Abstract
Aerosol sampling is used to evaluate the health hazards associated with particles deposited in the human breathing system. Impactors, which are extensively employed as aerosol samplers, have low collection efficiency because of particle bounce. The impaction plate is typically coated with oil or grease to prevent particle bounce. However, such coating materials cannot sustain long-term heavy particle loading.
In this study, the impaction plate was recessed, forming a cavity filled with Trypticase Soy Agar (TSA) to reduce particle bounce and re-entrainment. An ultrasonic atomizing nozzle was employed to generate challenge aerosols. An Aerodynamic Particle Sizer (APS) was utilized to measure the number concentrations and the size distributions upstream and downstream of the size-selective devices. A multi-hole impactor and Personal Environmental Monitor PM 2.5 (PEM–PM 2.5 ) were used to evaluate particle bounce and heavy particle loading. Liquid type-Dioctyl phthalate (DOP), soluble solid type-potassium sodium tartrate tetrahydrate (PST) and insoluble solid type-polymethyl methacrylate (PMMA) were investigated, as were different impaction surfaces/surface combinations. The multi-hole impactor coated with silicone oil was compared with a TSA-filled plate. Laboratory results demonstrate that the solid PST particles bounced off the TSA-filled plate less than off the silicone-coated aluminum plate. This study also used a 700-μm-thick layer of silicone oil to prevent TSA dehydration. The experimental results revealed that the silicone-TSA double layer minimized PST particle bounce during the two-hour heavy sampling (mass concentration was around 7.22 mg/m 3 ). Moreover, the PEM-PM 2.5 impactor yielded consistent results when the silicone-TSA double layer method was used. These results are useful for designing bounce-free impaction substrates during heavy load sampling.
INTRODUCTION
Impactors have been commonly used to measure the mass concentration and size distribution of ambient particles. Impactors can also be utilized in respirable sampling when appropriately designed. To determine accurate particle size distributions, particles impacting on the collection substrate must stick to it without bouncing off resulting in subsequent collection on a downstream stage (CitationDzubay et al. 1976; CitationEsmen 1980; CitationMarkowski 1984; CitationLai et al. 2002). Particle bounce-off depends on many factors such as the impaction surface characteristics, coating type and depth, particle types, particle loading on the impaction surface, sampling conditions and impaction substrate design (CitationRao and Whitby 1978a, Citationb; CitationReischl and John 1978; CitationTurner and Hering 1987; CitationNewton et al. 1990; CitationPak et al. 1992; CitationTsai and Cheng 1995; CitationChang et al. 1999; CitationHinds 1999). Therefore, in order to obtain unbiased particle size and mass concentration distributions, particle bounce-off and re-entrainment from the impaction surface must be minimized. This is particularly important for long-term sampling in heavy particle loading conditions that often results in excessive layers of deposited particles. Novel impactor designs, such as the MOUDI, however, may alleviate the problems of excessive particle loading by using rotating impaction stages as described by CitationMarple et al. (1991).
Studies have shown that impaction plates coated with adhesive materials like grease or oil can effectively eliminate particle bounce-off problems. However, greased surfaces are often quickly overloaded with particles. Under such conditions incoming particles impact on collected particles rather than the greased surface resulting in particle bounce-off. This effect is particularly likely to occur during long-term sampling under heavy particle loading concentrations (Reischl and John 1978; Turner and Hering 1987). In some studies low viscosity oils have shown a better performance than grease under certain conditions. This was attributed to the fact that the oils could wick up to the top of particle layer by capillary action and thereby incoming particles would be more likely to be impacting on the coated surface (CitationPak et al. 1992). Low viscosity oil, however, may not be convenient for personal sampling especially when the subject is moving and the sampler may tilt or shake. For example, CitationRobert et al. (2001) studied the sensitivity of the USEPA WINS PM2.5 Separator to different impaction surface substrate preparations in its well-shaped collection region. The WINS' impaction surface substrate consists of a glass fiber filter (37 mm in diameter) saturated with 1 ml of silicone oil. The size-selective performance of WINS was independent of the volume of oil used in the range from 0.75–3 ml. Compared to a conventional flat plate impactor, the WINS impactor was found to be very effective in capturing all large particles in the well-shaped collection region. Unlike conventional flat plate impactors, loading of the WINS substrate shifts the performance curve to smaller cutpoints and large particles are collected before the afterfilter. A metallic well without oil, however, produced a broad separation curve with a 50% cut-off diameter larger than the design value of 2.5 μ m.
CitationLai and Chen (2000) also compared aerosol separation efficiency of inlet heads of a Harvard impactor, Personal Environmental Monitor (PEM) and Anderson dichotomous sampler (model 241). Their study showed that particle bounce-off effect was minimized by a silicone oil coating on the impaction plate. However, impaction cavities and wells can improve impactor performance compared to flat surfaces. Several studies have examined varying configuration and sampling conditions for impactors with collection cavities such as quiescence, no surface coating and additional substrates (CitationScott and Ranz 1976; CitationBiswas and Flagan 1988; CitationTsai and Cheng 1995; CitationChang et al. 1999). Their experimental results indicate that greased substrates eliminate particle bounce. These studies were conducted exclusively to minimize particle bounce and re-entrainment, and, the effect of long-term heavy particle loading was not investigated. The study presented here examines the possibility of achieving high particle collection efficiency for long-term sampling with heavy particle loading through the investigation of various coating techniques. Trypticase Soy Agar (TSA), a commonly used culture medium, was prepared and used to fill the open cavity of an impactor body to minimize solid particle bounce and loading. Laboratory tests identified an optimum configuration, minimizing particle bounce and sustaining heavy particle loading.
EXPERIMENTAL
Two samplers were employed in this experiment—the PEM-PM2.5 (model 200, MSP Co., Minneapolis, MN, USA) and a modified multi-hole impactor. The PEM-PM2.5 sampler is a one-stage impactor with a porous metal impaction plate. The sampling flow rate of the PEM-PM2.5 impactor was 10 L/min, with a 50% cut-off size of 2.5 μ m. The multi-hole impactor was modified and used as a size-selective respirable sampler for sampling particles () (CitationLai et al. 2002). The impactor is comprised of the following three components: (1) a nozzle plate with six inlet holes (diameters of 1.9 mm) as the sampling nozzles; (2) a ring-type impaction medium (thickness of 3.5 mm), including a conventional flat aluminum plate and TSA colloid (Difco, Detroit, MI, USA); and (3) a body with a ring-type open cavity (depth of 3.5 mm). The sampling flow rate of the test multi-hole impactor was set at 3.6 L/min with a calculated 50% cut-off size of approximately 4 μ m. Typically, for a round jet impactor, cut-size (d p50) can be predicted (Marple and Willeke 1976; CitationHinds 1999) based on the impactor's critical dimensions and the properties of the air stream:
Aerosols were drawn into the multi-hole impactor through six inlet nozzles positioned radially at 60 degrees. The particle accelerates through the nozzle and impacts on the collection medium. Two impaction plates were designed—a conventional flat-ring aluminum plate and an elastic TSA-filled plate. When the aluminum plate was assembled with a body part with an open cavity, the multi-hole impactor was designed as a conventional one-stage impactor. However, the soft TSA-filling can be an impaction plate. TSA is a commonly used, soft, semi-solid culture medium designed to support the growth of various bacterial species. In this study, different mass concentrations of TSA were dissolved in hot water; solutions of 1, 3, and 6% TSA were prepared and poured into the open cavity of the impactor body to minimize solid particle bouncing and loading. Unlike a fluid silicone oil filled into an impinger and a conventional hard-flat impaction plate, TSA provides a soft impaction surface. However, to avoid the dehydration of TSA, the TSA-filling cavity was coated with a thin layer of silicone oil (thickness, approximately 700 μ m) to prevent evaporation resulting from air flow. The aerosol flow after impaction was directed into a conical expander (body part) designed to yield a uniform aerosol deposition on the downstream filter for future use. The sampling time for all the sampling procedures was 60 sec. Five replicates of sampling were utilized to measure separation curves. The sampling system, displayed in , was grounded during testing to avoid any interference by electrostatic attraction. Average flow velocity in the test section was 0.1 m/s, measured using an anemometer (model V-01-AN I. Denshi CO., Ltd., Tokyo, Japan.). Hence, the flow field in the test section was under generally calm air conditions. While these two samplers had different sampling flow rates (the multi-hole impactor was 3.6 L/min; the PEM-PM2.5 was 10 L/min), the downstream sampling train between the aerodynamic particle sizer (APS, model 3321, TSI, Inc., Minneapolis, MN, USA) and samplers had an alternate suction apparatus to draw or supply auxiliary air for the samplers. The reference sampling probe was incorporated into the APS attachment and was used to measure upstream challenge aerosols. Aerosol size distribution, number concentrations and wall losses at the sampler, and an upstream measurement location were checked; no statistically significant differences were found. Except for large particle sizes, the size-dependent aspiration efficiency of the reference sampling probe at 3.6 l/min and 10 l/min was similar. All the test results were adjusted for aspiration efficiency. However, the determination of the aspiration efficiency of the reference sampling probe in calm air is a complex procedure not performed in this work.
FIG. 2 Schematic diagram of the experimental set-up (PMMA generation was not simultaneous with PST/DOP generation).
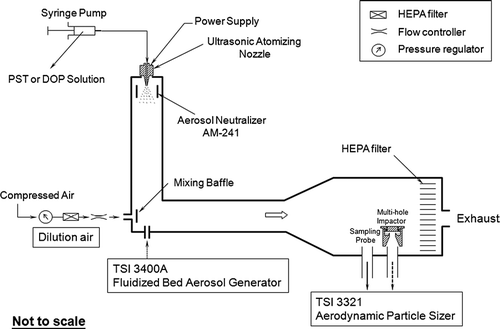
An ultrasonic atomizing nozzle (model 8700-48, Sonotek Inc., Highland, NY, USA) was employed to generate solution droplets with a count median diameter (CMD) of 40–50 μ m and a geometric standard deviation (GSD, σ g ) of around 2. The solution was forced through silicone tubing using a syringe pump. After the solvent had evaporated, the volumetric concentration of the solution being atomized was set to provide a preferred CMD. Furthermore, a calculation based on Hatch-Choate equations provides an estimate of mass mean diameter (MMD) (CitationHinds 1999):
Solid potassium sodium tartrate tetrahydrate (PST) generated by an ultrasonic atomizing nozzle was selected as the challenge aerosol. A liquid Dioctyl phthalate (DOP) was selected to measure impactor penetration characteristics with particles that could not exhibit bounce. To determine the effects of water solubility of the PST, a fluidized bed aerosol generator (model 3400A, TSI, Inc., Minneapolis, MN, USA) was used to generate water-insoluble polymethyl methacrylate (PMMA) powder. Before the aerosols were introduced into the test chamber, the aerosol output from the generator was dried using filtered compressed air and then neutralized to the Boltzmann charge equilibrium by passing the aerosols through an annular 10 mCi Am-241 radioactive source (). The size distribution of challenge aerosols was set to a CMD of 5.86 μ m, with a GSD of about 1.56, to yield a complete separation curve over the size range of interest. A mass concentration of PST of about 7.22 mg/m3 was generated to show a severe particle loading situation. The mass concentration of PST loading was decided based on NIOSH and US-EPA standards. For example, the 8-h time-weighted average (TWA) of water-soluble silicon carbide (non-fibrous) is 10 mg/m3, and the respirable fraction is 5 mg/m3(CitationNIOSH 1994). Moreover, the US-EPA has set primary (health-related) and secondary (welfare-related) National Ambient Air Quality Standards (NAAQS) for particulate matter. The 24-h average mass concentration of PM10 is 125 μ g/m3 (US EPA 2006). Additionally, the mass concentration of PMMA generated by the fluidized bed aerosol generator was about 14.1 mg/m3 (the PMMA generation was not simultaneous with PST/DOP generation). The APS was also utilized to measure the aerosol number concentrations and the size distributions of the test samplers. After the upstream aerosol concentration was measured using a reference sampling probe, the downstream aerosol concentration was measured using the test sampler.
RESULTS AND DISCUSSION
plots the variability of aerosol penetrations of a multi-hole impactor assembled with a conventional flat aluminum impaction plate or an open cavity. Each penetration curve indicates that the multi-hole impactor exhibited severe particle bounce when there was no flat-ring aluminum plate (open cavity only) and challenged with solid PST particles (with no silicone oil coating on open cavity). For particles larger than 4.5 μ m, particle bounce resulted in 20–65% overestimation of penetration. Similar experimental results were obtained even when the open cavity of the multi-hole impactor body was coated with silicone oil (viscosity, 100 mPa.s at 20°C; density, 0.98 g/ml). The designation of the open cavity in this study was not similar to earlier investigations (CitationScott and Ranz 1976; CitationBiswas and Flagan 1988; CitationTsai and Cheng 1995; CitationChang et al. 1999). The dimensions of the open cavity were based on ease of use and were different from those used elsewhere (), facilitating assembly of the flat-ring aluminum plate and filling the TSA. When the flat-ring aluminum plate was assembled with the open cavity and coated with silicone oil, no particle bounce from the plate occurred. However, long-term heavy particle loading remained a challenge, as described below.
FIG. 3 Variability of PST particle penetrations of a multi-hole impactor (sampling flow rate: 3.6 L/min) assembled with a conventional flat aluminum impaction plate or an open cavity.
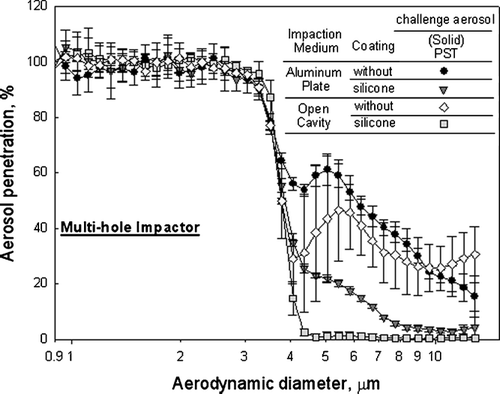
The main distinction between liquid and solid particles is the tendency of aerosol particles to bounce off an impactor as a function of particle size. Impactors typically experience particle bounce while collecting solid aerosols; however, liquid DOP aerosols are not expected to bounce. The liquid challenge DOP aerosol was used to demonstrate non-bounce separation curves, yielding a complete separation curve for a multi-hole impactor for comparison with the solid PST challenge aerosol. plots the variability of aerosol penetrations of the multi-hole impactor versus different challenge aerosols and different impaction mediums. The cut-off size of the multi-hole impactor was approximately 4 μ m; however, the penetration-curve slope was steeper than the ISO/CEN/ACGIH respirable curve when the multi-hole impactor was challenged with PST or DOP particles. In this investigation, however, the multi-hole impactor was developed to demonstrate the method for reducing particle bounce and loading.
FIG. 4 Variability of aerosol penetrations of multi-hole impactor (sampling flow rate: 3.6 L/min) versus different challenge aerosols and different impaction media.
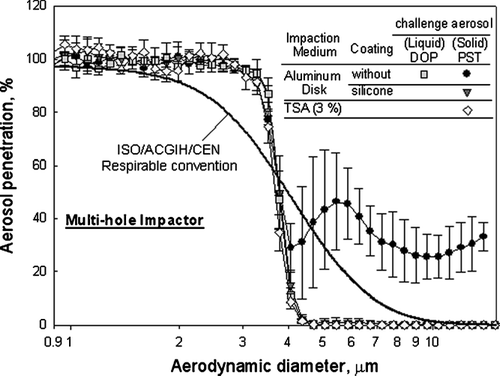
As described, research studies have established that an impaction substrate coated with an adhesive material can reduce particle bounce and re-entrainment. However, following long-term particle loading, the adhesive material cannot continue to prevent incoming particles from bouncing off the impaction plate. Regardless of which adhesive material is used, incoming particles rebound off previously deposited particle layers. The TSA was prepared with water or other solvents with weak chemical bonds such that the discharge of water (aqueous layer) or solvent from TSA could dissolve incoming soluble particles, consequently minimizing particle bounce. The separation curve () obtained when 3% TSA was present in the open cavity, agrees closely with that obtained when the multi-hole impactor was challenged with solid water soluble PST particles: no particles bounced off the soft TSA jelly, as in the case when the flat-ring aluminum plate was coated with silicone oil.
According to experimental results (), 1–6% of TSA exhibited superior efficiency in reducing PST particle bounce. Nevertheless, a mass concentration of TSA of < 1% or > 6% was difficult to prepare. Notably, TSA at < 1% was excessively soft and could not maintain its shape, while TSA at > 6% was rigid and its consistency so firm that it could not be used to fill the impactor open cavity. In view of the fact that TSA is commonly prepared using hot water and continues discharging water due to its weak bond with water, long-term sampling of flowing air may blow away discharged water (aqueous layer) and accelerate TSA dehydration. Therefore, using a high concentration of TSA to fill the open cavity is difficult.
FIG. 5 1–6% of TSA exhibited superior efficiency in reducing PST particle bounce using a multi-hole impactor (sampling flow rate: 3.6 L/min).
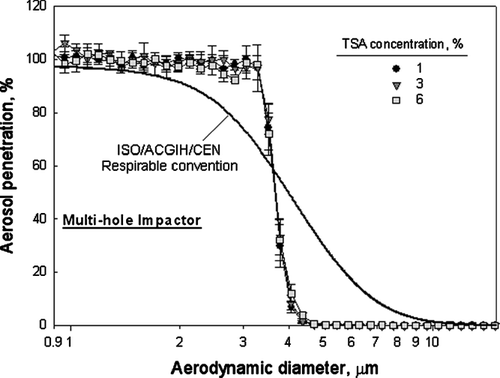
Particles were loaded for 100 min to compare impaction media and coating materials using a multi-hole impactor (). The top-left plot in refers to long-term heavy particle loading when the multi-hole impactor was equipped with a flat-ring aluminum plate. The CMD of the challenge PST aerosols was 5.86 μ m with a GSD of 1.56, and the mass concentration of PST was around 7.22 mg/m3. The closed symbols in represent the initial sampling separation curve of the impactor, and the open symbols represent the sampling separation curve of the impactor obtained after 100 min of particle loading. Particles bounce was initially severe after 100 min of sampling when the aluminum plate was not coated with silicone oil. As expected, solid PST particles initially stuck to the impaction plate; however, the particles bounced after 100 min of sampling (in contrast to the sampling separation curve ()), when the aluminum plate was coated with silicone oil (top right plot in ). Solid water soluble PST particles were dissolved on the aqueous layer of TSA-filled plate before loading; however, significant particle bouncing occurred after 100 min of sampling, even when 3% TSA-filled plate was used (left lower plot in ). The particles bounced and reduced collection efficiency, perhaps because of TSA dehydration after 100 min of sampling. The TSA had shrunk when the TSA-filling cavity was observed. The solid PST directly bounced off the open cavity. Since the distance between the nozzle and impaction surface was reduced, the cut-size and penetration curve, as expected, shifted to a smaller size after particle loading. Therefore, the penetration of small particles decreases as loading increases. However, penetration of large particles increases as loading increases due to bouncing particles. Thereafter, the TSA-filling cavity was coated with a thin layer of silicone oil to prevent dehydration of TSA due to air flow. Solid soluble PST penetrated the silicone layer and was dissolved in the aqueous layer formed between the silicone and TSA. The silicone-TSA double layer minimized PST particle bounce and long-term particle loading (lower right plot in ).
FIG. 6 The PST particles were loaded for 100 min to compare impaction media and coating materials using a multi-hole impactor (sampling flow rate: 3.6 L/min; The CMD of challenge PST particles was 5.86 μ m with a GSD of 1.56, and the mass concentration of PST was around 7.22 mg/m3).
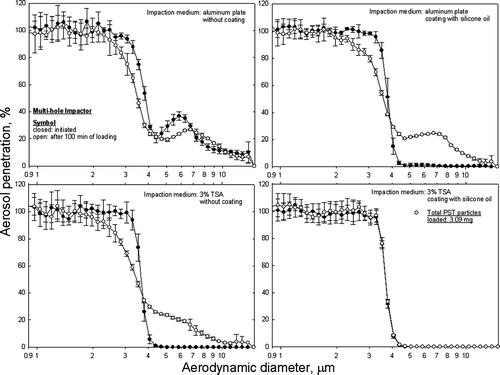
In this study, from the upstream and downstream aerosol concentrations measured by APS, the estimated total loaded PST particle mass for the multi-hole impactor was about 3.09 mg for a 100-min loading. The 100-min loading time for the experiment was decided based on the test system stability and the sampling strategy. Since the mass concentration of challenge aerosol is about 7.22 mg/m3, loading time should not be excessively long to maintain APS sampling efficiency. As incoming particles are always soluble in TSA medium, water solubility dominates the particle loading capacity. Furthermore, for sampling the particle burden in work environments, an 8-h sampling period can be divided into several continuous sampling periods. For sampling ambient environments unburdened with particles, a superior thermostability silicone oil can be used to extend sampling time for a silicone-TSA double layer.
Notably, TSA concentrations of 1, 3, and 6% were compared for long-term particle loading to elucidate the effect of TSA dehydration and the effect of using a silicone-TSA double layer. plots the PST aerosol penetration at 5 μ m during the 100-min sampling versus time in each loading scenario. Previous penetration results in this study show that the amount of PST aerosol that had penetrated at 5 μ m was zero when the flat-ring aluminum plate or TSA-filling was used. Consequently, as particle bouncing occurred, and particle penetration increased was apparent in experimental data. The PST aerosol bouncing at 5 μ m from the aluminum plate was 20–33% without a coating of silicon oil on the plate. The bare flat plate was responsible for 33% of particle bounce, as no sticky material was present to capture the 5 μ m PST particles. However, the percentage of particles bouncing decreased and stabilized after loading for 20 min, perhaps because incoming particles impacted on existing particle layers. The surfaces coated with oil increased adhesion energy, promoted deformation, and dissipated energy, thereby reducing particle bounce. Conversely, the silicone oil layer was too thin to reduce long-term particle loading. Experimental results similar to the bare flat plate were obtained after 20 min of loading: incoming particles impacted previously deposited particle layers, leading to a particle bounce of 20%. Moreover, TSA discharged water and shrunk rapidly when subjected to air flow.
FIG. 7 The PST particle penetration of 5 μ m during the 100 min sampling against time in each loading situation using a multi-hole impactor (sampling flow rate: 3.6 L/min).
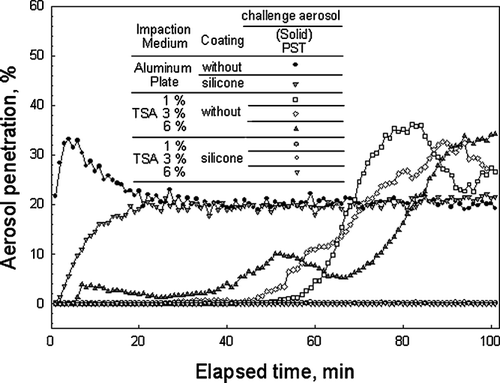
Three concentrations of TSA-filling 1, 3, and 6% and two sampling conditions coated with silicone oil and uncoated were compared for long-term particle loading. No particle bounce occurred for the plates filled with 1, 3, and 6% TSA when TSA-filled plate surface was coated with silicone oil. The 700-μm-thick silicone oil, in the silicone-TSA double layer, significantly resisted dehydration from the TSA-filled plate. Additionally, when PST particles impacted the layer between the TSA and silicone oil, PST was dissolved in water discharged from TSA. However, this discharged water was not blown away by air flow due to the silicone coating the TSA surface. In contrast, the three TSA concentrations were associated with various degrees of 5-μ m particle bounce when the TSA-filled plate was not coated with silicone oil. For 1% TSA, PST particle bounce was observed after approximately 40 min as the air flow blew away discharged water. Similar experimental results were observed for 3 and 6% TSA; the PST particles rebounded in approximately 23 and 5 min, respectively. The increased penetration for each TSA concentration varied with repeated sampling, probably because the shapes of the shrunk TSA differed. However, the effective time associated with decreased PST particle bounce was similar in all cases.
A PEM-PM2.5 sampler was employed to confirm the effectiveness of the TSA filling. In this experiment, the PEM-PM2.5 impactor was expected to exhibit particle bounce as it collected solid PST aerosols when no adhesive coating was present. For particles > 3 μ m, particle bounce was overestimated by 5–15% during solid PST particle sampling (). Conversely, when the porous metal plate was coated with silicone oil and particle loading was minimal, no particles bounced off the porous plate of the PEM-PM2.5 impactor. The cut-off size of the PEM-PM2.5 sampler was around 2.5 μ m (); however, the penetration curve slope exceeded that of the US-EPA PM2.5 curve when the PEM-PM2.5 sampler was challenged with PST or DOP particles. The CMD of challenge PST aerosols was 5.86 μ m with a GSD of 1.56, and the mass concentration of PST was around 7.22 mg/m3. The TSA-filling process was conducted by removing the original porous metal plate from the PEM-PM2.5 impactor and replacing it with 3% TSA. Under minimal particle loading, no particle bounce was observed with 3% TSA, however, a 100-min particle loading could not be achieved because of TSA dehydration (). The TSA discharged water and shrank rapidly when exposed to air flow. Conversely, no other particle-rebound effects were observed when a silicone-TSA double layer was used as the impaction plate and applied to the PEM-PM2.5 sampler during 100 min of sampling. As expected, solid soluble PST was dissolved in the aqueous layer formed between the silicone and TSA. The estimated total loaded PST particle mass of the PEM-PM2.5 sampler was about 8.66 mg for a 100-min loading.
FIG. 9 The silicone-TSA double layer was compared with only TSA-filling using a PEM-PM2.5 impactor (sampling flow rate: 10 L/min; loading PST particles for 100 min).
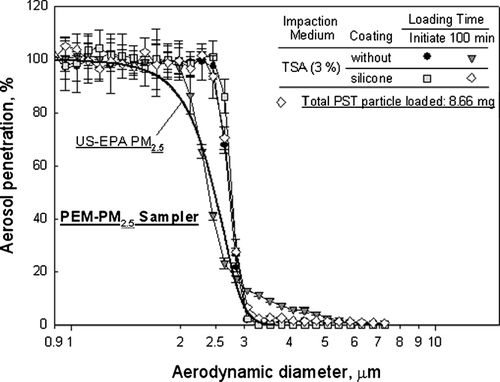
The severe case of particle loading involved the silicone-TSA double layer and water insoluble PMMA aerosols (). The CMD of the PMMA aerosols was 5.17 μ m with a GSD of about 1.28. The mass concentration of PMMA was generated by the fluidized bed aerosol generator at approximately 14.1 mg/m3, illustrating the severe particle loading situation. The silicone oil in the silicone-TSA double layer was a moisture-conserving structure that resisted dehydration from the TSA-filled plate (). The water soluble PST was dissolved by aqueous layer discharged from TSA. However, the silicone-TSA double layer could not dissolve PMMA, which is water insoluble. When 3% TSA coated with silicone oil was used to collect PMMA, the insoluble PMMA bounced off the TSA-filled plate in the multi-hole impactor, resulting in a 20% overestimation of penetration during PMMA sampling. The estimated total loaded PMMA particle mass of the multi-hole impactor was about 4.87 mg for a 100-min loading. In order to show the differences in loading tendency between soluble and insoluble particles, the PST loading results were also plotted in , despite the differences in total loading (3.09 mg total vs. 4.87 for 100 min). Even when the 0.5% TSA was used to minimize PMMA bounce, penetration overestimation was still 10% (estimated total loaded PMMA particle mass of the multi-hole impactor was about 5.48 mg for a 100-min loading). The incoming PMMA particles impacted on hundreds of exposed layers of previously deposited particles. The 0.5% TSA was likely a soft impaction plate; however, the 0.5% TSA was not prepared easily and did not effectively reduce PMMA particle bounce and long-term heavy particle loading.
FIG. 10 The severe case involved the silicone-TSA double layer and solid insoluble PMMA aerosols using a multi-hole impactor (The CMD of challenge PST aerosols was 5.86 μ m with a GSD of 1.56, and the mass concentration of PST was around 7.22 mg/m3. The CMD of the PMMA aerosols was 5.17 μ m with a GSD of about 1.28. The mass concentration of PMMA was approximately 14.1 mg/m3).
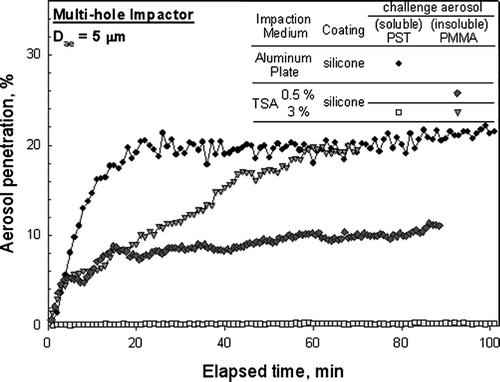
Numerous experimental studies show that under heavy particle loading, low viscosity oil reduces bounce. This capacity is attributable to capillary action through which collected particles are covered with oil; thus, incoming particles are ideally always in contact with the coated plate. However, low-viscosity oil is ineffective for long-term particle loading, especially in a particle-burden situation. The silicone oil was not effectively wicked up via capillary action through previously deposited particles. Since the deposited particles were always accumulated on the impaction plate surface, the silicone oil had limited capillary action. Moreover, low viscosity oil may not be convenient for personal sampling especially when the subject is moving and the sampler may tilt or shake.
Alternatively, a commonly used, soft, semi-solid culture medium, TSA, can be used as an impaction plate. If incoming particles are water-soluble, the TSA water solubility determines particle loading capacity. Experimental results demonstrate that the bouncing effect and heavy particle loading of solid particles was minimized by using TSA in the open cavity as a replacement for a conventional impaction plate. Three concentrations of TSA (1, 3 and 6%) were associated with various degrees of 5 μ m particle bounce when the TSA-filled plate was not coated with silicone oil (). With 1% TSA, the PST particles rebounded in approximately 40 min because the air flow blew away the released water. Similar experimental results were observed for 3 and 6% TSA, where PST particles rebounded in approximately 23 and 5 min, respectively.
However, TSA frequently discharged water due to weak chemical bonding; consequently, the air flow rapidly blew away water discharged from the TSA-filled plate during long-term sampling. To prevent TSA dehydration, silicone oil was coated on the surface of the TSA-filled plate as a moisture conservation technique and, thus, a silicone-TSA double layer was created. When a multi-hole impactor and commercial PEM-PM2.5 impactor were combined with a silicone-TSA double layer in the open cavity as a replacement for the impaction plate, solid particle bounce and long-term heavy particle loading were reduced, as solid soluble PST penetrated the silicone layer and was dissolved in the aqueous layer formed between the silicone and TSA. Unlike PST, water-insoluble PMMA bounced off the 3% TSA-filled plate in the multi-hole impactor. The incoming PMMA particles impacted on hundreds of exposed insoluble PMMA particle layers, resulting in a 20% penetration overestimation during PMMA sampling.
In conclusion, the TSA-filled open cavity can be used to reduce particle bounce and heavy particle loading, since TSA can be prepared using water or another solvent, and the water or solvent discharged from TSA can be used to dissolve particles, thereby minimizing particle bounce. Different solvents can be used to dissolve particles depending on particle composition. The two-layer structure, comprising a solvent-conserving layer and solvent-releasing layer, can be employed to reduce particle bounce and heavy particle loading. However, these novel techniques must be used carefully in ambient sampling at near freezing temperatures. Although antifreeze can be added to the solvent-releasing layer, further experimental proof is required.
Acknowledgments
The authors would like to thank the National Science Council of the Republic of China, Taiwan, for financially supporting this research under Contract Nos. NSC 91-2212-E-040-001 and NSC 93-2815-C-040-001-E. Chung Shan Medical University is appreciated for supporting the patent application.
REFERENCES
- Biswas , P. and Flagan , R. C. 1988 . The Particle Trap Impactor . J. Aerosol Sci. , 19 : 113 – 121 .
- Chang , M. , Kim , S. and Sioutas , C. 1999 . Experimental Studies on Particle Impaction and Bounce: Effects of Substrate Design and Material . Atmos. Environ. , 33 : 2313 – 2322 .
- Chen , C. C. , Lai , C. Y. , Shih , T. S. and Yeh , W. Y. 1998 . Development of Respirable Aerosol Samplers Using Porous Foams . Am. Ind. Hyg. Assoc. J. , 59 ( 11 ) : 766 – 773 .
- Chen , C. C. , Lai , C. Y. , Shih , T. S. and Hwang , J. S. 1999 . Laboratory Performance Comparison of Respirable Samplers . Am. Ind. Hyg. Assoc. J. , 60 : 601 – 611 .
- Dzubay , T. G. , Hines , L. E. and Stevens , R. K. 1976 . Particle Bounce Errors in Cascade Impactors . Atmos. Environ. , 100 : 229 – 234 .
- Esmen , N. A. and Lee , T. C. 1980 . Distortion of Cascade Impactor Measured Size Distribution Due to Bounce and Blow-Off . Am. Ind. Hyg. Assoc. J. , 41 : 410 – 419 .
- Hinds , W. C. 1999 . Aerosol Technology—Properties, Behavior, and Measurement of Airborne Particles. , 2nd ed. , New York : John Wiley and Sons, Inc. .
- Kenny , L. C. and Liden , G. 1991 . Comparison of Measured Respirable Dust Sampler Precision Curves with Sampling Convention . Ann. Occup. Hyg. , 35 : 485 – 504 .
- Lai , C. Y. and Chen , C. C. 2000 . Performance Characteristics of PM10 Samplers under Calm Air Condition . J. Air & Waste Management Assoc. , 50 : 578 – 587 .
- Lai , C. Y. , Chen , C. C. and Hwang , J. S. 2002 . Overall Performance Evaluation of Aerosol Pre-classifier, Adapter and Aerosol Number Samplers . Aerosol Sci. Technol. , 36 : 84 – 95 .
- Lidcn , G. and Kenny , L. 1992 . The performance of respirable dust samplers: Sampler bias, precision and inaccuracy . Ann. Occup. Hyg. , 36 ( 1 ) : 1 – 22 .
- Liden , G. and Kenny , L. C. 1994 . Errors in inhalable dust sampling for particles exceeding 100 micrometres . Ann. Occup. Hyg. , 38 : 373 – 384 .
- Markowski , G. R. 1984 . Reducing blowoff in cascade impactor measurements . Aerosol Sci. Technol. , 3 : 431 – 439 .
- Marple , V. A. , Rubow , K. L. and Behm , S. M. 1991 . A microorifice uniform deposit impactor (MOUDI): description, calibration, and use . Aerosol Sci. Technol. , 14 : 434 – 446 .
- National Institute for Occupational Safety and Health (NIOSH) . 1994 . International Chemical Safety Cards:1061 http://www.cdc.gov/niosh/ipcsneng/neng1061.html
- Newton , G. J. , Cheng , Y. S. , Barr , E. B. and Yeh , H. C. 1990 . Effects of collection substrates on performance and wall losses in cascade impactor . J. Aerosol Sci. , 21 : 467 – 470 .
- Pak , S. S. , Liu , B. Y. H. and Rubow , K. L. 1992 . Effect of Coating Thickness on Particle Bounce in Inertial Impactors . Aerosol Sci. Technol. , 16 : 141 – 150 .
- Rao , A. K. and Whitby , K. T. 1978a . Non-Ideal Collection Characteristics of Inertial Impactors—I. Single-State Impactors and Solid Particles . J. Aerosol Sci. , 9 : 77 – 86 .
- Rao , A. K. and Whitby , K. T. 1978b . Non-Ideal Collection Characteristics of Inertial Impactors—II. Cascade Impactors . J. Aerosol Sci. , 9 : 87 – 100 .
- Reischi , G. P. and John , W. 1978 . The collection efficiency of impaction surfaces: A new impaction surface . Staub-Reinhaltungder Luft , 38 : 55
- Robert , W. V. , Thomas , M. P. , Sanjay , N. , Michael , P. T. , David , B. G. and Russell , W. W. 2001 . Sensitivity Analysis of the USEPA WINS PM2.5 Separator . Aerosol Sci. Technol. , 34 ( 5 ) : 465 – 476 .
- Scott , J. H. and Ranz , W. E. 1976 . Jet-cone impactors as aerosol particle separators . Environ. Sci. Technol. , 10 : 1250 – 1256 .
- Tsai , C. J. and Cheng , Y. H. 1995 . Solid particle collection characteristics on impaction surface of different design . Aerosol Sci. Technol. , 23 : 96 – 106 .
- Tsai , C. J. and Shih , T. S. 1995 . Particles Collection Efficiency of Two Personal Respirable Dust Samplers . Am. Ind. Hyg. Assoc. J. , 56 ( 9 ) : 911 – 918 .
- Turner , J. R. and Hering , S. V. 1987 . Greased and oiled substrate as bounce-free impaction surfaces . J. Aerosol Sci. , 18 : 215 – 224 .
- U.S. Environmental Protection Agency (US-EPA) . 2006 . http://www.epa.gov/reg5oair/naaqs/pm.htm