry and Physics , Boca Raton : CRC Press .
- Lund , L. G. and Aust , A. E. 1991 . Iron-Catalyzed Reactions May Be Responsible for the Biochemical and Biological Effects of Asbestos . Biofactors , 3 : 83 – 89 .
- Mar , T. F. , Norris , G. A. , Koenig , J. Q. and Larson , T. V. 2000 . Associations between Air Pollution and Mortality in Phoenix, 1995–1997 . Environmental Health Perspectives , 108 : 347 – 353 .
- McMillin , B. K. , Biswas , P. and Zachariah , M. R. 1996 . In Situ Characterization of Vapor Phase Growth of Iron Oxide-Silica Nanocomposites. 1. 2-D Planar Laser-Induced Fluorescence and Mie Imaging . Journal of Materials Research , 11 : 1552 – 1561 .
- Oberdorster , G. 2001 . Pulmonary Effects of Inhaled Ultrafine Particles . International Archives of Occupational and Environmental Health , 74 : 1 – 8 .
- Oberdorster , G. , Finkelstein , J. , Ferin , J. , Godleski , J. , Chang , L. Y. , Gelein , R. , Johnston , C. and Crapo , J. D. 1996 . Ultrafine Particles as a Potential Environmental Health Hazard—Studies with Model Particles . Chest , 109 : S68 – S69 .
- Reynolds , W. C. 1986 . “ The Element Potential Method for Chemical Equilibrium Analysis: Implementation in the Interactive Program Stanjan ” . In TStanford University Report , 270 HO : ME .
- Rumminger , M. D. and Linteris , G. T. 2000 . The Role of Particles in the Inhibition of Premixed Flames by Iron Pentacarbonyl . Combustion and Flame , 123 : 82 – 94 .
- Samet , J. M. , Dominici , F. , Curriero , F. C. , Coursac , I. and Zeger , S. L. 2000 . Fine Particulate Air Pollution and Mortality in 20 US Cities, 1987–1994 . New England Journal of Medicine , 343 : 1742 – 1749 .
- Samoli , E. , Analitis , A. , Touloumi , G. , Schwartz , J. , Anderson , H. R. , Sunyer , J. , Bisanti , L. , Zmirou , D. , Vonk , J. M. , Pekkanen , J. , Goodman , P. , Paldy , A. , Schindler , C. and Katsouyanni , K. 2005 . Estimating the Exposure-Response Relationships between Particulate Matter and Mortality within the Aphea Multicity Project . Environmental Health Perspectives , 113 : 88 – 95 .
- Schlesinger , R. B. , Kunzli , N. , Hidy , G. M. , Gotschi , T. and Jerrett , M. 2006 . The Health Relevance of Ambient Particulate Matter Characteristics: Coherence of Toxicological and Epidemiological Inferences . Inhalation Toxicology , 18 : 95 – 125 .
- Schwartz , J. and Neas , L. M. 2000 . Fine Particles Are More Strongly Associated Than Coarse Particles with Acute Respiratory Health Effects in Schoolchildren . Epidemiology , 11 : 6 – 10 .
- Schwarze , P. E. , Ovrevik , J. , Lag , M. , Refsnes , M. , Nafstad , P. , Hetland , R. B. and Dybing , E. 2006 . Particulate Matter Properties and Health Effects: Consistency of Epidemiological and Toxicological Studies . Human & Experimental Toxicology , 25 : 559 – 579 .
- Shirakashi , T. 1993 . Reduction Reaction of Fe3+ Ion by Activated Carbon . Nippon Kagaku Kaishi , : 924 – 930 .
- Singh , P. , DeMarini , D. M. , Dick , C. A. J. , Tabor , D. G. , Ryan , J. V. , Linak , W. P. , Kobayashi , T. and Gilmour , M. I. 2004 . Sample Characterization of Automobile and Forklift Diesel Exhaust Particles and Comparative Pulmonary Toxicity in Mice . Environmental Health Perspectives , 112 : 820 – 825 .
- Weinberg , M. C. , Uhimann , D. R. and Smith , G. L. 2002 . Influence of Radiation and Multivalent Catxon Additions on Phase Separation and Crystallization of Glass DOE FG07-970ER45670
- Yang , G. S. , Teague , S. , Pinkerton , K. and Kennedy , I. M. 2001 . Synthesis of an Ultrafine Iron and Soot Aerosol for the Evaluation of Particle Toxicity . Aerosol Science and Technology , 35 : 759 – 766 .
- Zachariah , M. R. , Shull , R. D. , McMillin , B. K. and Biswas , P. 1996 . In Situ Characterization and Modeling of the Vapor-Phase Formation of a Magnetic Nanocomposite . Nanotechnology , 622 : 42 – 63 .
- Zhao , X. Q. , Zheng , F. , Liang , Y. , Hu , Z. Q. and Xu , Y. B. 1994 . Preparation and Characterization of Single-Phase Beta-Fe-Nanopowder from Cw Co2-Laser Induced Pyrolysis of Iron Pentacarbonyl . Materials Letters , 21 : 285 – 288 .
- Zhou , Y. M. , Zhong , C. Y. , Kennedy , I. M. , Leppert , V. J. and Pinkerton , K. E. 2003a . Oxidative Stress and Nf Kappa B Activation in the Lungs of Rats: A Synergistic Interaction between Soot and Iron Particles . Toxicology & Applied Pharmacology , 190 : 157 – 169 .
- Zhou , Y. M. , Zhong , C. Y. , Kennedy , I. M. and Pinkerton , K. E. 2003b . Pulmonary Responses of Acute Exposure to Ultrafine Iron Particles in Healthy Adult Rats . Environmental Toxicology , 18 : 227 – 235 .