Abstract
After the anthrax incidents in October 2001, several techniques used for sampling surfaces for biological agents were found to be inadequately validated, especially at low surface loadings. Therefore a test chamber was developed to produce sample sets having targeted surface concentrations of dry biological agent simulant. Dry spore aerosols were initially dispersed into the chamber at relatively high air concentrations, and monitored in real time. The concentration decay (due to stirred settling and dilution) was measured and when the targeted air concentration was reached, the sampling surfaces were uncovered and exposed to the settling particles until >99% of the particles had settled. Multiple agar plates were used to estimate the true colony-forming-unit (CFU) surface concentration. The uniformity of surface loadings was limited by random deposition of small numbers of particles on the surfaces (Poisson distribution) and was characterized by how much greater the observed variability was than that predicted by Poisson statistics. The flow-enhanced powder mixture appeared to affect the spores' ability to grow on the agar medium. Three ways of analyzing the agar plates were used to evaluate the effect of spore coatings on viability and to differentiate between number of spore-containing particles and the number of spores. The presence of spore agglomerates re-suspended by various sample handling activities in the chamber further increased the variability of deposited particles. Based on estimated airborne particle concentration, it was possible to predict mean agar plate concentrations within narrow confidence intervals (CI) at low (4.8 CFU, 95% CI 3.5–6.4), medium (20 CFU, 95% CI 17–23), and high (160 CFU, 95% CI 140–190) concentrations.
INTRODUCTION
The 2001 contamination by dry aerosolized Bacillus anthracis spores in several environments, including the Hart Senate Office Building in Washington, D.C. and post office mail sorting facilities, was measured using a variety of surface sampling and measurement techniques (CitationWeis et al. 2002; CitationDull et al. 2004; CitationSanderson et al. 2004). These spores deposited on various surfaces and eventually had to be cleaned up. The cleanup involved surface testing to determine the presence or absence of culturable spores. Several sampling techniques were applied, involving the use of wet wipes, wet swabs, and vacuuming with special collection sock filters, combined with analysis by spore culturing. The variability of results and lack of validation of these techniques for Bacillus anthracis raised concern regarding the sensitivity and accuracy of these sampling and analytical techniques (CitationTeshale et al. 2002; CitationRose et al. 2004; CitationSanderson et al. 2004; CitationBeecher 2006).
Previous studies have primarily investigated sampling techniques by inoculating surfaces using a high-concentration liquid suspension of bacteria or spores (CitationRose et al. 2004; CitationHodges et al. 2006) or by evaluating environmental samples (Krischner and Puleo 1979; CitationSanderson et al. 2004). These approaches either do not directly simulate the dry deposition of bacteria or spores from the aerosol state or may not have sufficient precision or accuracy to adequately differentiate between techniques. In addition, these studies have not evaluated surface concentrations near the limit of detection as required during certification of cleanliness. Several researchers have tried to address the issue of dry deposition by creating chambers that simulated rooms where exposure might occur. One study (Battner and Stetzenbach 1993) used an experimental room (4 m by 4 m by 2.2 m) and an acoustically fluidized bed to generate spores. The spores were introduced into the room through the air supply registers. Air concentration was obtained using an Aerodynamic Particle Sizer (TSI, Inc., Shoreview, MN) to measure Penicillium chrysogenum conidia (spores) of the size range of 1.8 μm to 3.5 μm. Airborne spore concentration was about 1000 m−3. Four types of airborne samplers were used. RODAC plates were used as the method of sampling surfaces. In the same chamber, CitationButtner et al. (2004) introduced Bacillus atrophaeus subsp. globigii (BG) spores. The spores were allowed to settle onto flooring material placed on a bench. Brown et al. (Citation2007a; Citation2007b; Citation2007c) aerosolized BG spores into a chamber and produced surface concentrations in the range of 102 to 105 colony forming units per cm2.
In the anthrax attack of 2001, some of the material was believed to be in a “fluidized” form (defined here as having fumed silica added). In order to simulate the aerosol-deposited nature of the anthrax biowarfare agent on surfaces, a two part study was initiated. The present work was part of a larger study to investigate surface sampling techniques and analytical techniques. The focus of the work presented here was to develop a system to prepare multiple samples in a chamber, which allowed predictable concentrations of aerosolized spores (closely simulating the type of spores used in the actual attack) to settle on at least two types of surfaces and at concentrations that tested the limits of detection of the sampling and analytical methods. The second part of the study, to be reported elsewhere, produced a range of low concentration surface loadings on stainless steel and carpet surfaces, sampled the surfaces using several techniques, and analyzed the samples using multiple laboratories.
In order to provide multiple surfaces with equal surface loadings of spores so that different surface sampling techniques applied to samples prepared in the same chamber experiment could be compared, a chamber was built to accommodate the number and size of the samples needed. The spores used in this study, both BG and Bacillus anthracis strain Sterne (BaS), were nominally 1 μm diameter, although agglomerated spore particles were expected from the generation system. The BG was initially used to evaluate and improve the performance of the chamber rather than inert particles because of many issues with spore behavior and culturability. Appropriate mixing and conditioning of the aerosol in the chamber had to be achieved to result in uniform loading of a desired concentration on all the horizontal surfaces at the bottom of the chamber.
When an aerosol is thoroughly and continually mixed at low velocity in an air volume and particle settling is the dominant mechanism for deposition, the deposition rate is indicated as “stirred settling” and can be described by the equation
This indicates that if a bolus of airborne particles is introduced into a chamber with thorough and continuous mixing, the particles will fall onto the bottom surface of the chamber at a predictable exponential decay rate, with larger particles settling at a higher rate. If settling is not the primary mechanism, non-uniform deposition may occur. Therefore, care must be taken that the air velocity produced by mixing not be so high that it causes inertial impaction of particles on the chamber surfaces. Diffusional deposition of spore-containing particles 1 μm or larger is negligible compared to that from settling, (CitationHinds 1999, p. 162).
In the present study, the sample surfaces were at the bottom of the chamber and were initially covered. The spores were aerosolized and mixed with the chamber air. The concentration was allowed to decay until the number concentration reached a targeted value and then the sample surfaces were uncovered. The remaining particles in the chamber were allowed to settle onto the surfaces. Note that the settling rate increased with the square of the particle diameter (Equation [2]). Therefore, if the bolus of particles was allowed to settle for a long time prior to exposing the sampling surfaces, smaller particles would predominate, resulting in deposition mostly of single spores rather than multiple spores (clumps or agglomerates). If the time between introduction of the bolus and exposure of the sampling surfaces was short, more multiple-spore particles were expected to be present.
When small numbers of particles are deposited on a surface, the deposit variability on a given surface area can be described by the Poisson distribution. If the spores are deposited in a uniform random fashion, the standard deviation (s) of the number (n) of spores in a given area is the square root of the mean number of particles deposited (
1/2). This is the minimum variability that can be achieved under such deposition conditions. Any other mechanisms causing deposition, such as inertial impaction or electrostatic deposition, are likely to change the rate of deposition and increase the spatial variability of the deposits. Re-suspension of spores from surfaces can produce agglomerate particles that contain multiple spores. In addition, biological factors affecting the growth of the spores on agar plates may also affect the uniformity of deposit.
National Institute for Occupational Safety and Health (NIOSH) guidelines for sampling and analytical methods indicate that for achieving the recommended accuracy of 25% for 95% of the time, the maximum acceptable precision (relative standard deviation) is 12.8% (CitationKennedy et al. 1995). For low counts, Poisson variability limits the precision achievable; e.g., below a CFU count of 61, the 12.8% is thus not even theoretically possible. Further, because of the variability produced by having to deal with live organisms, it is not likely that the accuracy in measurement for spores will meet the NIOSH criteria. In studies of spore recovery from surfaces, the variability of CFUs per sample is not usually reported, only the recovery efficiency and its variability (CitationRose et al. 2004; CitationHodges et al. 2006; CitationBrown et al. 2007a; Citation2007b; Citation2007c). Recovery efficiency values reported in the Brown et al. studies had a range of 28–90%; Hodges et al. 25–91%; and Rose et al. 25–35%. In order to contribute as little as possible to the variability of sampling and analysis results, the goal in this study was to achieve a deposit precision that was as close as possible to that theoretically achievable when small numbers of spores were present and to have the precision better than 25% at higher concentrations.
These principles were used to construct the deposited-sample production chamber in this study as described in more detail below. The goal of this work was to develop a system that produced replicate samples of dry aerosol-deposited biowarfare agents at several low and predictable surface loadings near the limit of detection. In this study, we only addressed the ability to deposit spore particles onto agar plates so that these plates could be analyzed appropriately to represent reference concentrations when compared to surface samples among which the agar plates were interspersed. Some manipulation of other samples occurred concurrently with agar plate testing and results are mentioned that may impact agar reference sample precision.
METHODOLOGY
Spore Preparation
Dried BaS spores were produced as follows: Ten liter (L) fermentation vessels were seeded (5% V/V) with overnight nutrient broth cultures of BaS. Spores were grown in G medium that consists of: yeast extract, 2.0 g L−1; NH4SO4, 2.0 g L−1; Dow antifoam 204, 0.3 mL L−1; MgSO4 ·7H2O, 0.2 g L−1; MnSO4 ·H2O, 0.038 g L−1; ZnSO4 ·7H2O, 0.005 g L−1; CuSO4 ·5H2O, 0.005 g L−1; FeSO4 ·7H2O, 0.005 g L−1; CaCl2 ·2H2O, 0.25 g L−1; K2HPO4, 0.500 g L−1; glucose, 1.0 g L−1. The pH was adjusted to 7.0 ± 0.1 and the glucose was added separately as a sterile solution after autoclaving.
The culture was incubated at 30°C in a 10 L fermentation vessel with an agitation rate of 250 RPM and an aeration rate greater than 0.5 volumes min−1. Sporulation was generally complete within 24 h. Spores were collected by simple centrifugation to remove spent media. The pelleted material was dried by a proprietary azeotropic method. Ten percent (by weight) of an amorphous silica-based flow enhancer was added to the dried spores. The dried material was milled using an exclusionary ball mill. In this process the material passed through a series of stages separated by increasingly finer mesh screens. In each stage 0.01 m diameter steel balls forced the product through the screen separators. A pneumatic vibrator actuated the entire mill.
BG was obtained from a commercially manufactured stockpile of dry spores (produced under contract in 1963 by Westco Chemical, Shafter, CO) that had been used in numerous tests and experiments. The manufacturing process paralleled that of the procedure described for BaS above. Twenty percent by weight of amorphous silica was added to enhance the flow characteristics of the BG preparation.
Media Preparation
Butterfield Buffer with Tween (BBT) was prepared as follows: A Tween 80 Stock Solution (1%) was prepared by mixing 1.0 mL of polyoxyethylenesorbitan mono-oleate 80 (Tween 80) stock solution with 99 mL of water. Butterfield Buffer was prepared using 3 × 10−3 mol/L KH2PO4 and adjusting to pH 7.2 with NaOH. Tween 80 Stock Solution was added to the Butterfield Buffer to give 0.01% Tween 80.
Trypticase soy agar (TSA) reference settling plates were prepared as follows:Plastic Petri plates (100 × 15 mm) (Falcon #35-1029, BD Biosciences, San Jose, CA) were filled to the rim with agar (cat # 7100C, Accumedia Manufacturers, Inc., Baltimore, MD). Filling to the rim appeared to reduce turbulence near the edge of the plates and produced more even particle deposits on the surface. Other analyses were performed using plates filled with TSA II (Becton Dickinson, Franklin Lakes, NJ).
Settling Chamber
The settling chamber and generation system were constructed specifically for this study. The main settling chamber was 1.22 m high with a 1.22 m by 2.44 m cross section (). The main chamber was constructed with static-dissipative Plexiglas® walls and an extruded aluminum framing system (Parker Hannifin Corp., Wadsworth, OH). The edges of the walls were sealed into the framing with gaskets, but were further sealed with conductive silicone caulk. A removable door (0.66 m high by 2.29 m wide), sealed with a rubber gasket and held onto the chamber by toggle clamps, allowed access to the front of the chamber. The integrity of the chamber was tested using theatrical smoke fed into the chamber under positive pressure. The leaks in the chamber were observed using backlighting and these were eliminated where observed. Four glove ports were placed in the door on the front of the chamber. In addition, four horizontally sliding panels were placed inside the chamber to partially cover the door opening. These were used to reduce the opening size when the chamber door was removed; this provided an increase in the inflow velocity (induced by an internal pump) and reduced potential release of aerosol into the room environment. A support system was placed approximately 0.25 m from the bottom of the chamber on which the Plexiglas® (non-static-dissipative) sample shelves were placed. There were six sampling shelves, three on each side of the chamber. Each sampling shelf was a 1.75 cm thick plastic sheet with machined surfaces that allowed placement of the sampling substrates so that the sampling surfaces were flush with the support surface; the sampling locations are indicated in . Each shelf could be moved using a bent metal rod and be brought to the front of the chamber for easy access through the glove ports for sample processing. A raised track allowed movement of the front shelves out of the way while the back shelves were brought forward and processed. With the sample surfaces on these shelves raised above the bottom of the chamber, the effective height of the settling chamber was reduced to 0.98 m.
FIG. 2 Locations of samples on the settling surface. The circles are for agar plates and the large (0.30 m × 0.30 m) and small (0.1 m × 0.1 m) squares are for other sampling surfaces. The dotted lines indicate the six separate sample support shelves that can be moved individually.
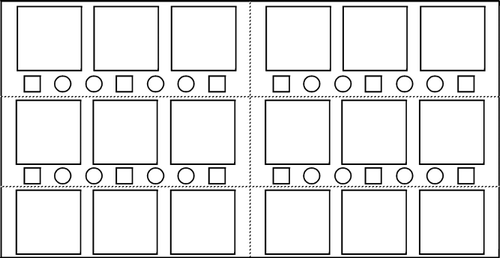
To protect the samples from exposure during times other than the desired exposure period, each sample location was provided with a machined Plexiglas® cover with a tongue that fit into a matching groove on the shelf into which it was inserted prior to and after the exposure period. The grooves were coated with silicone vacuum grease to reduce aerosol infiltration. Locations on the sampling shelves were provided for 46 sampling surfaces of several types: 0.30 m by 0.30 m samples, 0.1 m by 0.1 m samples, and standard 100 mm agar plates (measured diameter of agar surface was 87 mm). The sample surfaces are mentioned here only to indicate that uncovering and covering these samples in addition to the agar plates changed the amount of physical activity inside the chamber.
The chamber had a 0.30 m by 0.30 m HEPA filter installed in the side to equalize the pressure differential between the chamber and room air. Air was pumped from the chamber initially to reduce the aerosol concentration and then at a later time, smaller amounts of air were removed by the aerosol monitoring instrument. The initial phase, after introduction of aerosol but before uncovering the samples, was termed the pump-down phase in the following discussion. The subsequent phase, in which aerosol particles were allowed to deposit by stirred settling, was the settling phase.
The aerosol concentration was monitored using an Aerodynamic Particle Sizer (APS Model 3321, TSI, Inc., Shoreview, MN). The APS displayed an aerodynamic particle size distribution of the chamber aerosol cumulatively in 5 min intervals; the 5 min sampling time allowed reasonable concentration precision at higher concentrations. These data were the basis for determining when to uncover the sample surfaces to begin particle collection.
Four 0.30 m diameter brushless electronic fans (1720 rpm) were used to stir the chamber. The fans were situated along the diagonals of the chamber approximately 0.30 m from the top of the chamber. The fan exhausts were pointed horizontally toward the center of the chamber, though slightly off the chamber diagonals to promote more random mixing. The voltage to the fans was reduced to lower the rotational speed. At consecutive 1 min intervals, each fan was activated for the shortest time possible, approximately 1 s, so that each fan was on once in a 4 min period. This arrangement was used to provide mixing of the aerosol while keeping the air velocity at the sampling surface at a minimum to reduce impaction and re-suspension of spore particles. The fan blades were oiled to reduce re-suspension of impacted particles. It has been shown that an oil with viscosity less than 300 cp, such as WD-40 (WD-40 Co., San Diego, CA), will wick over a deposited particle, covering it, and thus greatly reduce the likelihood of its re-suspension (CitationTurner and Hering 1987).
While the air flow inside the chamber was critical to mixing the aerosol, it was important to determine that the air velocity near surfaces was low enough to ensure that settling was the primary deposition mechanism. The velocity in the chamber was measured at several locations within the chamber using a hot wire anemometer (Model 8360, TSI, Inc.). The most critical flow area in the chamber was that near the bottom surface, where the velocity was below the range of the anemometer. Air velocity approximately 1 cm from the bottom of the chamber was measured by observing puffs of smoke over a grid (2.5 cm square) surface and monitoring the smoke motion with a video camera. The puff velocities were recorded at intervals of about 1–3 s over a 4 min period by manually observing the smoke puffs during several video frames and determining the distance moved.
Spore Aerosol Generation
The generation system was used to aerosolize “fluidized” agent simulant and was constructed adjacent to the settling chamber () using the same enclosure materials. This system served several functions: create an aerosol from a vial of powder, remove the larger particles and clumps, disperse the aerosol into a mixing tunnel, neutralize the aerosol, and feed it into the main chamber where it was further mixed and diluted with the chamber air.
The aerosol generation device was the venturi aspirator taken from a Small Scale Powder Disperser (SSPD, Model 3433, TSI, Inc.). Air flow in the generation system and in the mixing tunnel was provided from a tank of regulated ultra-high-purity nitrogen. The generator could be accessed through glove ports to introduce powder to the generator. During introduction of spore powder to the generator, the generator chamber was sealed and had its own filtered exhaust pump. This pump created a negative pressure and prevented any aerosol from exiting the generation system. The spore preparation was weighed out (2 mg) on a 6 place balance (Model AP250D, Ohaus, Pine Brook, NJ) into a small sealable vial (micro-centrifuge tube, VWR, West Chester, PA). The vial was introduced into the generation chamber and, with the air flow to the SSPD venturi turned on, the powder was carefully aspirated from the vial at a rate that took about 1 min to empty the vial. An impactor placed between the aspirator and the mixing chamber removed particles larger than about 5 μm aerodynamic diameter (Model 266, Sierra Instruments). The aerosol was then injected into the center of the mixing tunnel (0.25 m × 0.25 m × 1.42 m) downstream of the mixing element (). Dilution gas (nitrogen) was injected into the end of the tunnel through an Ion Air Cannon static eliminator (Exair, Inc., Cincinnati, OH) at a flow rate of 150 L/min for about 5 min. The air passed by a square turbulence induction element and mixed with the aerosol from the generator. After completing the injection of the powder aerosol into the mixing tunnel, a valve above the impactor was turned off to seal the generation chamber from the rest of the system. A second static eliminator (Ionizing Point, Exair, Inc.) was situated on the settling chamber wall near the HEPA filter. This provided additional neutralization of the aerosol during the pump-down and settling phases.
The aerosol concentration in the main settling chamber could be reduced either by particle settling alone or a combination of settling and pumping air out of the chamber. The pumped air was replaced primarily with room air coming in through the HEPA filter. Using the loss rate (Equation [2]) and including the pumping rate of the pump,
The spore concentration in the settling chamber was low and it was difficult to collect particles for microscope observation. BG spore powder was aerosolized using an aspirator similar to the SSPD into a separate small chamber (CitationBoundy et al. 2006) and, after an initial decay period, allowed to settle onto an scanning electron microscope (SEM) stub. The SEM samples were observed using a Hitachi scanning electron microscope (Model S4800 field emission SEM, Hitachi, Gaithersburg, MD).
Chamber Operation
The chamber was placed in a room that had exhaust ventilation at the back of the room so that air movement was away from the door and out through the HEPA-filtered house ventilation system. In addition, the door of the chamber was situated next to a HEPA-filtered clean air source (DuoFlow Model H5500, Hazleton Systems Inc., Aberdeen, MD) to minimize the number of room dust particles entering the settling chamber while loading samples into the chamber and while sampling the surfaces.
Agar plates were initially individually sealed in plastic bags and placed in the chamber. The sample covers were placed over the samples. The horizontal surfaces of the chamber and the fan blades were coated with a light oil (WD-40) to reduce particle re-suspension. Additionally, to reduce the concentration of the spores on the bottom of the settling chamber, the entire floor of the chamber was covered with a flexible polyethylene sheet coated with the same oil; this sheet was removed prior to opening the sample covers and was discarded after each experiment. The rubber gloves used to access the sample covers were also coated with the same oil. The chamber was sealed and the air in the chamber was pumped out (inflow through HEPA filter) for about 3 h (approx. 11 air changes) to reduce potential contamination by room aerosol. Blank agar plates were included in all processing except for exposure to spores in the chamber to check for contamination.
After aspirating the dry spore particles, the aerosol was mixed with dilution air, neutralized, and fed into the main chamber. The chamber was evacuated for 20 minutes or more using a vacuum pump to reduce the concentration in the chamber. This reduced the time required to achieve the target concentration.
The APS measured the size distribution of the aerosol in the chamber in 5 min increments so that the aerosol concentration decay could be followed. The initial particle number concentration in the chamber ranged from 30 to 70 total particles/cm3 as measured by the APS. This concentration was allowed to decay to, e.g., 0.5 total particles/cm3, of which approximately 0.01 particles/cm3 were 1 μm particles, at which time the settling phase commenced. Additional agar plates were placed in available sampling locations to improve statistical confidence in the results. The APS count monitored during each BaS experiment was the sum of six APS channels (1.114 μm to 1.596 μm). The lowest channel was chosen to minimize non-spore particle detection at the lower end of the BaS spore distribution.
The relationship between the APS particle count and the colony forming unit (CFU) count on exposed agar plates was determined from several experiments with each species of spore (data not presented). An algorithm, based on the exponential observed concentration decay curve for each experiment and updated after each APS measurement, was used to predict the optimum time to remove the sample covers based on the target concentration. This was accomplished by combining the logarithm of the target concentration, the logarithm of the APS concentration in each 5 min period, and the current time using the FORECAST function in an Excel spreadsheet (Microsoft Inc., Redmond, WA). This was an improvement over using the real time APS concentration, since these individual measurements had considerable variability at low concentrations. At the appropriate time, the agar plates were placed in their holders on the sample shelves and their covers removed.
After the 10 h settling period, the agar plate covers were replaced and the vacuum pump was turned on for about 1 h to further reduce the chamber concentration. After this final pumping stage, the agar plates were removed for cold storage or immediate analysis. The horizontal surfaces were washed with bleach and left for 30 min to air dry.
After removal of all the agar plates, the chamber was sterilized by vapor hydrogen peroxide (VHP) injection into the settling chamber using a sterilizer Model VHP1000 (Steris Corp., Mentor, OH). The VHP was subsequently outgassed from the chamber surfaces by heating the chamber for about 80 h, keeping the shelf surfaces and covers at 38°C to 50°C during this period. This outgassing procedure ensured that residual hydrogen peroxide levels did not interfere with subsequent spore experiments (CitationBaron et al. 2007).
Reference Sample Analysis and Experiments
Nine consecutive experiments were performed using BG or BaS to evaluate the agar plate analysis techniques and evaluate uniformity of deposition in the chamber. In these experiments, additional agar plates, for a total of 25 to 34 plates, were placed in the chamber instead of just at the 16 locations indicated in . After removal from the chamber, the agar plates were initially processed by heating to 35°C to 37°C for 12.5 h to allow the spores to grow. Agar plate results were compared to the APS monitoring data and it was determined that up to 98% of the BG spores were not culturable using this technique.
A second set of experiments was performed with BaS using two additional procedures in an attempt to better understand the surface concentration of culturable spores. The second method involved dispersing the spores using a spreader with 100 μL of BBT solution for 1 min while rotating the agar plate on a turntable prior to processing. The rationale behind this step was to break up any clumps of spores present in the sample in order to yield a concentration more similar to the total individual spore concentration measured on the test surfaces. A third approach involved building a small cylindrical chamber 25 cm high with a diameter just larger than that of the agar plate; a portable medical nebulizer (Model NE001-1, AirSep, Buffalo, NY) was attached; and BBT solution was nebulized into the chamber and allowed to settle onto the surface of each agar plate for a period of 1min. This produced a visible liquid coating on the agar surface. This approach ensured that each spore on the plate was exposed to and in contact with growth medium. The incubation of these samples was the same as for the other techniques above. The three agar plate analysis techniques are referred to as “settling,” “spreading,” and “misting” analyses, respectively, in the following discussion. In some of these experiments, the effect of leaving the agar samples overnight enclosed in their holders was evaluated.
Finally, when it was felt that all the significant issues with the chamber had been addressed, nine additional experiments were carried out using BaS: three experiments each at low (∼ 2 CFU/plate), medium (∼ 20 CFU/plate), and high concentrations (∼ 200 CFU/plate). In this final set of experiments, the “spreading” agar plate analysis technique was used and agar results were compared to the APS particle counts in order to estimate the culturable fraction.
Culturable fraction (fraction of airborne spore-containing particles resulting in a CFU) was estimated for the BaS experiments at low, medium, and high concentrations. The airborne particle concentration was estimated using the sum of six APS channels through the process described below. As indicated in , the small particle mode (only partially detected by the APS), overlapped with the spore mode (peak at about 1.3 μm). Therefore, the 6-channel APS count only covered a portion of the total spore mode. It was assumed that the particle size distribution of single-spore-containing particles was lognormal at the time of generation, but that the distribution was modified over time by decay as dictated by Equation (Equation1). The size distribution of spores remaining when sample exposure started was assumed to be that depositing on the surfaces during the subsequent 10 h period.
FIG. 3 Example size distributions of (a) BG and (b) Bacillus Anthracis subs. Sterne (BaS) as measured by the APS after injection of spores into the chamber. The distributions in each figure represent the peak concentration after injection and the concentration at the indicated time period later. The decay in concentration is caused by a combination of pumping and gravitational settling.
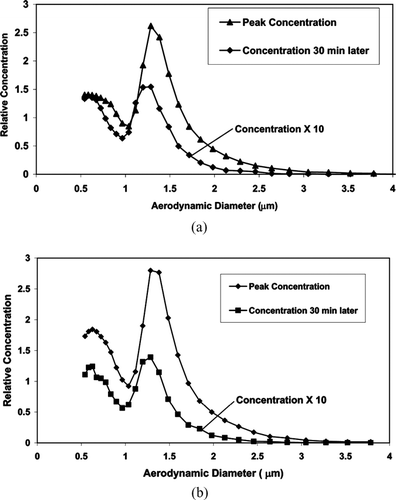
The total spore mode concentration was estimated by fitting two lognormal distributions (one for the small particle distribution and the other for the spore mode), modified by the settling that occurred prior to the sampling period, to the observed size distribution (). The integrated spore mode concentration (Ctotal, particles/cm3) was then used in the following calculation. The 6-channel APS-measured concentration (CAPS, particles/cm3) represented a fraction (F) of the entire spore mode concentration, so that F = CAPS /Ctotal. F was determined for each experiment because the relative size of the spore and small particle modes differed principally due to differing settling times.
FIG. 4 Example of curve fitting process described in text for estimating the total number of single-spore-containing particles from the APS data. This example uses two lognormal curves modified for settling that are summed up to fit the APS size distribution data. For the prediction values, only data from the range indicated (1.114 to 1.596 μm) are used. The fitted APS size distribution was deconvoluted to estimate the total number of spores in the spore particle mode. In this example, the measured APS range was 85% of the entire spore distribution.
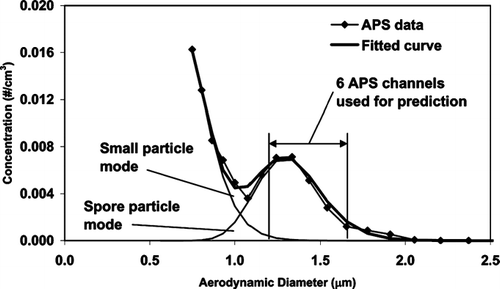
The target concentration for each experiment was used in the decay fitting calculation to determine the time at which the chamber concentration was at the correct level. Since the sample surfaces were uncovered at this time, the target concentration, CTarget, also represented the best estimate of the chamber concentration at this time, and, when combined with the chamber volume, it also allowed calculation of the best estimate of the surface concentration ultimately deposited on the chamber floor. The number of particles that settled onto each agar plate was CTarget times the volume of air above the agar plate (V ap = area of agar plate times the height of the chamber). Thus, CTarget was both the target concentration and the number used to estimate the deposited particle number concentration. It was assumed that the entire spore mode represented the true concentration of spore containing particles. It was then possible to calculate the ratio of the mean number of CFUs observed on the agar plates (CFU ap , measured by spreading) to the estimated total number of airborne spore-containing particles that would have settled on those plates. This ratio was termed the culturable fraction (FCult).
Statistical Analysis
SAS 9 Software® (SAS Institute Inc., Cary, NC) was used to perform all statistical analyses. Analyses included simple descriptive statistics for the agar results in each experimental run of the chamber. Variability was estimated using the relative standard deviation (RSD = sample standard deviation/ sample mean) which was compared to the RSD expected assuming that deposition in the chamber was governed by a Poisson process for which the variance is equal to the mean (i.e., RSDPoisson = (sample mean)1/2/sample mean, or 1/(sample mean)1/2).
For the final set of BaS experiments, 95% confidence intervals for the mean agar count were estimated using Poisson confidence intervals (estimated using the GENMOD procedure in SAS by specifying the Poisson distribution and a logarithmic link function; to account for over-dispersion, the covariance matrix was scaled by the deviance divided by the degrees of freedom). Negative binomial regression modeling (via the GENMOD procedure in SAS by specifying a negative binomial distribution and a logarithmic link function) was used to relate the agar plate counts to CTarget. The negative binomial regression model, which allows the variance to be greater than the mean through the introduction of a dispersion parameter, was used instead of a Poisson regression model since there was evidence of overdispersion (i.e., the variance was larger than expected based on the Poisson distribution). The dependent variable was the agar plate count and the independent variable was the natural log transformed CTarget. The model predicts mean counts according to the following equation:
RESULTS
The APS size distribution measurements of the aerosolized spores indicated a peak at approximately 1 to 1.5 μm as expected for single spores (). The decay of spore particle concentration in the chamber was followed over time as exemplified for a single size (1.286 μm APS channel) by . The curve indicates an initial rise in concentration during the aerosol injection phase, a rapid decay during the pump-down phase and a slower decay during the particle settling phase. The variability in an individual concentration measurement increased significantly toward the end of the settling phase because of counting statistics. Note that a concentration of 0.0002 particles/cm3 represents a single APS particle count in a 5 min period. Accumulation of all the APS measurements and use of the forecasting function allowed a more accurate estimate of concentration late in the settling phase.
FIG. 5 Concentration decay of 1.3 μm (one APS channel) particles of silica-coated BG spores as measured by the APS. These data are compared with the predicted settling rate of these particles as well as the fitted curve after the pump was turned off (primarily particle settling). The initial increase in measured concentration occurs when particles are first injected into the chamber and mixed with the background air. The measured decay rate corresponds to a particle aerodynamic diameter of 2.1 μm. The higher than predicted decay rate may be an artifact of the APS measurement or other losses in the chamber (see text).
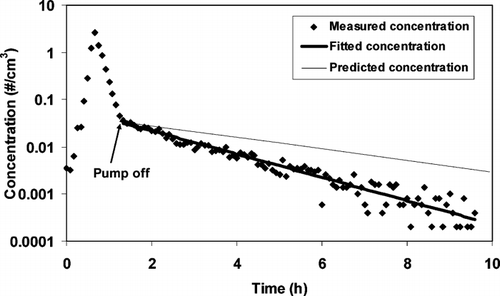
An example decay curve for the six APS channels is plotted in . Another group of 12 channels (2.129 μm to 4.698 μm) representing a larger particle range is also plotted in same figure. An increase in concentration was noted when the agar plates and other samples were being uncovered and again, to a lesser extent, when they were re-covered.
FIG. 6 Decay curve of particles detected by the APS in two size ranges. The sum of six channels (1.114–1.596 μm) covering most of the spore containing particles and the sum of 12 channels (2.129–4.698 μm) that represent larger particles. Physical activity in the chamber caused additional particles to be aerosolized in the chamber, as can be seen from concentration spikes immediately following sample uncovering and covering.
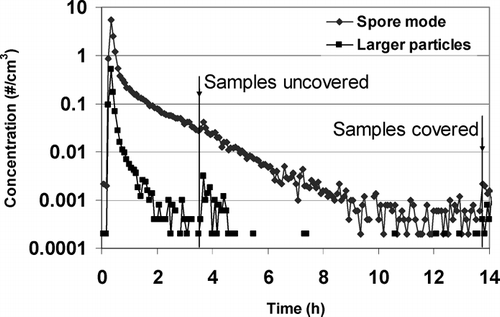
A picture of a particle presumed to be a single BG spore coated with fumed silica is indicated in . Many particles similar to this were observed, while no single spores without the silica coating were observed. However, agglomerated spores with only a light coating of silica were readily observed as indicated in .
FIG. 7 Pictures of spores (BG) agglomerated with fumed silica. (a) Particle assumed to contain a spore coated with fumed silica. The scale markers are at 0.1 μm intervals. (b) Spore agglomerate with small amount of fumed silica attached. The scale markers are at 0.2 μm intervals. (Courtesy of M. Hernandez, Hitachi High Technologies America, Inc.)
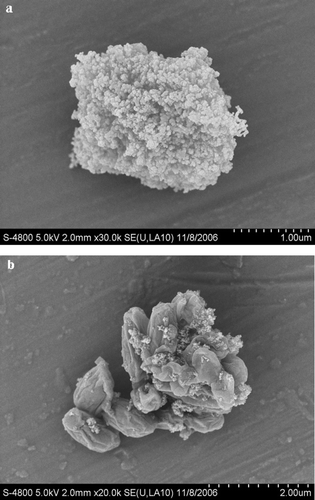
The highest air velocity measured in the settling chamber was directly in front of the fans, i.e., 0.9 m/s. Because each fan was only on momentarily during each 4 min period, the air velocity decayed to below the detection limit of the anemometer in just under a minute. Thus, there was measurable air flow in the upper part of the chamber nearly all the time. The air velocities near the bottom of the chamber (from video monitoring) ranged from virtually stagnant to momentary peaks of about 0.25 m/s (resulting from the flow pulse when the fans were turned on), with a mean velocity of 0.055 m/s (standard deviation 0.045 m/s).
presents agar plate data from nine consecutive experiments in which the agar plates were processed by the settling method. The observed variability averaged about 20% greater than theoretically predicted for the Poisson distribution. Blank agar plates gave negligible concentration (0 or 1) and background was assumed to be zero in the subsequent data analyses.
TABLE 1 Data from agar plate settling counts within a set of consecutive chamber experiments.Footnote a
The CFU concentrations from several experiments were compared using agar plate analysis by settling, misting, and spreading. The results from the initial reference sample analysis with BaS comparing data obtained by settling, misting, and spreading are indicated in . The ratio of average concentrations of misting CFUs/plate over settling CFUs/plate for BG was approximately 20 (data not presented), while for BaS it was approximately 1.3. The concentration data for misting and spreading in the BaS experiments were plotted as a frequency plot of the number of agar plates in each concentration range (or concentration bin); an example is shown in .
TABLE 2 Data from agar plate settling, misting, and spreading counts within a set of consecutive chamber experiments for Bacillus anthracis, species Sterne.
FIG. 8 Frequency plot of agar plate data (from Experiment 6 in ) showing the difference between the misting and spreading techniques. The distribution for the spreading analysis is more skewed to higher concentration than the misting, suggesting the presence of large clumps on some of the plates. This skewness frequently causes the spreading data standard deviation to be dramatically higher than that for the misting data. Note that the highest CFU point in the spreading curve represents the two off-scale values (124, 180 CFU) indicated.
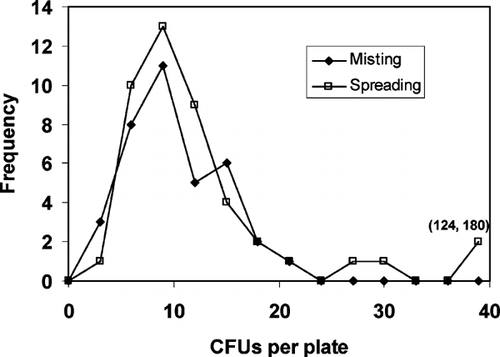
In the course of these experiments, the spores were allowed to remain on the agar plates in the chamber at room temperature during the settling phase for up to 22 h. It was observed that when the settling time was 14.5 to 16.5 h (experiments 2, 4, 5, and 6 in , e.g., ), the misting and spreading data agreed well except for a few agar plates that apparently had large spore clumps on them. When the settling time was 19.5 h or greater (experiments 1 and 3 in , e.g., ) the spreading data showed a shift in the mode that suggested growth of settled spores had occurred during the spore deposition period.
FIG. 9 In this frequency plot of CFU data (from Experiment 1 in ), a comparison of misting and spreading demonstrates an increase in spore concentration when spores rest on the agar surface for sufficient time; in this case, the plates sat in the chamber at room temperature for 19.5 h during sample exposure. The mode for spreading indicates about twice the number of spores per CFU observed with misting.
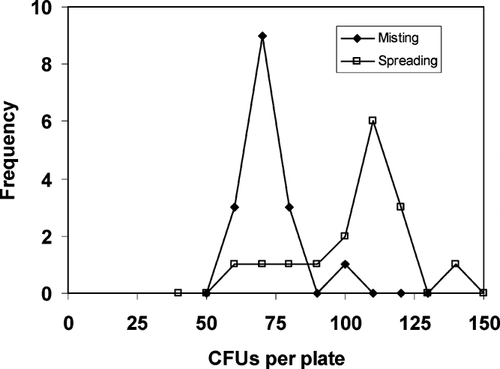
The data from the final experiment using BaS at low, medium, and high concentrations are indicated in . These data also have the corresponding measured and theoretical standard deviation as well as the estimated culturable fraction. The resulting 95% confidence intervals for the mean CFU count per agar plate are indicated in . As for other parameters, the data were evaluated with and without outliers. All the agar plate CFU data also have been plotted in against the APS particle concentration selected to determine the opening time of the sampling surfaces.
TABLE 3 Data from agar plate settling counts within a set of consecutive chamber experiments for Bacillus anthracis, species Sterne.Footnote a
FIG. 10 Comparison of all agar plate CFU data from nine experiments () with estimated airborne particle concentrations based on APS counts. Note that CTarget is at the same time the target concentration and also the best estimate of the airborne concentration to which the agar plates were exposed as explained in the text. The bars at each APS concentration represent the mean at that concentration. The heavy line represents the predicted mean CFU concentration = 888 (C Target )0.947. The lines on either side are the upper and lower 95% confidence bounds. The bars represent the mean concentration for each experiment with no outliers removed.
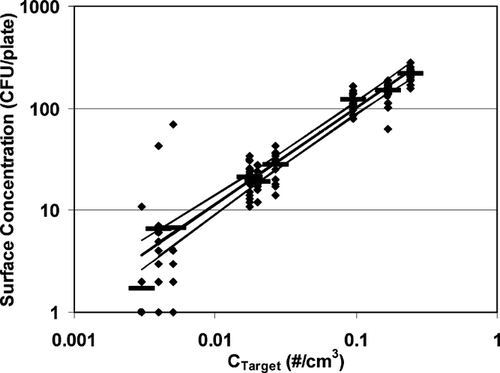
In order to estimate the ability to predict the mean concentration in a future experiment, the data from the nine experiments in were used. A negative binomial regression model gave a relationship Mean CFU = 888 (CTarget)0.947. The negative binomial model fit the data much better than the Poisson model because of the over-dispersion of the data. Predicted mean agar plate counts based on this model are 4.8 CFU (95% confidence interval 3.5–6.4), 20 CFU (95% CI 17–23), and 160 CFU (95% CI 140–190) for future experiments at low, medium, and high concentrations, respectively.
DISCUSSION
Aerosol Particle Size and Concentration Decay
The aerosol generated from the BG and BaS powders had a mode at about 0.9 and 1.3 μm, respectively (), indicating that most of the particles initially generated were likely to include single spores. The maxima of these modes decreased slightly at later times in the settling process as predicted by Equation (Equation2) (larger particles settle more quickly). The remaining aerosol consisted of particles containing no spores (only debris or non-culturable spores), particles containing single spores and particles containing multiple spores. It was assumed that the generated organic material had a density close to 1 g cm−3. However, the spores were mixed and coated with a flow enhancement agent, fumed amorphous silica with a higher density. The BG spores, stored for more than 40 years, appeared to be more culturable by spreading than by settling (by a factor of about 20); the BaS spores were more culturable by spreading than settling by a factor of about 1.3. shows a particle potentially containing a single BG spore; since no uncoated single spores were observed, this suggests that virtually all single spores remained coated with silica. The coating apparently solidified from exposure to water in the air over the years of sample storage and use. However, multiple spores or clumps were found frequently and these were often largely uncoated as indicated in . The reason for the difference in coating adherence to different sized particles is unclear. The BaS spore particles were not analyzed by SEM because of potential safety issues.
It was estimated that the particles indicated in , measured as 1.3 μm using the APS, decayed by settling at a rate consistent with particles that were actually 2.1 μm aerodynamic diameter (). This increased decay rate of particles in the chamber could have been caused by several factors, including underestimation of particle size by the APS, electrostatic deposition in the chamber, or impaction on fan or other surfaces (CitationThatcher et al. 2002). The latter two loss mechanisms were reduced to the extent possible. Nothing could be done to change the APS measurement system. The APS accelerates particles to about 150 m/s in the detector and this acceleration may be enough to affect the adhesion of the silica particles to the spore and to each other (CitationBaron et al. 2001). Thus, the detection process may strip some of the silica from the spore and the resulting particle may be detected as smaller than the particle as it might settle in the chamber. This was not directly observed, but provides one potential explanation of why the settling rate (or loss) of the particles in the chamber was higher than predicted based on the APS-measured diameter (Equation [Equation4]).Electrostatic effects were reduced by neutralizing the aerosol as it was generated and in the chamber using ion sources and by reducing charge on plastic surfaces using anti-static spray. Further, the fan rotational velocity was reduced by 20%; impaction increases as the air velocity squared, so this velocity reduction should have significantly lowered impaction as well as re-suspension. Both particle deposition and re-suspension have been demonstrated to increase with particle size and air velocity in filters (CitationQian et al. 1997). It is expected that the same mechanisms apply to other surfaces such as fan blades.
Ideally, the particle decay in the chamber should follow the trend indicated in . However, the actual decay during an experiment in which samples were opened often had a noticeable spike in concentration at the time the samples were uncovered and also when they were covered (). These additional particles were apparently caused by the activity inside the chamber in spite of the precautions indicated. These spikes in concentration ranged from virtually unnoticeable to almost a factor of two. This evidently contributed to the variability of the deposited particles. The slight increase in particle concentration in the spore mode was not enough to significantly affect the prediction capability of the APS based on prior concentration data as evidenced by . However, the additional particles may have contributed to the increased variability, especially since the larger particle concentration increase was proportionately greater than for the smaller particles (). The data from settling analysis in experiments indicated in and exhibited less variability that those in . This lower variability in the initial experiments probably occurred because only agar plates were uncovered and covered as opposed to agar plates and the other samples in the latter experiment. The presence of a small number of larger particles caused by the disturbance of additional manipulations inside the chamber was probably the source of the outliers since re-suspended particles were more likely to be agglomerated spores. Although further work to eliminate this source of undesirable particles was not carried out because of time constraints, the use of the APS to detect these particles should be a useful technique for future improvement of the chamber operation.
also shows that at very low concentration (long settling times), the APS indicates a low background concentration. We have no evidence as to the nature of these particles, but it is possible that they were introduced through ambient air leaks into the chamber.
Agar Plate Analysis
There was a consistent trend in the BaS experiment data, with the settling, misting, and spreading data having increasing concentration and increasing standard deviation in that order. These techniques all measure culturability, though under different conditions. Our interpretation of the effect of these three analysis techniques is as follows. The settling technique, commonly used for sampling bioaerosols, allows the spore-containing particle to interact with the agar media leaving the spore coating largely undisturbed. This means that if the spore particle is completely coated with silica, it may not have sufficient contact with the growth medium to grow and be detected as a CFU. However, when the spore does grow, only a single CFU is detected for a particle even when the particle contains more than one spore. With the misting technique, the deposited particles are coated with a liquid layer that either washes off the coating from the spore or provides better contact between the spore and the growth medium. Misting thus provides a better estimate of the total number of particles that contained culturable spores. In this case, all spores are likely to grow, but multiple spores within an agglomerate will still be counted as a single CFU. With spreading, not only is the spore particle washed with liquid that can remove the coating, but the mechanical action of spreading can separate clumps or agglomerated spores. This provides a better estimate of the total number of spores. The spreading technique is thought to be most similar to the process by which samples are collected from surfaces and then analyzed by using a liquid suspension of the sampled particles.
During the development of the chamber operation procedures, the comparison between the spreading and misting data provided information about the presence of agglomerates that were deposited on the surfaces. These agglomerates represented contamination from a process other than settling, since only particles containing single spores should have settled on the surfaces based on the settling decay in the chamber (Equation [Equation1]). is an example of such a comparison, showing a frequency plot of CFU concentrations on the agar plates. The frequency plot peak from the misting and spreading data overlap, but in the spreading data there are several agar plates with much higher concentration. This indicates that a few large clumps with between 25 and 180 spores were present in these plates. These experiments were carried out before the oiling of all surfaces was added to the procedures. Hence, later experiments () had fewer large clumps.
When spores deposit on a growth medium such as agar, it is likely that the spores will begin to grow after some period, even at room temperature. Data provided by L. CitationRose (2006) indicated that when a suspension of BaS spores was deposited on agar plates, significant multiplication of spores occurred after sitting at room temperature for 12 h, but not after 9 h or less. In the case of the experiments in the settling chamber, the situation was somewhat more complex. The spores were coated with fluidizing agent that reduced contact between the spores and the growth medium, possibly reducing the growth rate during the time after the spores had settled on the agar surface. In addition, all the spores did not deposit on the agar medium at the same time, though most deposited in the first few hours of settling. Several experiments in which the settling time was kept below 16.5 h (e.g., ) indicated that the misting and spreading distributions were quite similar, except for several higher count plates in the spreading distribution (caused by spore agglomerates). When the settling time was 19.5 h or greater (e.g., ), the spreading data mode clearly shifted to a higher CFU concentration, indicating that the spores had multiplied by two or more in each particle. Thus, it was decided to keep the settling time in subsequent experiments to less than 12 h to ensure that no growth occurred prior to incubation of the samples.
Chamber Mixing and Surface Deposit Uniformity
Several studies on particle settling in indoor air suggested that sufficiently low air velocity is critical to ensuring particle deposition primarily by settling versus impaction (CitationByrne et al. 1995; CitationLai and Nazaroff 2000; CitationThatcher et al. 2002). The velocity measurements made near the bottom of the settling chamber indicated a mean air velocity of about 0.055 m/s, which was quite similar to measurement conditions (0.054 m/s) used by CitationThatcher et al. (2002) to simulate particle deposition onto smooth surfaces. This velocity produced deposition rates similar to those predicted theoretically for particle settling.
Initial measurements were made using BG spores as the generated and measured aerosol. The results for settling analysis from nine consecutive experiments () include the relative standard deviation for each experiment, as well as the relative standard deviation for truly random particle (Poisson) deposition as estimated based on the observed mean plate count. The data typically had higher variability than indicated by the Poisson model; this increased variability is termed over-dispersion. The percentage of the observed standard deviation compared to the Poisson standard deviation was taken as an index of success in achieving purely random deposition, with 100% being the desired goal. Note that most experiments were close to achieving this (≤129%), while one gave more than twice the variability (222%) of a random deposit. While this was not ideal, the range of variability observed was considered acceptable for the study. As indicated above, we are not aware of any other studies achieving this level of precision in deposited spores, especially at such low concentrations. Other steps in the measurement, such as sampling and processing of the surface samples, were expected to contribute more to the measurement variability than indicated by these levels.
After these data were obtained, it was discovered that the agar plates were inefficiently detecting the number of CFUs collected (analysis by settling only). This was discovered using the spreading analysis technique described above. The precise fraction of non-germinating spores was not determined because the spreading samples were too numerous to count; however, it was estimated that the non-cultured fraction by settling was >95%. It was surprising that settling counts were so consistent with such a large fraction of non-cultured spores.
summarizes agar plate CFU data for BaS that were obtained during experiments after various problems with the system had been sorted out. The medium and high concentration count distributions could be approximated by either a normal or lognormal distribution, the standard deviations were within 250% of the predicted Poisson variability (with outliers removed). Note that a Poisson distribution can be approximated by a normal distribution when the count is large. There were insufficient data in the low concentration experiments to evaluate the type of distribution. When outliers were removed (), all the relative standard deviations were less than 235% of that expected for Poisson deposits. This indicated that the settled particles were probably being deposited close to a uniform random manner. The presence of outliers suggested the presence of re-suspended particles that increased the variability of the deposits. These outliers cannot be ignored when comparing with other surface samples because these samples would be exposed to similar re-suspension activities in the chamber. Further work is needed to trace down and eliminate the source of the re-suspended particles.
The prediction of the CFU data based on the APS measurement of airborne particles appeared to work well as indicated by over at least two orders of magnitude in concentration. The means of the medium and high range samples were predicted within ± 20%, while the low range samples were predicted to within ± 35%. The ability to predict the surface concentration of spores was critical to the success of the chamber operation, allowing generation of samples appropriate for each experiment.
The culturability data in were determined based on CTarget. The medium and high concentration experiments had an average viability of 0.16 with a range of 0.098–0.23. The range for the low concentration values was somewhat higher (0.066–0.31). The low concentration data have limited precision because of the small number of CFUs on each agar plate. The calculation of the culturability was based on assumptions about the shape of the size distribution and thus had additional uncertainty associated with the values reported.
CONCLUSIONS
A biological agent aerosol generation system producing aerosol particles from dry powder and a chamber for collecting replicate surface samples of agent particles at low concentrations were constructed to simulate real world sampling situations. The entire system was made operational under safe and controlled conditions. The use of aerosol monitoring with the APS allowed prediction of mean surface concentrations over a range of two orders of magnitude. Several techniques were used to compare the number concentration of particles in the chamber air, the number of spore-containing particles settled onto agar surfaces, and the total number of spores deposited on agar surfaces. The higher than predicted sample variability and occasional high values produced by spreading agar plates with BBT were probably largely caused by re-suspension of spore agglomerates from the mixing fans or other surfaces, especially during sample handling activity inside the chamber. This relatively high variability and the outliers may be controlled in future experiments by more careful control of surfaces during activity inside the chamber. However, the achieved level of variability was considered acceptable for testing the surface sampling procedures when compared to other spore deposition studies and to the variability of the analytical techniques used for surface sampling.
Disclaimer. The findings and conclusions in this report are those of the authors and do not necessarily represent the views of the National Institute for Occupational Safety and Health.
Notes
a All agar plates processed via the settling method.
b BG = Bacillus globigii; BaS = Bacillus anthracis, species Sterne.
c RSD (relative standard deviation) = standard deviation/mean.
d Poisson RSD = expected standard deviation/mean = mean1/2/mean = mean−1/2.
e Percent of Poisson = 100% × RSD/Poisson RSD.
f Values in parentheses are for two outliers removed.
a Agar plates sat for ≥ 19.5 hours prior to processing for experiments 1 and 3 and for 14.5–16.5 hours for experiments 2, 4, 5, and 6. No outliers (high values in spreading) were excluded.
b RSD (relative standard deviation) = standard deviation/mean.
c Poisson RSD = expected standard deviation/mean = mean1/2/mean = mean−1/2.
d Percent of Poisson = 100% × RSD/Poisson RSD.
a All agar plates processed via the spreading method; n = 16 agar plates per experiment.
b 95% confidence intervals for the Poisson mean were obtained using the GENMOD procedure in SAS (distribution = Poisson, link function = log). To account for over-dispersion, the covariance matrix was scaled by the deviance divided by the degrees of freedom.
c RSD (relative standard deviation) = standard deviation/mean.
d Poisson RSD = expected standard deviation/mean = mean1/2/mean = mean−1/2.
e Percent of Poisson = 100% × RSD/Poisson RSD.
f Culturable fraction = (F × CFU ap )/(CTarget× V ap ) where F is the fraction of the entire spore mode represented by the APS measured particles in the 1.114 μm to 1.596 μm range, CFU ap is the mean agar plate count, CTarget is the target concentration, and V ap is the volume of air above the agar plate (area of agar plate times the height of the chamber).
g Values in parentheses are for one outlier removed.
REFERENCES
- Baron , P. A. , Estill , C. F. , Beard , J. K. , Hein , M. J. and Larsen , L. 2007 . Bacterial Endospore Inactivation Caused by Outgassing of Vaporous Hydrogen Peroxide from Polymethyl Methacrylate (Plexiglas®) . Lett. Appl. Microbiol. , 45 : 485 – 490 .
- Baron , P. A. , Mazumder , M. K. and Cheng , Y. S. 2001 . “ Direct-Reading Techniques Using Particle Motion and Optical Detection ” . In Aerosol Measurement: Principles, Techniques and Applications , Edited by: Baron , P. A. and Willeke , K. 495 – 535 . New York : John Wiley & Sons .
- Beecher , D. J. 2006 . Forensic Application of Microbiological Culture Analysis to Identify Mail Intentionally Contaminated with Bacillus anthracis Spores . Appl. Environ. Microbiol. , 72 ( 8 ) : 5304 – 5310 .
- Boundy , M. , Leith , D. and Polton , T. 2006 . Method to Evaluate the Dustiness of Pharmaceutical Powders . Ann. Occup. Hyg. , 50 ( 5 ) : 453 – 458 .
- Brown , G. S. , Betty , R. G. , Brockmann , J. E. , Lucero , D. A. , Souza , D. A. , Walsh , K. S. , Boucher , R. M. , Tezak , M. S. and Wilson , M. C. 2007a . Evaluation of Filter Sock Surface Sampling Collection Method for Bacillus Spores from Porous and Non-Porous Surfaces . J. Environ. Monit. , 9 ( 7 ) : 666 – 671 .
- Brown , G. S. , Betty , R. G. , Brockmann , J. E. , Lucero , D. A. , Souza , D. A. , Walsh , K. S. , Boucher , R. M. , Tezak , M. S. , Wilson , M. C. and Rudolph , T. 2007b . Evaluation of a Wipe Surface Sample Method for Collection of Bacillus Spores from Nonporous Surfaces . Appl. Environ. Microbiol. , 73 ( 3 ) : 706 – 710 .
- Brown , G. S. , Betty , R. G. , Brockmann , J. E. , Lucero , D. A. , Souza , D. A. , Walsh , K. S. , Boucher , R. M. , Tezak , M. S. , Wison , M. C. , Rudolph , T. , Lindquist , H. D. A. and Martinez , K. F. 2007c . Evaluation of Rayon Swab Surface Sample Collection Method for Bacillus Spores from Nonporous Surfaces . J. Appl. Microbiol. , 103 ( 4 ) : 1074 – 1080 .
- Burke , S. A. , Wright , J. D. , Robinson , M. K. , Bronk , B. V. and Warren , R. L. 2004 . Detection of Molecular Diversity in Bacillus atrophaeus by Amplified Fragment Length Polymorphism Analysis . Appl. Environ. Microbiol. , 70 ( 5 ) : 2786 – 2790 .
- Buttner , M. P. , Cruz , P. , Stetzenbach , L. D. , Klima-Comba , A. K. , Stevens , V. L. and Emanuel , P. A. 2004 . Evaluation of the Biological Sampling Kit (BiSKit) for Large-Area Surface Sampling . Appl. Environ. Microbiol. , 70 ( 12 ) : 7040 – 7045 .
- Buttner , M. P. and Stetzenbach , L. D. 1993 . Monitoring Fungal Spores in an Experimental Indoor Environment to Evaluate Sampling Methods and the Effects of Human Activity on Air Sampling . Appl. Environ. Microbiol. , 59 : 219 – 226 .
- Byrne , M. A. , Goddard , A. J. H. , Lange , C. and Roed , J. 1995 . Stable Tracer Aerosol Deposition Measurements in a Test Chamber . J. Aerosol Sci. , 26 ( 4 ) : 645 – 654 .
- Dull , P. , Wilson , K. E. , Kournikakis , B. , Whitney , E. A. S. , Boulet , C. A. , Ho , J. Y. , Ogston , J. W. , Spence , M. R. , MacKenzie , M. M. , Phelan , M. A. , Popovic , T. and Ashford , D. 2004 . Bacillus anthracis Aerosolization Associated with a Contaminated Mail Sorting Machine . Emerg. Infect. Dis. , 8 ( 10 ) : 1044 – 47 .
- Hinds , W. C. 1999 . Aerosol Technology , New York : J. Wiley and Sons .
- Hodges , L. R. , Rose , L. J. , Peterson , A. , Noble-Wang , J. and Arduino , M. J. 2006 . Evaluation of Macrofoam Swab Protocol for the Recovery of Bacillus anthracis Spores from a Steel Surface . Appl. Environ. Microbiol. , 72 ( 6 ) : 4429 – 4430 .
- Kennedy , E. R. , Fischbach , T. J. , Song , R. , Eller , P. M. and Shulman , S. A. 1995 . Guidelines for Air Sampling and Analytical Method Development and Evaluation, DHHS (NIOSH) Publ. No. 95–117
- Kirschner , L. E. and Puleo , J. R. 1979 . Wipe-Rinse Technique for Quantitating Microbial Contamination on Large Surfaces . Appl. Microbiol. , 38 ( 3 ) : 466 – 470 .
- Lai , A. C. K. and Nazaroff , W. W. 2000 . Modeling Indoor Particle Deposition from Turbulent Flow Onto Smooth Surfaces . J. Aerosol Sci. , 31 ( 4 ) : 473 – 476 .
- Qian , Y. , Willeke , K. , Ulevicius , V. and Grinshpun , S. A. 1997 . Particle Reentrainment from Fibrous Filters . Aerosol Sci. Technol. , 27 ( 3 ) : 394 – 404 .
- Rose , L. J. 2006 . Private communication (email April 7), Centers for Disease Control 1 and Prevention, Atlanta
- Rose , L. J. , Jensen , B. , Petersen , A. , Banerjee , S. N. and Arduino , M. J. 2004 . Swab Materials and Bacillus anthracis Spore Recovery from Nonporous Surfaces . Emerg. Infect. Dis. , 10 ( 6 ) : 1023 – 1029 .
- Sanderson , W. T. , Stoddard , R. R. , Echt , A. S. , Piacitelli , C. A. , Kim , D. , Horan , J. , Davies , M. M. , McCleery , R. E. , Muller , P. , Schnorr , T. M. , Ward , E. M. and Hales , T. R. 2004 . Bacillus anthracis Contamination and Inhalational Anthrax in a Mail Processing and Distribution Center . J. Appl. Microbiol. , 94 : 1048 – 1056 .
- Teshale , E. H. , Painter , J. , Burr , G. A. , Mead , P. , Wright , S. V. , Cseh , L. F. , Zabrocki , R. , Collins , R. , Kelley , K. A. , Hadler , J. L. and Swerdlow , D. L. 2002 . Environmental Sampling for Spores of Bacillus anthracis . Emerg. Infect. Dis. , 8 ( 10 ) : 1083 – 1087 .
- Thatcher , T. L. , Lai , A. C. K. , Moreno-Jackson , R. , Sextro , R. G. and Nazaroff , W. W. 2002 . Effects of Room Furnishings and Air Speed on Particle Deposition Rates Indoors . Atm. Environ. , 36 : 1811 – 1819 .
- Turner , J. R. and Hering , S. V. 1987 . Greased and Oiled Substrates as Bounce-Free Impaction Surfaces . J. Aerosol Sci. , 18 ( 3 ) : 215 – 224 .
- Weis , C. P. , Intrepido , A. J. , Miller , A. K. , Cowin , P. G. , Durno , M. A. , Gebhardt , J. S. and Bull , R. 2002 . Secondary Aerosolization of Viable Bacillus anthracis Spores in a Contaminated US Senate Office . JAMA , 288 ( 22 ) : 2853 – 2858 .