Abstract
The detailed chemical composition of particulate matter emissions from four non-smoking gasoline powered motor vehicles were measured using three different driving conditions: a cold-cold start Unified Driving Cycle (UDC), a hot UDC, and a steady state cruise driving cycle. The cold-cold start UDC tests were performed with a cold-cold start temperature of 0°C, which is significantly lower than the 24°C cold start temperature widely used for motor vehicle testing. Each vehicle was operated over three cold-cold UDC cycles, three hot UDC cycles, and a steady state driving cycle comprised of 2 hours at 100 kilometers per hour (kph) plus 1 hour at 50 kph. Particulate matter emissions were characterized for elemental carbon (EC), organic carbon (OC), sulfate ions, nitrate ions, ammonium ions, and organic compounds using gas chromatography mass spectrometry (GCMS). Mass emissions rates for the test vehicles using both the hot UDC and steady state driving cycles ranged from < 0.1 to 1.3 mg km −1 , while the average cold-cold UDC cycle emissions ranged from 1.0 to 7.1 mg km −1 for the four vehicles. The cold-cold start UDC emissions averaged 5–30 times higher than the hot start UDC emissions. EC was an important contributor to the particulate matter emissions for the cold-cold start UDC emissions. Speciation of the organic compounds in the particulate matter emissions demonstrates differences in the composition of the organic aerosol emissions for the different driving cycles. The results of the present study demonstrate the important impact of cold-cold start temperature and driving conditions.
INTRODUCTION
Efforts to properly assess the impact of motor vehicles on atmospheric particulate matter concentrations require an accurate representation of the mass emissions and chemical compositions of vehicular emissions in the study domain. Due to the complex distribution of particulate matter emissions among vehicles, and the sensitivity of each vehicle's emissions on driving cycle, cold start temperature, and fuel composition have largely not been examined. Efforts to measure the chemical composition of particulate matter emissions from motor vehicles have largely relied on the measurement of typical vehicles operating over a standardized driving cycle (CitationHildemann et al. 1991; CitationCadle et al. 1997, Citation1999; CitationSchauer et al. 1999, Citation2002). These “representative” mass emissions rates and chemical compositions have been used in air quality models without adjustment for specific vehicle activity patterns (i.e., actual driving cycles and the actual distribution of cold starts), or for actual vehicle cold start temperatures (CitationSchauer et al. 1996, Citation2002; CitationLurmann et al. 1997; CitationKleeman and Cass 1998; CitationSchauer and Cass 2000; CitationZheng et al. 2002; CitationFraser et al. 2003). However, as efforts are being made to develop models that more accurately quantify the effect of motor vehicles on air quality and human health in regions with diverse vehicle driving cycles and cold start temperatures, there is a need to develop location specific source profiles for particulate matter emissions from mobile sources. This article demonstrates the significant impact driving conditions and cold start temperature have on the detailed chemical composition of particulate matter emitted from four in-use gasoline-powered motor vehicles. Although a small number of past studies have addressed differences in particulate matter emissions of vehicles as a function of driving cycle and temperature (CitationCadle et al. 1997, Citation1999; CitationMoosmuller et al. 2001), these efforts have not measured detailed chemical changes in particulate matter composition as a function of operating condition for specific vehicles. This study provides details on the important changes in emissions as a function of driving cycle that demonstrate the variables that need to be included in future vehicles emissions testing programs that seek to support air quality studies. Likewise, this study helps provide the regulatory and health effects communities with an understanding of the importance of driving condition and cold start temperature on the composition of particulate matter emissions from motor vehicles.
METHODS
Vehicle Testing
Four in-use gasoline-powered motor vehicles were recruited in Minnesota and Michigan and transported to Ann Arbor, Michigan for testing. These vehicles were tested with commercial gasoline as received, without any vehicle maintenance or repair. Emissions tests were conducted on a chassis dynamometer equipped with a single stage constant volume dilution sampler (CVS) that operated at a constant diluted exhaust flowrate of 17.0 m3 min−1. The dilution air cleaning system on the CVS was comprised of only a furnace-type particle filter, and did not contain an absorption bed to remove semi-volatile and gaseous pollutants from the dilution air. The dilution air temperature was held between 48 and 52°C for all tests. presents the test matrix and information on the vehicles that were tested. The four vehicles ranged in model year from 1995 through 1999 and had vehicle mileages of 30,000 to 140,000 miles. The tailpipe particulate matter emissions from each vehicle were collected for chemical analysis using 5 dynamometer tests, which included three duplicate cold-cold UDC tests (CitationHo and Winer 1998), one composite test of three hot UDCs, and a steady state driving cycle test comprised of two hours at a nominal speed of 100 kilometers per hour followed by one hour at 50 kilometers per hour. In addition, five dynamic blank tests were inter-dispersed among the vehicle tests. These dynamic blank tests were performed by operating the dilution tunnel and sampling equipment in the same manner as with the vehicle tests except that the sample port, which was normally connected to the vehicle tailpipe, was plugged prohibiting vehicle emissions from being introduced into the CVS. In addition, a substrate loading blank test was also included as part of the test matrix. Before each cold-cold UDC cycle, the test cell was cooled until an engine oil temperature of 0°C was achieved. The vehicles were warmed up on the dynamometer prior to the hot UDC and steady state emissions tests and the dynamometer temperature was held at a nominal temperature of 25°C during the hot UDC tests. All four vehicles were tested using the same sequence of test cycles that interdispersed hot UDC cycles and steady state cycle among the cold-cold UDC tests. This was done to address biases that might be caused by lack of vehicle conditioning prior to testing on the dynamometer.
TABLE 1 Vehicle test matrix
Sample Collection
The dilution air system and diluted exhaust sampling equipment, which were located outside of the test chamber, were operated at an average temperature of 28°C for all of the tests. Diluted exhaust emissions from the CVS were collected for chemical analysis using three different samplers: a low volume sampler, a high volume sampler, and a nano-microorifice uniform deposit impactor (nano-MOUDI). The low volume and high volume samplers were constructed by the University of Wisconsin—Madison and consisted of commercially available sampler components. The low volume sampler incorporated three sampling legs (legs A through C) operating at 24 lpm each, with a combined flowrate of 72 lpm. Each sampling leg was configured with an Air & Industrial Hygiene Laboratory (AIHL) cyclone (Thermo-Anderson, Smyrna, GA) operated to achieve a PM2.5 sample cut-point, and followed by a flow splitter and two 47-mm filter holders. The flowrates through each filter holder were controlled at 12 lpm using critical flow orifices. Low volume sampler leg A contained two 47-mm Teflon membrane filters (Teflo, 2.0 μ m pore size, Pall-Gelman Life Sciences) that were weighed before and after sampling using a microbalance in a temperature and relative humidity controlled weighing room. These two filters were used to obtain duplicate fine particle (PM2.5) mass measurements. After the mass measurement was completed, one of the Teflon filters from leg A was analyzed for sulfate, nitrate and ammonium ions. Leg B was equipped with two 47 mm quartz fiber filters (Tissue quartz QAO, Pall-Gelman Life Sciences) that were pre-baked at 550°C before sample collection. One of these filters was used for OC and EC analyses. Downstream of one quartz fiber filter in leg B was a single polyurethane foam (PUF) plug (Badger Foam; 5.7 cm diameter by 7.6 cm long) that was pre-cleaned with organic solvents and used to collect semi-volatile organic compounds. Leg C of the low volume sampler contained two Teflon membrane filters (Teflo, 2.0 μ m pore size, Pall Life Sciences) that were pre-cleaned with dilute acid, rinsed with purified water and air dried in a metals clean room before sample collection. Pre-cleaned Teflon filters were handled with gloved-hands and plastic forceps to minimize contamination. One of the pre-cleaned Teflon filters was designated for trace metals analyses, results that are not reported in this article.
The high volume sampler consisted of a high volume PM2.5 cyclone (URG, Chapel Hill, NC) followed by a single 90-mm filter holder operating at 92 lpm. The flowrate of the high volume sampler was controlled by a bank of critical orifices that operated in parallel to achieve a total flowrate of 92 lpm. The high volume sampler was operated with a 90-mm quartz fiber filter (Tissue quartz QAO, Pall-Gelman Life Sciences) that was pre-baked at 550°C and was used for the analysis of particle phase organic compounds using GCMS techniques. Flowrates through the high volume and low volume samplers were measured before and after each test with rotometers that were previously calibrated inline with a dry gas meter and an electronic bubble flow meter, respectively.
Daily integrated samples of the CVS dilution air were also collected using a second high volume sampler and a single leg version of the low volume sampler. The dilution air low volume sampler was equipped with one pre-weighed 47-mm Teflon membrane filter for mass, sulfate, nitrate and ammonium ion measurement, and one pre-cleaned 47-mm Teflon membrane filter for metals analysis. The high volume sampler was operated with a 90-mm quartz fiber filter and was used for OC and EC measurements, and organic speciation analysis.
A single nano-MOUDI was used to collect size-resolved particulate matter samples of the diluted exhaust for chemical analysis and mass measurements. Five micro-orifice uniform deposit impactor (MOUDI) experiments were performed (Test 4, Test 10, Test 13, Test 16, and Test 23) in which impactor samples were collected using pre-baked aluminum foil substrates that were not coated with grease. Three additional MOUDI experiments (Tests 5 plus 7, Tests 17 plus 19, and Test 26) were collected using pre-cleaned and pre-weighed Teflon membrane substrates, which were also used uncoated.
Chemical Analysis
Teflon membrane filters for mass measurement were weighed using a microbalance (Metler MX-5) in a relative humidity and temperature controlled weighing room before and after sampling. Prior to weighing, each filter was equilibrated at 21 ± 2°C and 38 ± 3% relative humidity for 24 hours. Filters were re-weighed at least three times before and after sampling. Uncertainties in the measurement of the particle mass on the filters were determined from the standard deviation of the triplicate weighing for the samples and associated blanks.
Samples collected on Teflon membrane filters for analysis of sulfate, nitrate and ammonium ions were wetted with 300 μ l of ethanol and extracted into 30 ml of high purity water. The extracts were analyzed by ion chromatography (IC) using a modified version of NIOSH (National Institute for Occupational Safety and Health) Method 7903 and OSHA (Occupational Safety and Health Administration) Method 188. Laboratory blanks and spikes were included with each batch of samples. Uncertainties for the IC analysis have been estimated from historical records of duplicate analyses and spike samples, and have been approximated as 5% of the measured concentration with a threshold uncertainty equal to the standard deviation of the blanks.
The OC and EC present in the particulate matter samples were measured using the protocols standardized for the ACE-Asia intercomparison study (CitationSchauer et al. 2003), which is a modified version of the NIOSH 5040 method that uses a Sunset Laboratory Inc. (Forest Grove, OR) laboratory-based instrument. Uncertainties were calculated in accordance with the software provided by Sunset Laboratory Inc. that defines measurement uncertainty as 5% of the measured values with a threshold uncertainty of 0.20 μ g per square centimeter of filter deposit for both OC and EC.
Particle-phase organic compounds collected on the quartz fiber filters and gas-phase semi-volatile organic compounds collected on the PUF cartridges were analyzed using an updated version of the extraction and GCMS analysis reported by CitationSchauer et al. (Schauer et al. 1999, Citation2002). Briefly, the substrates used for organics analysis were spiked with a mixture of twelve deuterated compounds (dodecane-d 26, hexadecane-d 34, eicosane-d 42, octacosane-d 58, hexatriacontane-d 74, decanoic acid-d 19, heptadecanoic acid-d 33, phthalic acid-3,4,5,6-d 4, benzaldehyde-d 6, 4,4′dimethoxybenzophenone-d 8, acenaphthene-d 10, chrysene-d 12, dibenz[ah]anthracene-d 14, cholesterol-2,2,3,4,4,6-d 6, α α α -20R-cholestane-d 4) and one carbon-13 labeled compound (levoglucosan-13C6). The samples were then extracted in a soxhlet using methylene chloride for 24 hours, followed by methanol for 24 hours. The extracts were combined and then reduced in volume to about 5 ml using a rotary vacuum evaporator. The extracts were concentrated to 250 μ l and were then derivatized with diazomethane to convert the organic acids to their methyl ester analogues. The extracts were analyzed using an Agilent HP5973 GCMS equipped with a HP-5MS Gas Chromatography (GC) column (30 meters length, 0.25 mm diameter, 0.25 μ m film thickness). Before and after each batch of samples was analyzed using the GCMS, multipoint calibration curves were generated using authentic standards spiked with the same final concentrations of internal standards as used in the samples. The quantification standards included polycyclic aromatic hydrocarbons (PAH), substituted PAH, n-alkanes, hopanes, steranes, n-alkanoic acids, and diacids. All compounds that were not present in the quantification standards were identified using qualitative standards and/or secondary standards and were quantified using response factors of compounds with similar chemical structure and molecular weights. As part of the quality control and quality assurance program for this study, spiked filters were analyzed to determine the overall efficiency and accuracy of the measurements. Average recoveries (average ± standard deviation) for the spiked samples were 93% ± 18% for PAHs, 94% ± 7% for the hopanes and steranes, 98% ± 10% for n-alkanes, and 93% ± 4% for the n-alkanoic acids.
Duplicate mass measurements, ions, EC, OC, and trace metals were analyzed for each vehicle tested, the dynamic blanks, and the loading blank. Due to analytical detection limits and cost considerations, organic speciation of the particulate matter and the semi-volatile organic compounds was performed on composite samples from the different tests, including a composite of the dynamic blank tests. In addition, dilution air blanks from each test were analyzed for EC, OC, and ions, along with one composite GCMS analysis of dilution air samples.
MOUDI substrates that were analyzed for mass, and ions were analyzed using the same protocols as used for the PM2.5 samples. The EC and OC analyses for the MOUDI substrates were modified to account for the fact that the laser used for pyrolysis correction by the ECOC instrument does not penetrate the MOUDI foils. To this end, the split time between EC and OC for the MOUDI substrates was estimated to be the same as in the analysis of the parallel PM2.5 EC and OC samples (CitationKleeman et al. 1999, Citation2000). MOUDI samples from a cold UDC test of Vehicle 2 (Test 13) and the steady state test of Vehicle 3 (Test 16) were analyzed for OC, EC, sulfate, nitrate, and ammonium ions. In addition, one set of MOUDI samples from a composite of two cold-cold UDC tests of Vehicle 1 (Test 5 and Test 7) was analyzed for trace metals.
RESULTS
Blank Correction
The ability to accurately measure low level emissions requires accurate correction for the background concentrations present in the dilution tunnel. The dilution tunnel background concentrations arise both from contaminants passing through the dilution air particle filter and the “emissions” of contaminants from the dilution sampler surfaces. The concentrations (average ± standard deviation) in the CVS system during the dynamic blank tests were 12.6 ± 4.0 μ g m−3 for fine particle mass, 7.6 ± 1.9 μ g m−3 for fine particle OC, 1.8 ± 1.1 μ g m−3 for fine particle EC, 1.7 ± 1.3 μ g m−3 for fine particle nitrate ion, 2.7 ± 2.3 μ g m−3 for fine particle sulfate ion, and 1.6 ± 1.0 μ g m−3 for fine particle ammonium ion. In all cases, the daily average concentrations of these species in the dilution air were less than or equal to the dynamic blank concentrations. All of the vehicle emissions tests were blank corrected using the average concentration of the chemical species present in all dynamic blank tests. The variability in the dynamic blank tests was used in an error propagation calculation for the emissions rate of these species. A similar result was observed for the particle-phase organic compounds, and the same blank correction approach was employed. The concentrations of gas-phase, semi-volatile organic compounds were not measured in the dilution air; however, it was observed that the concentrations of these compounds during the emissions tests were very similar to the composite dynamic blank tests. The emissions of semi-volatile organic compounds were not quantified, since “emissions” from the CVS surfaces and/or the concentration of these species in the dilution air were a significant contributor to the concentration of these species in the CVS during the vehicle emissions tests. As a result, the blank corrected semi-volatile organic compound emissions had very high relative errors and could not be accurately quantified. The uncertainty for the analysis of the particle-phase organic compounds was estimated to be ± 20%, based on the work by CitationSheesley et al. (2003) and CitationManchester-Neesvig et al. (2003). Uncertainties in the emissions rates of particle-phase organic compounds were determined using an error propagation analysis of the blanks and diluted exhaust emissions samples.
Emissions Rates and Bulk Chemical Composition
and present the blank corrected mass emissions rates of the major chemical species for the study vehicles operating over the different driving cycles. The three duplicate cold-cold UDC tests of each vehicle show good repeatability in terms of mass emissions rate and chemical composition (i.e., uncertainties of the triplicate mass and EC emissions rates for all for vehicles was less than 1 mg km−1). It is important to recognize that the measurement of organic carbon (OC) does not include hydrogen, oxygen, nitrogen, and sulfur, and a factor is needed to convert OC to organic compound mass (CitationTurpin and Lim 2001). Lubricating oil and fuel samples from the four test vehicles were analyzed for bulk composition, and the lubricating oil samples were found to contain between 83.9% and 85.0% carbon by mass. The fuel samples were found to contain between 83.7% and 88.2% carbon by mass. Thus, the organic fraction of particulate matter derived from unburned fuel and unburned lubricating oil would have an organic mass to OC ratio in the range of 1.13 to 1.19. CitationTurpin and Lim (2001) recommended the use of 1.6 as an organic mass to OC ratio for urban aerosols, but do not make a recommendation for primary emissions from motor vehicles. As shown in , the use of 1.2 for a ratio of organic compound mass to OC does not provide good closure on the mass balance for Vehicles 1 and 2, but does provide reasonable mass closure for Vehicles 3 and 4. The missing mass cannot be attributed to trace metals since these are only small contributors to the mass, as discussed later in this manuscript, and is not expected to be due to negative OC sampling artifacts (CitationMader et al. 2003). It is likely that the OC fraction, and possibly the EC fraction, contains a significant component of oxygen, nitrogen, or sulfur. It is interesting to note that the lubricating oils contained 2.2 to 3.5 wt% oxygen and 0.30 to 0.39 wt% sulfur, and the gasoline fuels contained 1.6 to 4.0 wt% oxygen and < 0.02 to 0.07 wt% sulfur. The lower EC emissions of the cold-cold start tests for Vehicle 3, the Hot UDC tests and the steady state tests, along with their better mass closure, suggest that the additional elements are associated with the EC. In this context, it is important to recognize that the EC measurement is operationally defined as refractory carbon and does not imply that it is pure carbon (CitationSchauer et al. 2003).
FIG. 1 Emissions of PM2.5 chemical species from four gasoline-powered motor vehicles operating over different driving cycles.
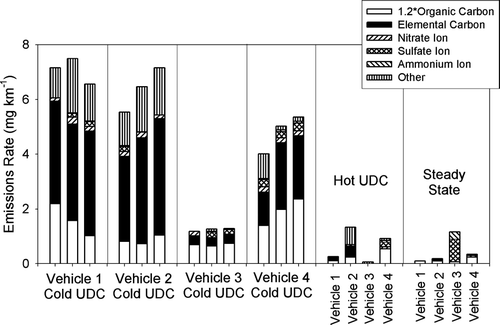
TABLE 2 Emissions rates of PM2.5 mass, OC, EC, sulfate ion, nitrate ion, and ammonium ion
As shown in and , carbonaceous particulate matter is the dominant contributor to the particulate matter emissions under cold-cold start conditions for all four vehicles. The particulate matter emissions from the cold-cold UDC tests of Vehicle 4 contain a small but statistically significant amount of sulfate, whereas the sulfate ion from the cold-cold UDC tests of the other vehicles is not statistically significant. Emissions of nitrate ions were statistically significant for Vehicles 1, 2, and 4, but contributed no more than 5% of the particle mass emissions. The composition of the particulate matter emitted during the hot UDC tests and the steady state tests was drastically different from the cold-cold start tests. The mass emissions, as well as the emissions of EC, OC, and ions, were not statically different from the dynamic blanks for the hot UDC tests of Vehicles 1 and 3 (see ). The mass emissions rate and emissions rate of carbonaceous particulate matter were above the dynamic blank levels for the hot UDC tests of Vehicles 2 and 4, with mass emissions rates around 1.0 mg km− 1. EC was a major contributor to the particulate matter emissions of Vehicle 1, while OC was a major contributor to the emissions of Vehicle 3. In all cases, however, the emissions of particle mass and carbonaceous aerosols were significantly smaller for the hot UDC tests than for the cold UDC tests. These results are consistent the impact of cold start and ambient temperature reported by CitationZielinska et al. (2004).
Other than Vehicle 3, the emissions from the steady state driving cycle were even lower than from the hot UDC cycle. Vehicle 3 had significant emissions of sulfate ion during the steady state test, which accounted for 73% of the particle mass emissions rate. Assuming a vehicle gasoline consumption of 8 kilometers per liter of fuel and a fuel density of 0.7 grams cm−3, this yields a sulfur emissions rate of 4.8 mg of sulfur per kilogram of fuel. The sulfur content of the fuel was 700 mg per kilogram of fuel (i.e., 0.07 wt% S) and the sulfur content of this vehicles lube oil was 3900 mg per kilogram of lube oil (0.39 wt% S). To this end, the oxidation of a small fraction of the sulfur in the fuel of lubricating oil within the engine, or the vehicle after-treatment system, yielded a significant increase in the mass of particulate matter emissions. It is unclear if the ammonium emissions resulted from the emissions of ammonia or ammonium ions from the vehicle, or from the reaction of the emitted sulfate ion with ammonia gas that was present in the dilution air.
Size Resolved Chemical Composition of Particulate Matter Emissions
shows six of the mass size distributions measured in the CVS using the nano-MOUDI. The results shown in are the actual mass size distributions measured in the CVS and have been loading blank corrected but not dynamic blank corrected. As shown in , the particle mass concentrations were high enough during only the cold-cold start UDC tests and the steady state test of Vehicle 3 to accurately measure the size distributions. It is interesting to note that the peak in the mass size distribution for the cold-cold start tests was at an approximate particle diameter of 0.14 μ m, and the peak in the distribution for the high sulfate emissions tests was 0.02 μ m, as shown in and . Although impactors are subject to sampling biases (CitationLawson 1980; CitationChan and Lawson 1981), the mass size distributions measured by these impactor samples were consistent with co-located particle size distribution measurements during the emissions tests using a differential mobility analyzer coupled with a condensation nuclei counter (DMA/CNC) (CitationKittelson et al. 2003).
FIG. 2 Particle mass size distributions of diluted vehicle exhaust emissions—measured with a nano-MOUDI impactor.
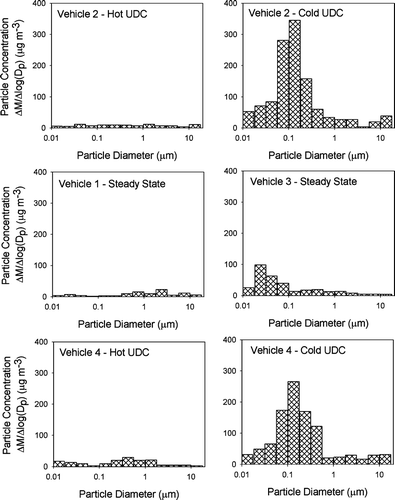
presents the EC and mass size distributions for the nano-MOUDI samples collected during the cold-cold UDC test of Vehicle 2. OC and the measured water-soluble ions were not above the nano-MOUDI loading blanks and are not shown in . The fine particle EC and mass concentrations measured by the nano-MOUDI for the cold-cold start UDC test of Vehicle 2 were not statistically different from the co-located PM2.5 EC measurements. presents the parallel size resolved chemical composition of the particulate matter emissions from the Vehicle 3 operating over the steady state cycle. The total fine particle sulfate measured by the nano-MOUDI impactor for this test was not statistically different from the fine particle sulfate measured with the co-locate PM2.5 sample. The source of the high nitrate ion mass on the large particle stage of the nano-MOUDI collected during this test, as shown in , is not known but a possible explanation is an adsorption artifact of gas-phase nitric acid on the first stage of nano-MOUDI.
Emissions of Particle-Phase Organic Compounds
In order to obtain adequate organic aerosol mass for organic speciation, samples from compatible tests were composited for organic speciation analysis. The triplicate cold-cold UDC tests for each vehicle were composited into a single cold-cold UDC analysis for each vehicle. The hot UDC samples from Vehicles 1 and 2 were composited into one sample: those from Vehicles 3 and 4 were also composited into another. Likewise, the samples from the steady state tests of Vehicles 1 and 2 were composited, while those of the steady state tests of Vehicles 3 and 4 were analyzed as individual samples. In addition, one composite dynamic blank sample and one composite dilution air sample were analyzed by GCMS. As previously stated, the vehicle emissions samples were blank corrected using the composite dynamic blank sample. Using the approximated 20% uncertainty for the dynamic blanks and emissions samples, the concentrations of organic compounds in the CVS during the vehicle emissions tests that were not statistically different from the dynamic blanks were defined as below detection. provides the emissions rates of the measured organic compounds.
TABLE 3 Emissions rates of particle phase organic compounds
presents the emissions rates of four high-molecular weight PAHs, whose concentrations in the urban atmosphere are greatly impacted by gasoline powered motor vehicles (CitationSchauer et al. 1996). The emissions of these PAHs clearly increase under cold-cold start conditions. The difference between the emissions during the cold-cold start UDC cycle and the hot UDC cycle has been termed the incremental cold start. For the 4 vehicles tested, the emissions of these PAHs are dominated by the incremental cold start. In contrast to the PAH, shows relatively constant emissions rates of hopanes and steranes, which are components of motor vehicle lubricating oil (CitationSimoneit 1985, Citation1986, Citation1999). An interesting result is that emission rates of the compounds seem to have a consistent average across the different driving cycles for all of the vehicles tested. CitationZielinska et al. (2004) observed that PAH, hopanes, and steranes all increased with decreasing cold start temperature. Although the current results show agreement for PAH, the current results suggest that the trends in hopane and sterane emission rates are highly dependent on the specific vehicles and their representation of the true on-road fleet.
FIG. 5 Emissions of selected PM2.5 high molecular weight PAH compounds from four gasoline-powered vehicles operating over different driving cycles. Values shown as zero were below detection.
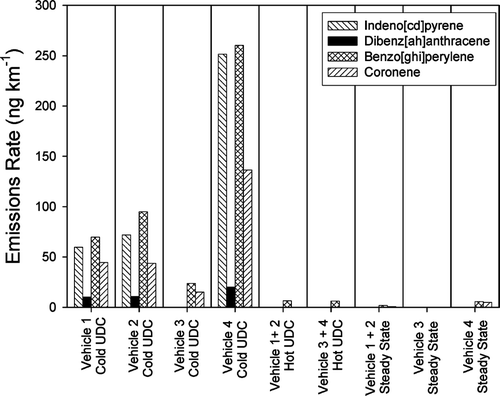
FIG. 6 Emissions of selected PM2.5 Hopane and Sterane compounds from four gasoline-powered vehicles operating over different driving cycles. Values shown as zero were below detection.
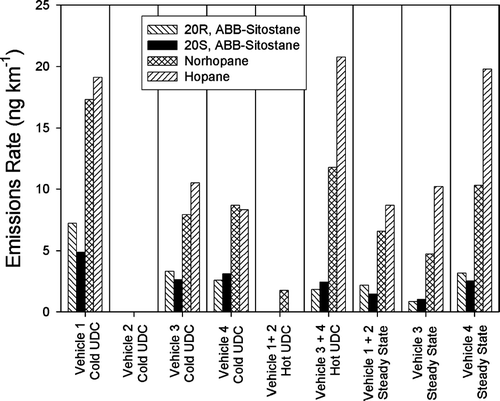
The emissions of high molecular weight n-alkanes in particulate matter from gasoline-powered motor vehicles are not fully understood. Previous measurements have demonstrated much higher emissions of these compounds than would be predicted from the concentrations of these compounds in lubricating oils and gasoline (CitationSchauer et al. 2002). There is reason to believe that these compounds are formed in the engine. shows the emissions rates of four of these n-alkanes. It is observed that the emissions of these compounds are higher for the steady state and hot UDC cycle than for the cold-cold UDC cycle. In addition, the composite of Vehicles 1 and 2 for the hot UDC cycle and the steady state cycle are significantly higher that the emissions observed from the other vehicles. Further investigations are warranted to understand the potential mechanism of n-alkane formation in the engine.
DISCUSSION
Although the current study is based on only a limited of motor vehicles, the results demonstrate that cold start temperature has a significant impact on the emission rate and composition of particulate matter form gasoline-powered spark ignition vehicles. Both emission rates and the elemental carbon fraction of the particulate matter both increased significantly with decreasing ambient temperature for all tested vehicles. A similar tend was observed for PAH but not hopanes, steranes, and particle-phase n-alkanes.
Although significant changes were also observed in the particulate matter emissions and particulate matter composition for the subject vehicles as a function of driving cycle, the trends were not consistent across vehicles. As a result, the study cannot be used to draw conclusions about the expected trends in PM emissions rate and composition as a function of driving cycle for the average on-road fleet. However, the study suggests that changes due to different driving cycles may not be as dramatic as the impact of cold start temperature.
The results of the study demonstrate the need to develop low temperature source profiles for winter time source apportionment studies in locations where winter temperatures are frequently in the range of, or below, 0°C. The expected changes in the EC and PAH contents of the emissions are important characteristics often exploited in the splitting emissions from gasoline and diesel engines.
This work has been supported by Dr. James Eberhardt of the U.S. Department of Energy's Offices of Heavy Vehicle Technologies and FreedomCAR Vehicle Technologies. Dr. Doug Lawson (NREL) was the project manager for the study.
Notes
*Assumes Ratio of Organic Mass to OC = 1.2.
REFERENCES
- Cadle , S. H. , Mulawa , P. A. , Ball , J. , Donase , C. , Weibel , A. , Sagebiel , J. C. , Knapp , K. T. and Snow , R. 1997 . Particulate Emission Rates from In Use High Emitting Vehicles Recruited in Orange County, California . Environ. Sci. Technol. , 31 ( 12 ) : 3405 – 3412 .
- Cadle , S. H. , Mulawa , P. A. , Hunsanger , E. C. , Nelson , K. , Ragazzi , R. A. , Barrett , R. , Gallagher , G. L. , Lawson , D. R. , Knapp , K. T. and Snow , R. 1999 . Composition of Light–Duty Motor Vehicle Exhaust Particulate Matter in the Denver, Colorado Area . Environ. Sci. Technol. , 33 ( 14 ) : 2328 – 2339 .
- Chan , T. L. and Lawson , D. R. 1981 . Characteristics of Cascade Impactors in Size Determination of Diesel Particles . Atmos. Environ. , 15 : 1273 – 1279 .
- Fraser , M. P. , Yue , Z. W. and Buzcu , B. 2003 . Source Apportionment of Fine Particulate Matter in Houston, TX, Using Organic Molecular Markers . Atmos. Environ. , 37 ( 15 ) : 2117 – 2123 .
- Hildemann , L. M. , Markowski , G. R. and Cass , G. R. 1991 . Chemical-Composition of Emissions from Urban Sources of Fine Organic Aerosol . Environ. Sci. Technol. , 25 ( 4 ) : 744 – 759 .
- Ho , J. and Winer , A. M. 1998 . Effects of Fuel Type, Driving Cycle, and Emission Status on In-Use Vehicle Exhaust Reactivity . J. Air Waste Mange. Assoc. , 48 ( 7 ) : 592 – 603 .
- Kittelson , D. , Watts , W. , Johnson , J. , Zarlick , D. , Baltensperger , U. , Schauer , J. J. and Christenon , C. 2003 . Gasoline Vehicle Exhaust Particle Sampling Study—Final Report, University of Minnesota .
- Kleeman , M. J. and Cass , G. R. 1998 . Source Contributions to the Size and Somposition Distribution of Urban Particulate Air Pollution . Atmos. Environ. , 32 ( 16 ) : 2803 – 2816 .
- Kleeman , M. J. , Schauer , J. J. and Cass , G. R. 1999 . Size and Composition Distribution of Fine Particulate Matter Emitted from Wood Burning, Meat Charbroiling, and Cigarettes . Environ. Sci. Technol. , 33 ( 20 ) : 3516 – 3523 .
- Kleeman , M. J. , Schauer , J. J. and Cass , G. R. 2000 . Size and Composition Distribution of Fine Particulate Matter Emitted from Motor Vehicles . Environ. Sci. Technol. , 34 ( 7 ) : 1132 – 1142 .
- Lawson , D. R. 1980 . Impaction Surface Coating Intercomparison and Measurement with Cascade Impactors . Atmos. Environ. , 14 : 195 – 199 .
- Lurmann , F. W. , Wexler , A. S. , Pandis , S. N. , Musarra , S. , Kumar , N. and Seinfeld , J. H. 1997 . Modelling Urban and Regional Aerosols. 2. Application to California's South Coast Air Basin . Atmos. Environ. , 31 ( 17 ) : 2695 – 2715 .
- Mader , B. T. , Schauer , J. J. , Seinfeld , J. H. , Flagan , R. C. , Yu , J. Z. , Yang , H. , Lim , H. J. , Turpin , B. J. , Deminter , J. T. , Heidemann , G. , Bae , M. S. , Quinn , P. , Bate , T. , Eatough , D. J. , Huebert , B. J. , Bertram , T. and Howell , S. 2003 . Sampling Methods Used for the Collection of Particle-Phase Organic and Elemental Carbon During ACE-Asia . Atmos. Environ. , 37 ( 11 ) : 1435 – 1449 .
- Manchester-Neesvig , J. B. , Schauer , J. J. and Cass , G. R. 2003 . The Distribution of Particle–Phase Organic Compounds in the Atmosphere and Their Use for Source Apportionment during the Southern California Children's Health Study . J. Air Waste Mange. Assoc. , 53 In press
- Moosmuller , H. , Arnott , W. P. , Rogers , C. F. , Bowen , J. L. , Gillies , J. A. , Pierson , W. R. , Collins , J. F. , Durbin , T. D. and Norbeck , J. M. 2001 . Time-Resolved Characterization of Diesel Particulate Emissions. 2. Instruments for Elemental and Organic Carbon Measurements . Environ. Sci. Technol. , 35 ( 10 ) : 1935 – 1942 .
- Schauer , J. J. and Cass , G. R. 2000 . Source Apportionment of Wintertime Gas-Phase and Particle-Phase Air Pollutants Using Organic Compounds as Tracers . Environ. Sci. Technol. , 34 ( 9 ) : 1821 – 1832 .
- Schauer , J. J. , Kleeman , M. J. , Cass , G. R. and Simoneit , B. R. T. 1999 . Measurement of Emissions from Air Pollution Sources. 2. C-1 through C-30 Organic Compounds from Medium Duty Diesel Trucks . Environ. Sci. Technol. , 33 ( 10 ) : 1578 – 1587 .
- Schauer , J. J. , Kleeman , M. J. , Cass , G. R. and Simoneit , B. R. T. 2002 . Measurement of Emissions from Air Pollution Sources. 4. C-1-C-27 Organic Compounds from Cooking with Seed Oils . Environ. Sci. Technol. , 36 ( 4 ) : 567 – 575 .
- Schauer , J. J. , Kleeman , M. J. , Cass , G. R. and Simoneit , B. R. T. 2002 . Measurement of Emissions from Air Pollution Sources. 5. C-1-C-32 Organic Compounds from Gasoline-Powered Motor Vehicles . Environ. Sci. Technol. , 36 ( 6 ) : 1169 – 1180 .
- Schauer , J. J. , Mader , B. T. , Deminter , J. T. , Heidemann , G. , Bae , M. S. , Seinfeld , J. H. , Flagan , R. C. , Cary , R. A. , Smith , D. , Huebert , B. J. , Bertram , T. , Howell , S. , Kline , J. T. , Quinn , P. , Bates , T. , Turpin , B. , Lim , H. J. , Yu , J. Z. , Yang , H. and Keywood , M. D. 2003 . ACE–Asia Intercomparison of a Thermal-Optical Method for the Determination of Particle–Phase Organic and Elemental Carbon . Environ. Sci. Technol. , 37 ( 5 ) : 993 – 1001 .
- Schauer , J. J. , Rogge , W. F. , Hildemann , L. M. , Mazurek , M. A. and Cass , G. R. 1996 . Source Apportionment of Airborne Particulate Matter Using Organic Compounds as Tracers . Atmos. Environ. , 30 ( 22 ) : 3837 – 3855 .
- Sheesley , R. J. , Schauer , J. J. , Chowdhury , Z. , Cass , G. R. and Simoneit , B. R. T. 2003 . Characterization of Organic Aerosols Emitted from the Combustion of Biomass Indigenous to South Asia . J. Geophys. Res.—Atmospheres , 108 ( D9 ) art. no.-4285
- Simoneit , B. R. T. 1985 . Application of Molecular Marker Analysis to Vehicular Exhaust for Source Reconciliations . International Journal of Environmental Anal. Chem. , 22 ( 3–4 ) : 203 – 233 .
- Simoneit , B. R. T. 1986 . Characterization of Organic-Constituents in Aerosols in Relation to Their Origin and Transport—A Review . International Journal of Environmental Anal. Chem. , 23 ( 3 ) : 207 – 237 .
- Simoneit , B. R. T. 1999 . A Review of Biomarker Compounds as Source Indicators and Tracers for Air Pollution . Environmental Science and Pollution Research , 6 ( 3 ) : 159 – 169 .
- Turpin , B. J. and Lim , H. J. 2001 . Species Contributions to PM2.5 Mass Concentrations: Revisiting Common Assumptions for Estimating Organic Mass . Aerosol Sci. Technol. , 35 ( 1 ) : 602 – 610 .
- Zheng , M. , Cass , G. R. , Schauer , J. J. and Edgerton , E. S. 2002 . Source Apportionment of PM2.5 in the Southeastern United States Using Solvent-Extractable Organic Compounds as Tracers . Environ. Sci. Technol. , 36 ( 11 ) : 2361 – 2371 .
- Zielinska , B. , Sagebiel , J. , McDonald , J. D. , Whitney , K. and Lawson , D. R. 2004 . Emission Rates and Comparative Chemical Composition from Selected In-Use Diesel and Gasoline-Fueled Vehicles . Journal of the Air and Waste Management Association , 54 : 1138 – 1150 .