Abstract
We report on a new instrument developed to perform rapid, size-resolved aerosol hygroscopicity measurements. The differential aerosol sizing and hygroscopicity spectrometer probe (DASH-SP) employs differential mobility analysis in-concert with multiple humidification and optical sizing steps to determine dry optical size and hygroscopic growth factors for size-selected aerosols simultaneously at three elevated relative humidities. The DASH-SP has been designed especially for aircraft-based measurements, with time resolution as short as a few seconds. The minimum particle diameter detected with 50% efficiency in the optical particle counters (OPCs) is 135 ± 8 nm, while the maximum detectable particle diameter is in excess of 1 μm. An iterative data processing algorithm quantifies growth factors and “effective” refractive indices for humidified particles using an empirically derived three-dimensional surface (OPC pulse height–refractive index–particle size), based on a calculated value of the “effective” dry particle refractive index. Excellent agreement is obtained between DASH-SP laboratory data and thermodynamic model predictions for growth factor dependence on relative humidity for various inorganic salts. Growth factor data are also presented for several organic acids. Oxalic, malonic, glutaric, and glyoxylic acids grow gradually with increasing relative humidity up to 94%, while succinic and adipic acids show no growth. Airborne measurements of hygroscopic growth factors of ship exhaust aerosol during the 2007 Marine Stratus/Stratocumulus Experiment (MASE II) field campaign off the central coast of California are presented as the first report of the aircraft integration of the DASH-SP.
INTRODUCTION
Uptake of water by atmospheric aerosols is important in a variety of phenomena: light scattering, ability to act as cloud condensation nuclei, and deposition within the human respiratory system. Aerosol hygroscopicity measurements have been made with a variety of systems (). The parameter normally quantified is the diameter growth factor (GF = D p,wet /D p,dry ). Laboratory growth factor measurements are commonly performed by levitating a particle in an electrodynamic balance (CitationCohen et al. 1987a, Citation1987b; CitationTang and Munkelwitz 1993, Citation1994a, Citation1994b; CitationChan et al. 1997; CitationPeng et al. 2001; CitationPeng and Chan 2001). This method probes single, laboratory-generated particles, directly measuring the mass change in response to humidity variation. The need to directly measure the growth factor of ambient aerosols led to the development of the hygroscopicity tandem differential mobility analyzer (HTDMA) (CitationLiu et al. 1978; CitationSekigawa 1983; CitationRader and McMurry 1986), in which dry particles of a selected size are exposed to a specific relative humidity (RH), after which the wet size is measured by classifying the grown particles with a second DMA, and counting with a condensation particle counter (CPC). Owing to the long duration of time required to measure the size distribution of the grown particles using a DMA, the HTDMA technique is relatively slow. However, both techniques above enable high precision measurements of the growth factor.
TABLE 1 Aerosol hygroscopicity measurement techniques
While these methods have produced important insights into the hygroscopic behavior of atmospheric aerosols, the long time required to determine growth factors for different particle sizes and RHs makes them impractical in a number of important measurement scenarios in which the properties of sampled particles vary rapidly, especially during airborne measurements. A number of alternate methods, which are described in detail in , have been employed to probe the hygroscopic properties of the atmospheric aerosol more rapidly than is possible with the HTDMA. Several of these methods probe the entire aerosol as measured at different humidities, using nephelometers to probe ensemble light scattering (CitationRood et al. 1985; CitationDougle et al. 1998; CitationCarrico et al. 1998, Citation2000; CitationSheridan et al. 2002; CitationMagi and Hobbs 2003; CitationKim et al. 2006), or measuring aerosol size distributions with optical particle sizing instruments in parallel (CitationKotchenruther and Hobbs 1998; CitationHegg et al. 2006, Citation2007; CitationSnider and Petters 2007), or multiple DMAs operated in parallel (CitationWang et al. 2003). The use of an optical particle counter (OPC) in place of a DMA detector is not new, as several investigators (CitationCovert et al. 1990; CitationHering and McMurry 1991; CitationBrand et al. 1992) used OPC detection of mobility-classified particles to gain insight into the mixing state of atmospheric aerosol and to determine their optical properties. One approach that attempts to provide the resolution of the HTDMA without the response time limitations is to classify particles with a DMA operated at one RH, equilibrate the classified particles at a second RH, and then measure the resulting size distribution using a fast particle sizer such as an OPC. CitationKreisberg et al. (2001) classified particles at a high RH, dried the classified particles, and measured the dry particle size using an OPC in an instrument they called the relative humidity-moderated differential mobility optical particle size spectrometer (RH-DMOPSS). The RH-DMOPSS, which is an extension of the DMOPSS design of CitationStolzenburg et al. (1998), measures shrinkage by drying rather than growth upon humidification like most other hygroscopic growth instruments. The RH-DMOPSS is an improvement upon the HTDMA in that it is faster and can measure a larger range of particle sizes (0.1–1.1 μ m). However, this instrument provides a growth factor at only one RH at any given time.
In this article, we describe a new instrument that enables simultaneous determination of growth factors at several RHs, and that overcomes the challenges of determining the size of dry particles with unknown composition. The instrument has been designed especially for aircraft-based measurements. The differential aerosol sizing and hygroscopicity spectrometer probe (DASH-SP), developed by Brechtel Mfg. Inc. (http://www.brechtel.com/), employs DMA classification of dry aerosol particles, equilibrates the classified particles to a new RH, and then measures the sizes of the grown particles using an OPC. An iterative data processing algorithm quantifies growth factors and “effective” refractive indices (n) for wet particles based on a calculated “effective” dry particle refractive index; iterations are performed on a three-dimensional surface (pulse height – n – D p ) based on dry particle calibration data from several salts with known refractive indices. By operating four controlled-RH channels in parallel and equipping each of the humidified channels with a separate OPC, hygroscopic growth factors can rapidly be determined at a number of particle sizes without having to incur the time delays required to stabilize a humidification column to a new RH. Depending upon the concentration and size distribution of the aerosol sampled, the growth factors of particles at any selected size within the size resolution of the instrument can be determined in as little as a few seconds.
We present first an overview of the design of the DASH-SP instrument. Results from laboratory characterization tests illustrate the performance of the instrument, including size detection limits, time resolution, stability, accuracy, and inherent uncertainties. The data processing algorithm is subsequently described, which is used to convert pulse height data to growth factors. Hygroscopicity measurements for various inorganic salts and organic acids are compared to theoretical predictions for growth factor dependence on RH. Finally, airborne field measurements establish that the DASH-SP is capable of measuring growth factors at multiple sizes and RHs with a time resolution as much as two orders of magnitude shorter than that of the HTDMA.
DASH-SP DESCRIPTION
The DASH-SP, illustrated in , couples a single classification DMA system with a set of growth factor measurement systems that are operated in parallel. The modules that comprise the instrument are, in the order in which the aerosol experiences them: (1) an aerosol drier; (2) an aerosol neutralizer; (3) a classification DMA that selects the dry mobility size range for which growth factor measurements are made; (4) a set of parallel aerosol conditioning modules in which the aerosol is brought to equilibrium at controlled RHs; and (5) a detector at the outlet end that determines the total particle concentration leaving the DMA, and the size distributions of the particles after RH equilibration. The aerosol path through the instrument will now be described in detail.
A 0.5 LPM aerosol sample flow first passes through a Nafion drier (described below), and then passes through a 210Po neutralizer that brings the dried particles to a stable, steady-state charge distribution. The aerosol then enters a cylindrical DMA with a 33.9 cm long, classification column, and inner and outer radii of 62.4 mm, and 72.3 mm, respectively. The DMA sheath flow rate is 5 LPM. The DMA selects particles in a narrow interval of mobility-equivalent diameters in the 0.1–1.0 μ m range. The minimum voltage at which particles are classified is 281 V (to select 150 nm diameter particles), so Brownian diffusion does not significantly broaden the DMA transfer function (CitationFlagan 1999), and the transmission characteristics of the DMA closely approach the ideal, nondiffusion transfer function of Knutson and Whitby (1974). Particles larger than 1 μ m can be classified by operating the DMA at lower flowrates, although the fraction of particles that carry multiple charges will become significant, making data analysis more difficult. For the present purposes, we shall limit discussion to measurement of growth factors of particles with dry diameters of 1 μ m or smaller. The classified aerosol leaving the DMA is split into five separate flows. The total concentration of classified particles is determined, without further conditioning of one of the five flows, by measuring the dry, classified particles using an integral TSI Model 3781 water-based condensation particle counter (CPC). The CPC serves as a redundant particle number concentration measurement against which data from the OPCs, to be described below, can be compared.
The remaining four classified aerosol flows are used to measure growth factors. They first pass through a Perma Pure, LLP, Nafion humidifier (Model MD-070-24FS-4) that consists of a Nafion membrane tube that is enclosed in a coaxial tube. The Nafion efficiently transmits water through the membrane, enabling the small aerosol flow through the center tube to equilibrate with the RH of the larger flow between the membrane and the outer shell. The RH of that larger flow is adjusted by mixing of dry air with air that has been saturated with water vapor by passage through a Nafion tube coil that is immersed in a warm water bath. Feedback control using an RH sensor (Model HMP50YCC1A2X, Vaisala) ensures that a constant, predetermined RH is achieved in both the conditioned aerosol flow, and the outer flow. One humidifier is kept dry (< 8% RH), while the other three are operated at higher and different RHs. The maximum RH is typically below 94% in order to prevent condensation within the instrument, although higher RH can be used for short periods of time.
The aerosol growth factor is measured as a surrogate for particle growth in response to humidity changes in the atmosphere where sufficient time is generally available for the droplets to fully equilibrate with atmospheric water vapor. To simulate the atmospheric response effectively, the time in the conditioning columns must be sufficient to ensure full equilibration. The characteristic time for a droplet to reach thermodynamic equilibrium with water vapor in unsaturated conditions (RH < 100%) is estimated to be on the order of microseconds (CitationPilinis et al. 1989). The ∼4 s residence time within each conditioning column is sufficient to ensure equilibration. Moreover, as described below, the outer flow from the Nafion humidifier is used as a sheath flow within the OPC to ensure that the equilibrium is maintained to the point of measurement.
The four conditioned aerosol flows pass directly to dedicated, custom-built OPCs, designed to detect particles in the 100 nm to 3 μ m diameter size range. To ensure that particles pass through the 1.4 × 10−3 mm3 view volume of the collection optics, the aerosol (0.06 LPM) and a coaxial sheath flow (4 LPM) are focused by acceleration through a nozzle. Laboratory tests have shown that the sheath and aerosol streams do not mix. The view volume of the OPCs is determined by calculating the intersection volume of the cylindrical particle beam and the laser light sheet through which particles pass. The design of the laser light sheet is such that all particles that enter the OPC optical cavity pass through the light. The thickness of the laser light sheet is measured in two ways: (1) projecting the laser against a distant surface and then measuring the projected width with a vernier caliper while using a light meter to define the edges of the light; and (2) by using an oscilloscope to measure the pulse width and, therefore, the transit time of individual particles through the light sheet. The particle velocity is calculated using the known flow rate through the accelerating nozzle. By multiplying the velocity by the transit time, the thickness of the laser light sheet is determined. The two methods for measuring the light sheet thickness agree to within 15% and found a thickness of 0.015 mm. However, the light sheet does not have perfect edges. Therefore, larger particles are observed above the comparator threshold for slightly longer pulse widths.
To minimize size changes during measurements, the OPCs measure scattering of light from single particles as they pass through the laser light sheet originating from the diode laser (λ = 532 nm, World Star Technologies, Model TECGL-30). Scattered light is collected using an elliptical mirror from a wide angle (+ 64°/–50° in vertical plane and +/–74° in horizontal plane) in the near forward direction to integrate over the Mie resonances, thereby minimizing intensity oscillations that might otherwise lead to ambiguity in size determination. A cone of scattered light (+/–22°) emanating from the view volume along the axis of the laser is not collected due to a pass-thru hole in the elliptical mirror that is needed to allow the laser to exit the optical cavity. The mirror focuses the scattered light onto a photomultiplier tube (PMT, Hamamatsu Model H6779-02). RH, temperature (T), flow, and pressure (P) sensors monitor conditions of all flows, both upstream and downstream (except flow) of each OPC.
The DASH-SP employs two data acquisition systems: a high speed system to acquire the pulse data produced by the photomultipliers, and a second system to monitor signals from the slower sensors used to record T, RH, DMA size selection, DMA voltage, OPC gain settings, and so on. The signal from the PMT is processed through two gain stages, low gain for measurement of particles larger than about 450 nm, and high gain for particles in the 135 < D p < 450 nm size range. The instrument automatically selects the appropriate gain stage according to the size expected for that channel. A four-channel high speed digitizer (National Instruments™, Model PCI-6132) accumulates the logarithmically amplified, detected peak PMT signals from individual particles for count rates up to 2.5 × 106 s−1, corresponding to a concentration of 2.5 × 106 cm−3. Particle coincidence, which occurs when multiple particles are simultaneously in the OPC view volume, is a function of particle number concentration, view volume, and particle size. The coincidence performance of the OPC was validated by simultaneously sampling the same DMA-selected particles with two CPCs and the OPC. One CPC sampled upstream of the OPC, while the second CPC sampled downstream of the OPC. The maximum coincidence error associated with the laboratory and field measurements during this study is 3%, while the 1% particle coincidence concentration limit is approximately 5000 cm−3 and 350 cm−3 for the smallest (∼135 nm for high gain) and largest (∼450 nm for high gain) detectable particles, respectively, in the OPCs.
The DASH-SP can be operated manually or autonomously. In manual operation, the user specifies the RH for each humidifier, the size of particles to be selected by the DMA, and the number of particles to be sized by the OPC for each RH channel. The autonomous mode can step through a number of particle sizes and RHs, measuring a specified number of particles for each D p /RH combination. Changing the RH on any conditioning column slows the measurements due to the time required for the RH to stabilize after such a change. The typical RH equilibration time needed after a step change in RH from < 10% to be within ±1% of 74%, 85%, and 92% was determined to be 236 ± 61 s, 372 ± 106 s, and 569 ± 97 s, respectively (n = 11); these equilibration times represent an upper limit since smaller changes in RH within these broad ranges would ideally be carried out during experiments.
EXPERIMENTAL METHODS
A series of laboratory experiments were conducted to characterize the DASH-SP while it operated in the manual mode. For all characterization tests described below, test salt aerosols were employed. Salt solutions were prepared by mixing a known amount of salt with Milli-Q water, usually resulting in 0.2% (by mass) solutions. A compressed air source, with a downstream high efficiency particulate air (HEPA) filter to remove particulate contaminants, supplied flow to a stainless steel, constant-rate atomizer (CitationLiu and Lee 1975) and sheath air flows for the DMA and OPCs. A dilution flow system was used to maintain atomizer output particle number concentrations below 1000 cm−3 at a DMA-selected size.
The DASH-SP was also deployed on the Center for Interdisciplinary Remotely-Piloted Aircraft Studies (CIRPAS) Twin Otter aircraft in the July 2007 Marine Stratus/Stratocumulus Experiment (MASE II) campaign, in which airborne aerosol and cloud measurements were carried out off the central coast of California. For the duration of the field study, the instrument operated in the autonomous mode where the scan sizes and minimum OPC pulse count quantities were pre-programmed. We briefly present data from one particular flight to report the first airborne deployment of the DASH-SP; a more comprehensive analysis of data from this entire campaign will be presented in subsequent work.
INSTRUMENT CHARACTERIZATION
Size Limits of OPC Detection
The range of particle sizes in which characterization experiments are carried out depends on the OPC size detection limits. The optical properties of salts vary, so the size detection limit of the OPCs is a function of the composition of an aerosol sample. By sampling a set of 16 different species (10 inorganic, 6 organic), the minimum particle diameters detected with 50% efficiency in the OPCs were found to be 135 ± 8 nm and 234 ± 16 nm for high- and low-gain settings, respectively. The variation in OPC response as a function of refractive index is contained within the standard deviations, which reflect the variability among the ensemble of salts tested. The maximum size that can be detected accurately by the OPC is approximately 450 ± 30 nm with the high-gain setting, and beyond 1 μ m with the low-gain setting. However, uncertainties associated with multiply charged particles are enhanced when detecting particles of increasing size, especially near 1 μ m. Also, electrical saturation of the OPCs results in enhanced uncertainties starting at D p > 450 nm (high gain) and D p > 700 nm (low gain).
Time Resolution
The time resolution of the instrument is determined by three factors: (1) the transport delays between the point where particles enter the DASH-SP or, for a steady aerosol, where they enter the DMA, and the OPC detectors; (2) delays associated with programmed changes in the sampled particle size; and (3) the time required to obtain statistically significant counts in each OPC channel. A maximum transition time of 17 s is needed when switching between different DMA-selected sizes for normal operating conditions in order to ensure particles of the previous size have exited the system; this addresses the first two factors governing time resolution. The tubing length of each stream from the 5-way splitter (see ) to each humidification chamber and OPC is equivalent, which results in the same particle transit time in each channel. Tests were performed to estimate the minimum number of particles at a given size required to obtain a response with varying degrees of statistical significance. These tests indicate the length of time the instrument should sample at a given set of operating conditions to obtain representative growth factors. Sample sets of over 10,000 pulses were obtained for a selected particle size for different salts (D p = 150–404 nm). Pulses were randomly removed from each data file in a way to determine the variation of the modal pulse height and standard deviation as a function of decreasing counts at a fixed particle size. The ratio of standard deviation to modal pulse height is usually within 10% for pulse numbers ranging from 50 to 10,000. The variation of the modal pulse height is ±1% when comparing pulse counts of over 10,000 to any number of counts down to 50, below which the difference increases relatively quickly. In order for the standard deviation of observed pulse heights to be within 10%, 5%, and 1% of the original standard deviation calculated for a population of over 10,000 particles, an average of 60, 220, and 530 pulses are required, respectively. displays the time required to satisfy these different limits of the original modal pulse height and standard deviation for a wide range of particle number concentrations at a selected dry diameter. For example, for 10 particles cm−3 at a DMA-selected size, a minimum of 5 s is needed to be within 1% of the modal pulse height had over 10,000 particles of the same composition been sampled. An additional order of magnitude of time is required to be within 1% of the original standard deviation as compared to 1% of the original mode. As will be shown subsequently, the mode is the critical parameter in quantifying growth factors via the iterative data processing technique. Assuming a pulse height distribution with a distinct and dominant mode (there are often less dominant modes due to multiply charged particles), the data processing algorithm will determine the correct pulse height distribution mode regardless of the variance in the distribution. Thus, sampling time at a given D p /RH combination should be reduced to improve time resolution at the expense of variance, which ultimately has no effect on the accuracy of quantified growth factors.
FIG. 2 Time required as a function of particle number concentration to obtain sufficient pulses for the modal pulse height to be within 1% of the mode that would be obtained when counting at least 10,000 particles at constant particle composition. Also shown is the time needed for the standard deviation to be within 1%, 5%, and 10% of the standard deviation that would be obtained when counting at least 10,000 particles at constant particle composition.
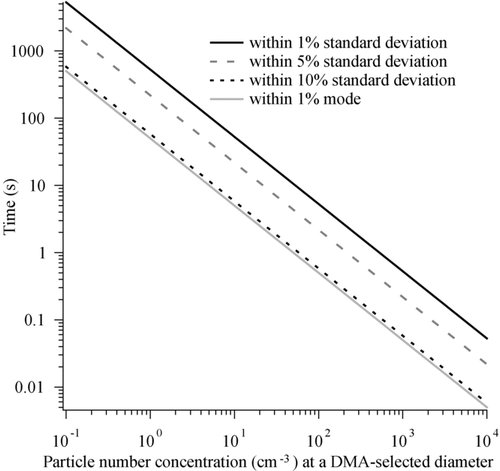
DASH-SP Accuracy, Precision, and Uncertainties
The uncertainty of the DASH-SP measurement of growth factor depends on the uncertainties in the RH and sizing (DMA and OPC) measurements. Critical to the accuracy of the RH measurements is the calibration procedure. One “reference” RH sensor (Model HMP50YCC1A2X, Vaisala) was initially calibrated over six saturated salt solutions within the 8–97% RH range. The remaining 12 RH sensors were then calibrated as an ensemble against the “reference” sensor in a temperature-controlled chamber through which a controlled mixture of dry and humid air was circulated. The accuracy of the RH measurements was evaluated by comparing theoretical deliquescence RH (DRH) for multiple salts with measurements. The DRHs of ammonium sulfate, sodium sulfate, and potassium chloride aerosols agreed with literature values (CitationSeinfeld and Pandis 2006) to within ±1.0%, while sodium chloride aerosols agreed to within ±1.6%. The overall RH measurement uncertainty was calculated by taking the summed root mean square errors of the measured OPC sample and sheath flow RHs, and combining this with the uncertainty associated with the RH sensors. The overall uncertainty was usually ±1.5%, while the RH sensor precision was ±0.7%.
Temperature fluctuations and gradients within each of the four humidification chamber-OPC units can lead to uncertainties due to the RH changing. Temperature sensors monitoring the sheath, sample, and exit flows of each OPC exhibited no significant change (Δ T < 1°C) during laboratory scans that lasted as long as ∼ 10 min, but temperatures did show a systematic increase (Δ T > 1°C) over longer periods of time. During aircraft flights lasting 5 h in which the DASH-SP was deployed, the twelve temperature sensors recorded the same level of variation. For example, the twelve sensors during one representative flight exhibited averages between 24.0–25.0°C and standard deviations between 3.0–3.5°C. However, the RH sensors exhibited significantly less variation due to the feedback control of the humidification by the instrument software: the RH standard deviation ranged between 0.1–1.8% for OPCs with upstream humidification chambers equilibrated at RHs of 74%, 85%, and 92%. Therefore, the RH variation resulting from temperature fluctuations in the humidification chamber-OPC units is normally within the uncertainty of the RH sensor measurements.
The instrument software calculates the DMA voltage required to classify a selected dry size for the specific geometry and operating parameters (T, P, flow rates) of the DMA. Since the DMA sheath flow rate is usually 10 times larger than the aerosol flow rate and relatively large particles are studied, the width of the sampled electrical mobility window is ±10%. DMA performance was frequently evaluated with calibrated PSL particles ranging in diameter between 152–1101 nm. Accurate DMA operation is exhibited when the maximum particle concentration is observed at the DMA-selected size that matches the actual size of the PSL particles, rather than a smaller or larger size. The maximum particle concentration was observed to occur at DMA-selected sizes within ±3% of the actual size of the PSL particles tested. It should be noted that the PSL particles studied have a reported size uncertainty ranging from ±1–3%. The average uncertainty associated with growth factor measurements is calculated to be ±4.3%, with an uncertainty as high as 8% at a dry size of 150 nm at lower RHs (< 80%).
Tests were done to check for contamination both in the form of leaks and in the source of Milli-Q water used in atomizing solutions. When feeding filtered air into the DASH-SP frequently over a period longer than 30 days, no particles were detected by the CPC and fewer than 1 cm−3 were detected by the OPC (high gain). Particles detected by the OPC corresponded to sizes below the 50% detection limit of the OPC (D p < 135 nm). Pure Milli-Q water was atomized into the DASH-SP system; no particles were detected by the CPC beyond 135 nm, and < 1 cm−3 were detected by the OPC (high gain). Below 135 nm, background particle concentrations measured by the CPC reached a value as high as 30 cm−3 at 5 nm; the OPC response at these lower sizes remained at < 1 cm−3. No background particle signals were observed using the low-gain OPC configuration for these tests. Since the experiments conducted with salts usually involved OPC number concentrations between 100–1000 cm−3 at a specific size, any background particles from the water source and inside the system itself are deemed negligible. Moreover, since no particles larger than 135 nm were observed by the OPC for these tests, contamination is negligible for ambient sampling, as the system is designed to sample at minimum dry D p exceeding 135 nm (150 nm for this study).
Stability
The stability in dry and wet particle data was studied. For dry data, stability was quantified over a time span of four months by calculating pulse height standard deviation relative to pulse height average (σ/) for both gain settings and different particle sizes (). Above 200 nm, at the high-gain OPC setting, σ/
is < 10%, with the species of greatest atmospheric relevance (ammonium sulfate, ammonium nitrate, sodium chloride) being < 3%. For the low-gain setting, σ/
is < 10% at 350 nm and ≤5% beyond 350 nm for all species studied. For wet studies, the stability in growth factor at different initial dry sizes and RHs between 30–92% was quantified for several species, where σ/
was usually < 3% for all species studied. In summary, the stability of the DASH-SP improves for both dry and wet particles with increasing size above the 50% detection limit of the OPC.
FIG. 3 Stability statistics for different species and sizes over a time span of four months. Panel A corresponds to the high-gain OPC setting, and Panel B represents the low-gain setting for dry tests. Panel C represents stability statistics for growth factor during wet studies using the high-gain OPC configuration (n > 20 for each salt in the bottom panel).
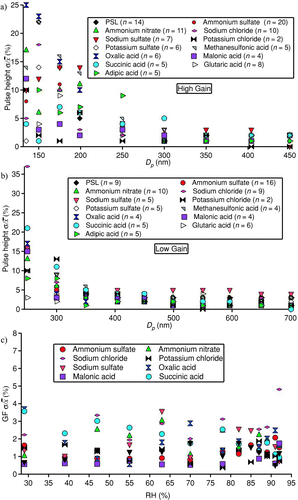
DATA ANALYSIS
Data resulting from the collection of scattered light from individual particles are returned by the OPCs as pulse heights, the amplitudes of which depend on particle size, shape, and refractive index, the latter depending on particle composition. For the purposes of pulse height data interpretation, the following assumptions are made: (1) purely scattering, spherical particles (one exception to the sphericity assumption for growth factor calculations is that a shape factor of 1.08 was assumed for dry sodium chloride particles, thought to be cubic, prior to humidification (CitationHinds 1999; CitationHameri et al. 2001)); (2) the DMA-selected particle size corresponds to the mode of the true particle size distribution, and therefore, the mode of the OPC pulse height distribution; and (3) for particles that undergo humidification, the mode of the OPC pulse height distribution represents the true wet particle size. The first assumption is an approximation for ambient particles, but has been commonly made in previous DMA-OPC studies (CitationStolzenburg et al. 1998; CitationHand et al. 2000; CitationAmes et al. 2000; CitationKreisberg et al. 2001; CitationHand and Kreidenweis 2002). Growth factors are calculated using the volume-equivalent diameter (D p ), while the DMA classifies particles based on their mobility-equivalent diameter (D p,mob ), the two diameters of which are only equivalent for spherical particles. Following the methodology of CitationGysel et al. (2002), employing a shape factor of 1.08 for dry sodium chloride particles results in a value of 0.96 for the ratio D p :D p,mob . This provides a representative indication of the sensitivity of growth factor measurements to particles with one type of shape effect (cubic rather than spherical); without the shape factor correction, growth factors for sodium chloride particles would be underestimated by 4%. The volume of water in particles after humidification is often substantial; the assumption that particles are spherical and scatter, but do not absorb, light is generally valid for those particles for which growth is significant.
Before the pulse height distribution modes can be used to determine the particle size and refractive index, dry particle calibrations must be performed to accumulate sufficient data relating pulse height, particle size, and refractive index. OPC calibrations were carried out for a set of six salts of known refractive index (1.39, 1.47, 1.50, 1.52, 1.54, and 1.60; salts listed in ) (CitationWeast 1987; CitationLide 2005) by measuring OPC pulse height response to a range of DMA-selected diameters (150, 175, 200, 225, 250, 300, 350, 375, 404, and 415 nm) without humidification. summarizes the OPC response as a function of dry particle size for salts of different refractive index from one set of calibrations. For each OPC, high-order polynomial equations accurately represent the relationship between OPC pulse height and particle dry diameter for different refractive indices (R 2 > 0.995), allowing calculation of size from a given refractive index and OPC pulse height:
FIG. 4 OPC response as a function of dry particle size for salts of different refractive index. These results are from one OPC for a single set of calibrations performed for each salt. All OPCs show the same general behavior.
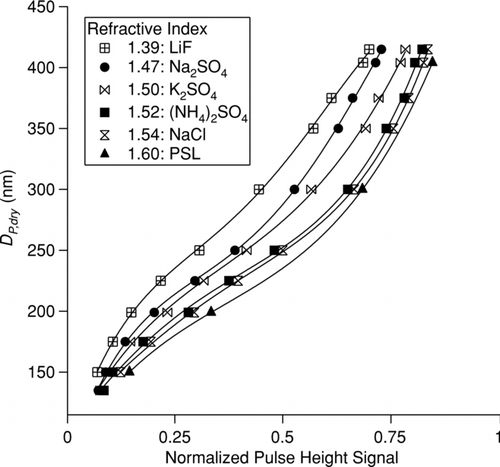
summarizes the algorithm used to calculate “effective” refractive index and growth factor from the dry and wet pulse height distribution modes. Initially, the dry pulse height distribution mode is used to iterate on a two-dimensional surface (n vs pulse height, fixed D p ) to determine the dry particle “effective” refractive index. After guessing a value for the wet particle diameter, a volume-weighted refractive index is calculated to account for the refractive index of both water and the dry particle. Using the wet particle pulse height data, iterations are carried out on the three-dimensional surface to converge on a value for the humidified particle's “effective” refractive index, which is subsequently used to determine the wet particle diameter and growth factor. This iterative process is not possible without a priori knowledge of the dry particle refractive index, which requires one of the four humidification chambers to be operated in the dry state. This algorithm assumes that particles are internally mixed and that particulate matter is completely dissolved in humidified particles.
FIG. 5 Data processing procedure from DASH-SP raw pulse height data to growth factors (GFs) for both laboratory-generated aerosol, for which the composition and refractive index (n) of the dry particles are known, and for ambient aerosol, where the composition and refractive index of the dry particles are unknown. The dry pulse height distribution mode is first used to determine the dry particle refractive index, which is used to calculate a volume-weighted wet particle refractive index, taking into account the volume fractions of water and the particle. Iterations are subsequently carried out on the three-dimensional surface shown to converge on a wet particle refractive index. The D p corresponding to the latter value of refractive index and the wet pulse height distribution mode is subsequently used to calculate growth factor.
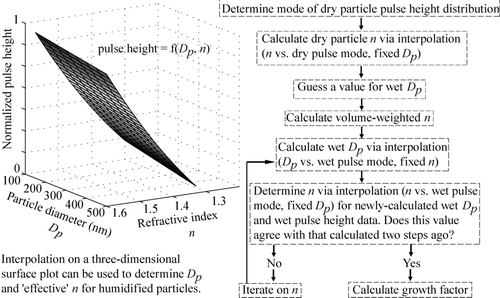
One exception to the iterative procedure for humidified particles is when testing laboratory-generated aerosols with known dry-particle refractive indices; a thermodynamic model of particle water uptake (CitationBrechtel and Kreidenweis 2000a, Citation2000b) can predict the refractive index for a specific salt at a given RH. Only one step is then needed to interpolate on the three-dimensional surface (D p vs. pulse height, fixed n) to determine the true wet particle size. The modified Köhler model used includes a parameterization for the solution osmotic coefficient that approaches the correct thermodynamic limit as the solution becomes infinitely dilute (CitationBrechtel and Kreidenweis 2000a, Citation2000b). Predictions from both this model and the Aerosol Inorganics Model (AIM) (CitationClegg et al. 1998; http://www.aim.env.uea.ac.uk/aim/aim.html) are used below for comparison with laboratory data for a number of salts.
GROWTH FACTOR MEASUREMENTS
Inorganic Salts
Experiments were carried out to determine growth factor values as a function of RH, from 10% to 94%, for different inorganic species (ammonium nitrate, ammonium sulfate, ammonium nitrate, sodium chloride, potassium chloride, and sodium sulfate) and organic acid species (oxalic, glyoxylic, malonic, succinic, glutaric, and adipic acids). The growth factors for these species are either known or can be calculated theoretically to assess the accuracy of the DASH-SP measurements. However, only the inorganics provide a firm comparison between theory and/or laboratory measurements with those of the DASH-SP since growth factors for organic acids are not as well established as those for inorganic salts.
shows the measured hygroscopic growth curves for various inorganic and organic acid species. With the exception of the organic acids and ammonium nitrate, a sharp increase in growth factor is observed at the DRH, providing evidence for the homogeneity of the humidity field experienced by the particles. The measured growth factors of the inorganic species agree well with thermodynamic theory for RH values above the DRH (±1%). For ammonium sulfate at an RH of 92% the discrepancy is ±4% based on the Brechtel and Kreidenweis (Citation2000a, Citation2000b) model, and ±1% based on the CitationClegg et al. (1998) model. Growth factor measurements at RH values above the DRH show the following levels of agreement with thermodynamic theory: sodium chloride (1.0% above theory, σ = 2.3%, n > 50), ammonium sulfate (1.5% above theory, σ = 1.7%, n > 50), and sodium sulfate (2.0% below theory, σ = 2.4%, n > 50). Ammonium nitrate particles have been shown to remain “wet” at RHs as low as 8% (CitationDougle et al. 1998; CitationLightstone et al. 2000), which explains why the observed growth factors exceed unity for RHs below the DRH. Multiple growth factor curves are reported for ammonium nitrate in to show that at sizes near 250 nm, the growth factors increase with larger dry particle sizes.
FIG. 6 Experimentally determined growth factor curves for inorganic and organic acid species. Predictions from the Brechtel and Kreidenweis (Citation2000a, Citation2000b) model are shown for all salts except for glyoxylic acid and potassium chloride, while predictions from the CitationClegg et al. (1998) model are also shown for ammonium sulfate. It should be noted that these two models differ in that the Brechtel and Kreidenweis (Citation2000a, Citation2000b) model includes the Kelvin term to account for droplet curvature. Sampled particles within the size range of the DASH-SP are sufficiently large so that the Kelvin effect correction is relatively small. The error bars reflect uncertainties associated with the RH and sizing (DMA and OPC) measurements. The reported deliquescence RH (DRH) and efflorescence RH (ERH) values are from the following references: CitationSaxena and Hildemann (1997) and CitationPeng et al. (2001) for organic acids, and CitationSeinfeld and Pandis (2006) for the inorganic species.
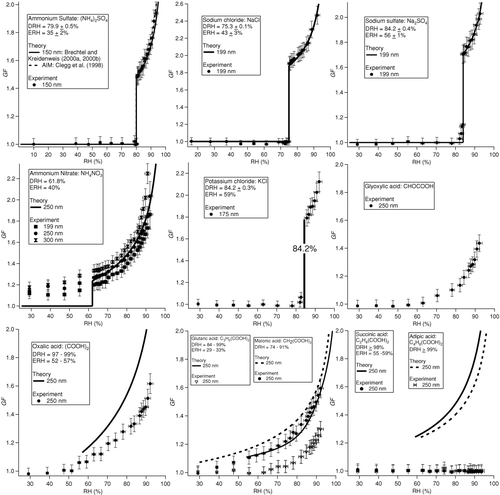
Organic Acids
In contrast to inorganic species, the hygroscopic properties of organic aerosol species are not as well known. Organic acids are an important component of atmospheric aerosols (CitationKawamura and Ikushima 1993; CitationKhwaja 1995; CitationChebbi and Carlier 1996; CitationSaxena and Hildemann 1996; CitationKawamura and Sakaguchi 1999; CitationKerminen et al. 2000; CitationYao et al. 2004; CitationHuang et al. 2005; CitationFisseha et al. 2006; CitationSorooshian et al. 2007a), the presence of which can affect the hygroscopicity of ambient particles (CitationSaxena et al. 1995; CitationHansson et al. 1998; CitationLi et al. 1998; CitationAnsari and Pandis 2000; CitationCruz and Pandis 2000; CitationPeng et al. 2001; CitationPeng and Chan 2001; CitationPrenni et al. 2001, Citation2003; CitationWise et al. 2003). Two- to six-carbon dicarboxylic acids are investigated here, in addition to glyoxylic acid, which is a direct precursor to oxalic acid, the most abundant particulate dicarboxylic acid in the atmosphere (CitationKawamura and Ikushima 1993; CitationKawamura and Sakaguchi 1999; CitationKerminen et al. 2000; CitationPoore 2000; CitationYao et al. 2004; CitationCrahan et al. 2004; CitationYu et al. 2005; CitationHuang et al. 2005, Citation2006; CitationSorooshian et al. 2006a, Citation2007a, Citation2007b).
Oxalic (C2), malonic (C3), glyoxylic (C2), and glutaric (C5) acids do not exhibit a DRH, but rather grow gradually with increasing RH (). This result is consistent with previous studies which showed that organic acids absorb water at all RHs (CitationSaxena and Hildemann 1997; CitationDougle et al. 1998). Unlike the other organic acids studied, succinic (C4) and adipic (C6) acids exhibited no hygroscopic growth over the RH range studied, which is consistent with the HTDMA results of CitationPrenni et al. (2001). With the exception of glyoxylic acid, previous studies have either measured or calculated DRHs for these organic acids; only malonic (DRH = 74–91%) and glutaric (DRH = 84–99%) acids exhibit DRHs in the range of RHs tested in this study, while the rest of the organic acids exhibit DRHs exceeding 97% (CitationSaxena and Hildemann 1997; CitationPeng et al. 2001).
Excluding oxalic and glyoxylic acids, the experimental data in indicate a clear odd-even carbon number effect, where the odd-carbon number organic acids (malonic and glutaric acids) exhibit relatively more hygroscopic growth as compared to the even-carbon number acids (succinic and adipic acids). This behavior is consistent with the literature (CitationSaxena and Hildemann 1996; CitationPrenni et al. 2001); solubility alternates with the number of carbon atoms in the organic acid molecule up to C10. Oxalic acid is significantly more hygroscopic than succinic and adipic acids, the other two even carbon-number dicarboxylic acids.
Growth factor values for dicarboxylic acids measured in this study tend to be lower than those predicted by the Brechtel and Kreidenweis model (Citation2000a, Citation2000b). The growth factors for malonic acid showed the best agreement with predictions as compared to the other organic acids, with a growth factor that is at most 6% below that predicted. The measured growth factor for glutaric acid was at most 17% below predictions. Malonic and oxalic acids exhibited relatively similar growth factors up to an RH of 92% (1.53 ± 0.07, 8% below theory, and 1.58 ± 0.07, 26% below theory, respectively), while glutaric acid showed less growth (1.27 ± 0.05, 19% below theory). Because thermodynamic parameters are not available for glyoxylic acid, only experimental results are shown; glyoxylic acid exhibits similar hygroscopic behavior when compared to oxalic, malonic, and glutaric acids. Glyoxylic acid has a measured growth factor of 1.44 ± 0.06 at an RH of 92%.
Five potential explanations for the disagreement between measurements and predictions for the dicarboxylic acid growth factors include: (1) uncertainties in the model parameters used for each dicarboxylic acid (osmotic coefficient, surface tension, solution density); (2) particles did not crystallize during drying prior to size-selection by the DMA and, therefore, do not properly reflect the quantity of solute; (3) particles were non-spherical; (4) volatilization of the organic acids occurred; and (5) the dicarboxylic acid particles exhibit slower water uptake than the inorganic species studied and did not have enough time to reach equilibrium at a given wet RH. The extent to which each of the five aforementioned explanations leads to the discrepancy between predictions and measurements is not known. It is noted that the second, third, and fifth explanations would ideally lead to an underestimate of the growth factor. In particular, there is less known about the morphology of organics as compared to inorganic species, therefore, if the organic acid particles were not spherical or compact, then the experimentally determined growth factors are less than their actual values from a morphology standpoint. The measurements do provide evidence that ambient particles that contain organic acids, specifically oxalic, malonic, glutaric, and glyoxylic acids, experience growth at low RHs (< 65%).
VALIDATION OF ITERATIVE DATA PROCESSING CODE
In order to assess the validity of the data processing technique for interpreting ambient data, the iterative procedure outlined in was applied to data collected from wet ammonium sulfate experiments to quantify “effective” refractive index and growth factor as a function of RH. The three wet RHs used in the field study subsequently discussed, 74%, 85%, and 92%, were also applied in these wet ammonium sulfate tests (n > 15 at each RH). The measurements that have been processed with the iterative code are compared to predictions of the Brechtel and Kreidenweis (Citation2000a, Citation2000b) and CitationClegg et al. (1998) models. shows that, although the calculated “effective” refractive index at an RH of 74% agrees perfectly with the literature (CitationWeast 1987), the DASH-derived average growth factors exceed theory at this RH by as much as 8%. The measured growth factors at RHs of 85% and 92% lie within one standard deviation of the model predictions, whereas the “effective” refractive indices agree to within 1%. This is a result of the fact that the calculation of growth factor in the iterative data processing code is more sensitive to DMA, OPC, and RH uncertainties as compared to calculation of refractive indices.
FIG. 7 Comparison between two thermodynamic models and DASH-SP data for ammonium sulfate particles. The reported DASH-SP results were calculated by using iterative procedure in to process the OPC pulse height data. The RH error bars reflect uncertainties in the RH measurement, while the GF error bars reflect the precision of the measurement after several repetitive trials.
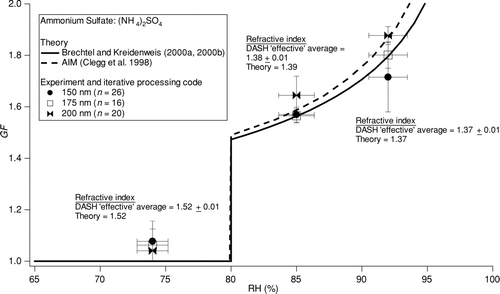
(A–C) shows examples of the dry and wet size distributions from wet ammonium sulfate data processed with the iterative code. Size distribution data are presented at four RHs (one dry, and three wet RHs) for each of three initial dry sizes (150, 175, and 200 nm). The size distributions for dry particles and those at an RH value of 74% should be identical since ammonium sulfate particles deliquesce at an RH of 79.9 ± 0.5% (CitationSeinfeld and Pandis 2006). However, the mode diameters of the size distributions at RH = 74% slightly exceed those of the dry size distributions. The dry and RH = 74% size distributions are broader at a dry size of 150 nm than at the two larger sizes; this broadening behavior is normally observed when the particle size is close to the minimum OPC detection limit. There is variation in the growth factors at constant RH, as the range in values at RHs of 74%, 85%, and 92% is 0.04 (average GF = 1.06), 0.08 (average GF = 1.59), and 0.16 (average GF = 1.80), respectively. The differences in growth factor at constant RH for various dry sizes is attributable to uncertainties in the measurements and the iterative data processing procedure, particularly the cubic and high-order polynomial equations. However, all of the growth factors reported in are within 10% of predictions from the Brechtel and Kreidenweis (Citation2000a, Citation2000b) and CitationClegg et al. (1998) models.
FIG. 8 Size distributions from wet ammonium sulfate and dry PSL aerosol laboratory tests. (a), (b), and (c) correspond to ammonium sulfate tests in which DMA-selected dry particle sizes of 150, 175, and 200 nm, respectively, were exposed to RHs of < 8% (dry), 74%, 85%, and 92%. Each curve is labeled with its calculated growth factor and “effective” refractive index (n), as calculated from the iterative data processing procedure in . (d) corresponds to dry PSL size distributions to show that for spherical particles the distributions are narrower than those for salts that may not be perfectly spherical. The size distributions tend to be broader near the minimum and maximum size detection limits of the OPCs.
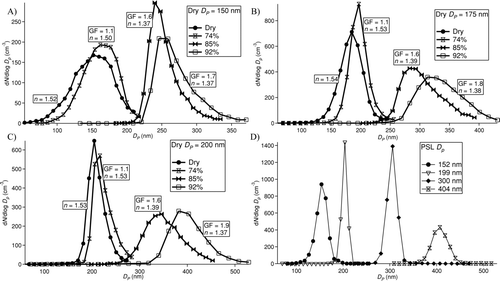
One would expect narrower size distributions for the dry ammonium sulfate results in since the DMA is selecting one size. To determine whether the broadness of the dry ammonium sulfate size distributions is caused by an instrument issue or by impurities and morphology issues associated with ammonium sulfate particles, dry PSL size distributions were also computed since PSL particles are spherical (). The PSL size distributions are considerably narrower than those of dry ammonium sulfate particles, suggesting that the latter aerosol likely consisted of non-spherical particles that affect the final optical sizing; particle shape variations can significantly influence their scattering and absorption properties (CitationChylek et al. 1984; CitationVideen et al. 1997). The PSL size distributions for particle diameters of 152 nm and 404 nm were slightly broader than distributions at diameters of 199 nm and 300 nm, providing further evidence that the OPCs have somewhat lower size resolution near their minimum and maximum size detection limits. It is unlikely that particle coincidence plays a role in the broadening behavior apparent in . If this were the case, the same shape would be observed in the dry PSL distributions for all sizes shown. It is possible, however, that some of the broadening is also due to the particles experiencing a range of RHs.
These results suggest that the iterative procedure is capable of accurately determining refractive indices and providing dry and wet size distributions. Although the distributions may be broader close to the OPC size detection limits, the modes of these distributions can still be used to accurately calculate growth factors.
FIELD IMPLEMENTATION OF DASH-SP
On July 16, 2007, by prior arrangement, the Twin Otter intercepted and repeatedly sampled the exhaust plume of a large PANAMAX container ship with a 55 MW main engine operating on heavy fuel oil. shows the flight tracks during the ship experiment. The aircraft first flew up the plume toward the ship, which was steaming directly into the wind, at an altitude of 30 m; this procedure was repeated three more times. The aircraft also performed several cross-wind transects of the plume at various altitudes in the marine boundary layer (< 250 m), which was clear on this day. Inside the plume, submicrometer particle number concentrations reached as high as 365,000 cm−3, as determined with a differential mobility analyzer with a time resolution of ∼73 s, whereas the background aerosol concentration outside of the plume was < 500 cm−3. The plume was ∼0.4 km wide during the first downwind transect at an altitude of 30 m and it was 2 km wide during the second to last transect at an altitude of 150 m (the plume was not detected in the last transect at 210 m).
FIG. 9 (a) Flight tracks during a MASE II flight (July 16, 2007) during which the Twin Otter aircraft sampled emissions from a large cargo ship off the central coast of California. The duration of the ship exhaust plume measurements was approximately 2.5 h. The plane flew at an altitude of 30 m in the ship's plume, before performing multiple cross-wind transects of the plume at the labeled altitudes. (b) Water-soluble species concentrations as measured by a PILS onboard the Twin Otter. The four, black vertical lines indicate when the aircraft was immediately behind the ship. (c) Growth factors at an RH of 92%. Marker sizes are proportional to the submicrometer particle number concentration (range = 50–365,000 cm−3), as determined by a DMA.
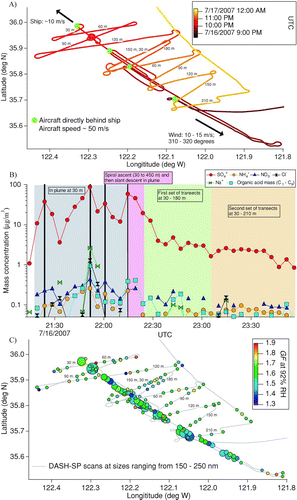
displays the water-soluble aerosol composition inside the plume as measured every 4.5 min (equivalent to a flown distance of ∼13.5 km) by a particle-into-liquid sampler (PILS; Brechtel Manufacturing Inc.) (CitationSorooshian et al. 2006b). The water-soluble aerosol mass, which reached values as high 93 μ g m− 3 just behind the ship, was composed mainly of sulfate (96.2% ± 4.0% of water-soluble mass; n = 15). Other water-soluble components of the aerosol included Na+ (1.0% ± 2.4%), NO3 − (1.0% ± 0.6%), organic acids (0.6% ± 0.3%), Cl− (0.6% ± 0.9%), and NH4 + (0.5% ± 0.4%). The aerosol was highly acidic, as the ammonium-to-sulfate molar ratio was, on average, 0.03. The ratio of water-soluble mass to the DMA-observed volume concentration of the aerosol was 1.13 g cm−3 ± 0.30 g cm− 3, suggesting that a significant fraction of the aerosol was accounted for by the PILS data, assuming that the ambient aerosol density was close to that of sulfuric acid (1.84 g cm− 3). As observed by an Aerodyne time-of-flight aerosol mass spectrometer, the aerosol contained a large proportion of hydrophobic organic species that were not detected by the PILS. It is likely that some of the hydrophobic species were light-absorbing, like soot, which introduces error in the iterative data processing algorithm because of non-zero imaginary components of the refractive index.
DASH-SP data are presented for the following dry diameters: 150, 175, 200, and 250 nm. The instrument was pre-programmed to detect a minimum of 300 pulses in each OPC channel for this flight. For 150 and 175 nm particles, 1 s and 10 s were usually required before all OPCs detected at least 300 pulses inside and outside of the ship plume, respectively. For 200 and 250 nm particles, 10–30 s and 10–60 s were required inside and outside of the plume, respectively.
The average dry “effective” refractive index of aerosol in the ship plume was determined to be 1.53 ± 0.04, while the “effective” refractive index in the background aerosol outside of the plume was 1.49 ± 0.04. It should be noted that the refractive indices of sulfuric acid, ammonium bisulfate, and ammonium sulfate are 1.41, 1.48, and 1.52, respectively (CitationWeast 1987; CitationAmes et al. 2000). The time resolution of the DASH-SP is evident in , which shows the spatial distribution of growth factors at an RH of 92%. In the ship plume, the average growth factors at RHs of 74%, 85%, and 92% were 1.11 ± 0.04, 1.46 ± 0.09, and 1.53 ± 0.10, respectively. The corresponding growth factors in the background aerosol were 1.19 ± 0.09 (RH = 74%), 1.54 ± 0.05 (RH = 85%), and 1.63 ± 0.08 (RH = 92%). Therefore, the overall averages suggest that there was a decrease in hygroscopicity inside the ship plume as compared to the background marine aerosol at RHs ≥74%. There was no significant difference in growth factor as the plane flew closer to the ship in its plume from a downwind distance greater than 30 km. We hypothesize that this occurred because the relative mass fractions of acidic sulfate and hydrocarbon-like organic species did not vary appreciably over the range of timescales of transport between the ship and aircraft. The observed growth factors in the plume are lower than what would be expected for pure sulfuric acid, and this is likely a result of the presence of other species, such as hydrophobic organics, in the particles that reduced the hygroscopicity.
CONCLUSIONS
This study introduces a new instrument, termed the differential aerosol sizing and hygroscopicity spectrometer probe (DASH-SP). In the instrument, a DMA-selected monodisperse aerosol flow is split into five flows, one to each of four OPCs with its own upstream humidification column, and one to a CPC. The DASH-SP has been designed for aircraft operation and other applications where hygroscopic growth observations are required at multiple sizes and RHs with high time resolution. An iterative data processing algorithm calculates growth factors and “effective” refractive indices for humidified particles based on a calculated value of the “effective” dry particle refractive index; iterations are performed on a three-dimensional surface (pulse height – refractive index – D p ) based on dry particle calibration data from several salts with known refractive indices.
Laboratory tests were performed to characterize the instrument's performance. The minimum particle diameter detected with 50% efficiency in the OPCs is 135 ± 8 nm; particles with diameters > 1 μ m are detected by the OPCs using the low-gain setting, subject to increasing uncertainty as a function of growing size due to multiply charged particles and electrical saturation of the OPCs. Estimates for the time required to obtain statistically significant counts in each OPC channel were reported for a wide range of particle number concentrations. The RH uncertainty is ±1.5% with an average uncertainty of ±4.3% for growth factor measurements. Measured DRHs of ammonium sulfate, sodium sulfate, and potassium chloride aerosols agree with literature values to within ±1%, while that for sodium chloride aerosols agrees to within ±1.6%. A high level of stability exists in the dry salt calibrations and growth factors for various salts at RHs up to 92%, especially at particle diameters larger than the 50% detection limit of the OPCs.
Excellent agreement exists between growth factor data for several inorganic salts and predictions from a thermodynamic model. Growth factor data for organic acids show that, with the exception of oxalic and glyoxylic acids (both C2 acids), the organic acids with an odd number of carbons (malonic and glutaric acids) exhibit greater hygroscopic growth than those with an even number (succinic and adipic acids). Succinic and adipic acids exhibit no hygroscopic growth up to an RH of 94%, while the other organic acids show gradual growth starting at low RHs (< 65%). Oxalic acid, the most abundant organic acid in ambient aerosols, exhibits the greatest hygroscopic growth among all of the organic acids studied.
The DASH-SP was successfully deployed in the 2007 Marine Stratus/Stratocumulus Experiment (MASE II) campaign on board the CIRPAS Twin Otter aircraft. Results are presented here for one flight during this study in which the plume from a large container ship was sampled. Growth factors of the exhaust plume aerosol downwind of this ship at RHs of 74%, 85%, and 92% were 1.11 ± 0.04, 1.46 ± 0.09, and 1.53 ± 0.10, respectively. The field data demonstrate that the DASH-SP is capable of providing first-of-a-kind, rapid, simultaneous, size-resolved aerosol hygroscopic growth measurements at multiple RHs. Future work will address the instrument response to non-spherical and light-absorbing particles.
Acknowledgments
Brechtel Mfg. Inc. acknowledges the support of Dr. Ronald Ferek and the ONR SBIR Program under grant N00014-05-C-0016. This work was also supported by the Office of Naval Research grant N00014-04-1-0118. We appreciate valuable comments and suggestions by Jeff Snider.
REFERENCES
- Aklilu , Y. , Mozurkewich , M. , Prenni , A. J. , Kreidenweis , S. M. , Alfarra , M. R. , Allan , J. D. , Anlauf , K. , Brook , J. , Leaitch , W. R. , Sharma , S. , Boudries , H. and Worsnop , D. R. 2006 . Hygroscopicity of Particles at Two Rural, Urban Influenced Sites During Pacific 2001: Comparison with Estimates of Water Uptake from Particle Composition . Atmos. Environ. , 40 : 2650 – 2661 .
- Ames , R. B. , Hand , J. L. , Kreidenweis , S. M. , Day , D. E. and Malm , W. C. 2000 . Optical Measurements of Aerosol Size Distributions in Great Smoky Mountains National Park: Dry Aerosol Characterization . J. Air Waste Manage. , 50 : 665 – 676 .
- Ansari , A. S. and Pandis , S. N. 2000 . Water Absorption by Secondary Organic Aerosol and its Effect an Inorganic Aerosol Behavior . Environ. Sci. Technol. , 34 : 71 – 77 .
- Baltensperger , U. , Streit , N. , Weingartner , E. , Nyeki , S. , Prevot , A. S. H. , Van Dingenen , R. , Virkkula , A. , Putaud , J. P. , Even , A. , ten Brink , H. , Blatter , A. , Neftel , A. and Gaggeler , H. W. 2002 . Urban and Rural Aerosol Characterization of Summer Smog Events During the PIPAPO Field Campaign in Milan, Italy . J. Geophys. Res. , 107 ( D22 ) doi:10.1029/2001JD001292
- Berg , O. H. , Swietlicki , E. and Krejci , R. 1998 . Hygroscopic Growth of Aerosol Particles in the Marine Boundary Layer Over the Pacific and Southern Oceans During the First Aerosol Characterization Experiment (ACE 1) . J. Geophys. Res. , 103 ( D13 ) : 16535 – 16545 .
- Brand , P. , Ruoss , K. and Gebhart , J. 1992 . Performance of a Mobile Aerosol Spectrometer for an Insitu Characterization of Environmental Aerosols in Frankfurt City . Atmos. Environ , 26 : 2451 – 2457 .
- Brechtel , F. J. and Kreidenweis , S. M. 2000a . Predicting Particle Critical Supersaturation from Hygroscopic Growth Measurements in the Humidified TDMA. Part I: Theory and Sensitivity Studies . J. Atmos. Sci. , 57 : 1854 – 1871 .
- Brechtel , F. J. and Kreidenweis , S. M. 2000b . Predicting Particle Critical Supersaturation from Hygroscopic Growth Measurements in the Humidified TDMA. Part II: Laboratory and Ambient Studies . J. Atmos. Sci. , 57 : 1872 – 1887 .
- Busch , B. , Kandler , K. , Schutz , L. and Neususs , C. 2002 . Hygroscopic Properties and Water-Soluble Volume Fraction of Atmospheric Particles in the Diameter Range from 50 nm to 3.8 μ m During LACE 98 . J. Geophys. Res. , 107 ( D21 ) doi:10.1029/2000JD000228
- Carrico , C. M. , Rood , M. J. and Ogren , J. A. 1998 . Aerosol Light Scattering Properties at Cape Grim, Tasmania, During the First Aerosol Characterization Experiment (ACE 1) . J. Geophys. Res. , 103 ( D13 ) : 16565 – 16574 .
- Carrico , C. M. , Rood , M. J. , Ogren , J. A. , Neususs , C. , Wiedensohler , A. and Heintzenberg , J. 2000 . Aerosol Optical Properties at Sagres, Portugal During ACE-2 . Tellus B , 52 : 694 – 715 .
- Carrico , C. M. , Kreidenweis , S. M. , Malm , W. C. , Day , D. E. , Lee , T. , Carrillo , J. , McMeeking , G. R. and Collett , J. L. 2005 . Hygroscopic Growth Behavior of a Carbon-Dominated Aerosol in Yosemite National Park . Atmos. Environ. , 39 : 1393 – 1404 .
- Chan , C. K. , Kwok , C. S. and Chow , A. H. L. 1997 . Study of Hygroscopic Properties of Aqueous Mixtures of Disodium Fluorescein and Sodium Chloride Using an Electrodynamic Balance . Pharmaceut. Res. , 14 : 1171 – 1175 .
- Chebbi , A. and Carlier , P. 1996 . Carboxylic Acids in the Troposphere, Occurrence, Sources, and Sinks: A Review . Atmos. Environ. , 30 : 4233 – 4249 .
- Chylek , P. , Ramaswamy , V. and Cheng , R. J. 1984 . Effect of Graphitic Carbon on the Albedo of Clouds . J. Atmos. Sci , 41 : 3076 – 3084 .
- Clegg , S. L. , Brimblecombe , P. and Wexler , A. S. 1998 . A Thermodynamic Model of the System H+ – NH4+ – Na+ – SO42− – NO3− – Cl− – H2O at 298.15 K . J. Phys. Chem. A. , 102 : 2155 – 2171 .
- Cocker , D. R. , Whitlock , N. E. , Flagan , R. C. and Seinfeld , J. H. 2001 . Hygroscopic Properties of Pasadena, California Aerosol . Aerosol Sci. Tech. , 35 : 637 – 647 .
- Cohen , M. D. , Flagan , R. C. and Seinfeld , J. H. 1987a . Studies of Concentrated Electrolyte-Solutions Using the Electrodynamic Balance. 1. Water Activities for Single-Electrolyte Solutions . J. Phys. Chem , 91 : 4563 – 4574 .
- Cohen , M. D. , Flagan , R. C. and Seinfeld , J. H. 1987b . Studies of Concentrated Electrolyte-Solutions Using the Electrodynamic Balance. 2. Water Activities for Mixed-Electrolyte Solutions . J. Phys. Chem , 91 : 4575 – 4582 .
- Covert , D. S. , Heintzenberg , J. and Hansson , H. C. 1990 . Electrooptical Detection of External Mixtures in Aerosols . Aerosol Sci. Tech , 12 : 446 – 456 .
- Covert , D. S. , Hansson , H.-C. , Winkler , P. and Heintzenberg , J. The Degree of Mixing of Hygroscopic Properties in Source and Receptor Locations in Northern Europe . Proceedings of AAAR 1991 . October 7–11 1991 , Travers City, Michigan, USA.
- Covert , D. S. and Heintzenberg , J. 1993 . Size Distributions and Chemical-Properties of Aerosol at Ny Alesund, Svalbard . Atmos. Environ. , 27 : 2989 – 2997 .
- Crahan , K. K. , Hegg , D. , Covert , D. S. and Jonsson , H. 2004 . An Exploration of Aqueous Oxalic Acid Production in the Coastal Marine Atmosphere . Atmos. Environ. , 38 : 3757 – 3764 .
- Cruz , C. N. and Pandis , S. N. 2000 . Deliquescence and Hygroscopic Growth of Mixed Inorganic-Organic Atmospheric Aerosol . Environ. Sci. Technol. , 34 : 4313 – 4319 .
- Dougle , P. G. , Veefkind , J. P. and ten Brink , H. M. 1998 . Crystallisation of Mixtures of Ammonium Nitrate, Ammonium Sulphate and Soot . J. Aerosol Sci. , 29 : 375 – 386 .
- Ferron , G. A. , Karg , E. , Busch , B. and Heyder , J. 2005 . Ambient Particles at an Urban, Semi-Urban and Rural Site in Central Europe: Hygroscopic Properties . Atmos. Environ. , 39 : 343 – 352 .
- Fisseha , R. , Dommen , J. , Gaeggeler , K. , Weingartner , E. , Samburova , V. , Kalberer , M. and Baltensperger , U. 2006 . Online Gas and Aerosol Measurement of Water Soluble Carboxylic Acids in Zurich . J. Geophys. Res. , 111 ( D12316 ) doi:10.1029/2005JD006782
- Flagan , R. C. 1999 . On Differential Mobility Analyzer Resolution . Aerosol Sci. Tech , 30 : 556 – 570 .
- Gasparini , R. , Li , R. J. , Collins , D. R. , Ferrare , R. A. and Brackett , V. G. 2006 . Application of Aerosol Hygroscopicity Measured at the Atmospheric Radiation Measurement Program's Southern Great Plains Site to Examine Composition and Evolution . J. Geophys. Res. , 111 ( D5 ) doi:10.1029/2004JD005448
- Gysel , M. , Weingartner , E. and Baltensperger , U. 2002 . Hygroscopicity of Aerosol Particles at Low Temperatures. 2. Theoretical and Experimental Hygroscopic Properties of Laboratory Generated Aerosols . Environ. Sci. Technol. , 36 : 63 – 68 .
- Gysel , M. , Weingartner , E. , Nyeki , S. , Paulsen , D. , Baltensperger , U. , Galambos , I. and Kiss , G. 2004 . Hygroscopic Properties of Water-Soluble Matter and Humic-Like Organics in Atmospheric Fine Aerosol . Atmos. Chem. Phys. , 4 : 35 – 50 .
- Hameri , K. , Laaksonen , A. , Vakeva , M. and Suni , T. 2001 . Hygroscopic Growth of Ultrafine Sodium Chloride Particles . J. Geophys. Res. , 106 ( D18 ) : 20749 – 20757 .
- Hameri , K. , Vakeva , M. , Hansson , H. C. and Laaksonen , A. 2000 . Hygroscopic Growth of Ultrafine Ammonium Sulphate Aerosol Measured Using an Ultrafine Tandem Differential Mobility Analyzer . J. Geophys. Res. , 105 ( D17 ) : 22231 – 22242 .
- Hand , J. L. , Ames , R. B. , Kreidenweis , S. M. , Day , D. E. and Malm , W. C. 2000 . Estimates of Particle Hygroscopicity During the Southeastern Aerosol and Visibility Study . J. Air Waste Manage. , 50 : 677 – 685 .
- Hand , J. L. and Kreidenweis , S. M. 2002 . A New Method for Retrieving Particle Refractive Index and Effective Density from Aerosol Size Distribution Data . Aerosol Sci. Tech. , 36 : 1012 – 1026 .
- Hansson , H. C. , Rood , M. J. , Koloutsou-Vakakis , S. , Hameri , K. , Orsini , D. and Wiedensohler , A. 1998 . NaCl Aerosol Particle Hygroscopicity Dependence on Mixing with Organic Compounds . J. Atmos. Chem. , 31 : 321 – 346 .
- Hegg , D. A. , Covert , D. S. , Crahan , K. K. , Jonsson , H. and Liu , Y. 2006 . Measurements of Aerosol Size-Resolved Hygroscopicity at Sub and Supermicron Sizes . Geophys. Res. Lett. , 33 doi:10.1029/2006GL026747
- Hegg , D. A. , Covert , D. S. , Jonsson , H. and Covert , P. A. 2007 . An Instrument for Measuring Size-Resolved Aerosol Hygroscopicity at Both Sub- and Super-Micron Sizes . Aerosol Sci. Tech , 41 : 873 – 883 .
- Hering , S. V. and McMurry , P. H. 1991 . Optical Counter Response to Monodisperse Atmospheric Aerosols . Atmos. Environ , 25 : 463 – 468 .
- Hinds , W. C. 1999 . Aerosol Technology: Properties, Behavior, and Measurement of Airborne Particles, , Second Edition , New York : John Wiley & Sons .
- Huang , X. F. , Hu , M. , He , L. Y. and Tang , X. Y. 2005 . Chemical Characterization of Water-Soluble Organic Acids in PM2.5 in Beijing, China . Atmos. Environ. , 39 : 2819 – 2827 .
- Huang , X. F. , Yu , J. Z. , He , L. Y. and Yuan , Z. B. 2006 . Water-Soluble Organic Carbon and Oxalate in Aerosols at a Coastal Urban Site in China: Size Distribution Characteristics, Sources, and Formation Mechanisms . J. Geophys. Res. , 111 : D22212 doi:10.1029/2006JD007408
- Kawamura , K. and Ikushima , K. 1993 . Seasonal-Changes in the Distribution of Dicarboxylic-Acids in the Urban Atmosphere . Environ. Sci. Technol. , 27 : 2227 – 2235 .
- Kawamura , K. and Sakaguchi , F. 1999 . Molecular Distributions of Water Soluble Dicarboxylic Acids in Marine Aerosols Over the Pacific Ocean Including Tropics . J. Geophys. Res. , 104 ( D3 ) : 3501 – 3509 .
- Kerminen , V. M. , Ojanen , C. , Pakkanen , T. , Hillamo , R. , Aurela , M. and Merilainen , J. 2000 . Low-Molecular-Weight Dicarboxylic Acids in an Urban and Rural Atmosphere . J. Aerosol Sci. , 31 : 349 – 362 .
- Khwaja , H. A. 1995 . Atmospheric Concentrations of Carboxylic-Acids and Related-Compounds at a Semiurban Site . Atmos. Environ. , 29 : 127 – 139 .
- Kim , J. , Yoon , S. C. , Jefferson , A. and Kim , S. W. 2006 . Aerosol Hygroscopic Properties During Asian Dust, Pollution, and Biomass Burning Episodes at Gosan, Korea in April 2001 . Atmos. Environ. , 40 : 1550 – 1560 .
- Knutson , E. O. and Whitby , K. T. 1975 . Aerosol Classification by Electric Mobility: Apparatus, Theory, and Applications . J. Aerosol Sci , 6 : 443 – 451 .
- Kotchenruther , R. A. and Hobbs , P. V. 1998 . Humidification Factors of Aerosols from Biomass Burning in Brazil . J. Geophys. Res. , 103 ( D24 ) : 32081 – 32089 .
- Kreisberg , N. M. , Stolzenburg , M. R. , Hering , S. V. , Dick , W. D. and McMurry , P. H. 2001 . A New Method for Measuring the Dependence of Particle Size Distributions on Relative Humidity, with Application to the Southeastern Aerosol and Visibility Study . J. Geophys. Res. , 106 ( D14 ) : 14935 – 14949 .
- Li , Z. D. , Williams , A. L. and Rood , M. J. 1998 . Influence of Soluble Surfactant Properties on the Activation of Aerosol Particles Containing Inorganic Solute . J. Atmos. Sci. , 55 : 1859 – 1866 .
- Lide , D. R. 2005 . CRC Handbook of Chemistry and Physics, , 86th Edition , Florida : CRC Press .
- Lightstone , J. M. , Onasch , T. B. , Imre , D. and Oatis , S. 2000 . Deliquescence, Efflorescence, and Water Activity in Ammonium Nitrate and Mixed Ammonium Nitrate/Succinic Acid Microparticles . J. Phys. Chem. A , 104 : 9337 – 9346 .
- Liu , B. Y. H. and Lee , K. W. 1975 . Aerosol Generator of High Stability . Am. Ind. Hyg. Assoc. J. , 36 : 861 – 865 .
- Liu , B. Y. H. , Pui , D. Y. H. , Whitby , K. T. , Kittelson , D. B. , Kousaka , Y. and Mckenzie , R. L. 1978 . Aerosol Mobility Chromatograph—New Detector for Sulfuric-Acid Aerosols . Atmos. Environ , 12 : 99 – 104 .
- Magi , B. I. and Hobbs , P. V. 2003 . Effects of Humidity on Aerosols in Southern Africa During the Biomass Burning Season . J. Geophys. Res. , 108 ( D13 ) doi:10.1029/2002JD002144
- Malm , W. C. , Day , D. E. , Kreidenweis , S. M. , Collett , J. L. , Carrico , C. , McMeeking , G. and Lee , T. 2005 . Hygroscopic Properties of an Organic-Laden Aerosol . Atmos. Environ. , 39 : 4969 – 4982 .
- Massling , A. , Wiedensohler , A. , Busch , B. , Neususs , C. , Quinn , P. , Bates , T. and Covert , D. 2003 . Hygroscopic Properties of Different Aerosol Types Over the Atlantic and Indian Oceans . Atmos. Chem. Phys. , 3 : 1377 – 1397 .
- Massling , A. , Leinert , S. , Wiedensohler , A. and Covert , D. 2007 . Hygroscopic Growth of Sub-Micrometer and One-Micrometer Aerosol Particles Measured During ACE–Asia . Atmos. Chem. Phys. , 7 : 3249 – 3259 .
- McMurry , P. H. and Stolzenburg , M. R. 1989 . On the Sensitivity of Particle-Size to Relative-Humidity for Los Angeles Aerosols . Atmos. Environ. , 23 : 497 – 507 .
- Peng , C. , Chan , M. N. and Chan , C. K. 2001 . The Hygroscopic Properties of Dicarboxylic and Multifunctional Acids: Measurements and UNIFAC Predictions . Environ. Sci. Technol. , 35 : 4495 – 4501 .
- Peng , C. G. and Chan , C. K. 2001 . The Water Cycles of Water-Soluble Organic Salts of Atmospheric Importance . Atmos. Environ. , 35 : 1183 – 1192 .
- Pilinis , C. , Seinfeld , J. H. and Grosjean , D. 1989 . Water-Content of Atmospheric Aerosols . Atmos. Environ. , 23 : 1601 – 1606 .
- Poore , M. W. 2000 . Oxalic Acid in PM2.5 Particulate Matter in California . J. Air Waste Manage. , 50 : 1874 – 1875 .
- Prenni , A. J. , DeMott , P. J. , Kreidenweis , S. M. , Sherman , D. E. , Russell , L. M. and Ming , Y. 2001 . The Effects of Low Molecular Weight Dicarboxylic Acids on Cloud Formation . J. Phys. Chem. A , 105 : 11240 – 11248 .
- Prenni , A. J. , De Mott , P. J. and Kreidenweis , S. M. 2003 . Water Uptake of Internally Mixed Particles Containing Ammonium Sulfate and Dicarboxylic Acids . Atmos. Environ. , 37 : 4243 – 4251 .
- Rader , D. J. and McMurry , P. H. 1986 . Application of the Tandem Differential Mobility Analyzer to Studies of Droplet Growth or Evaporation . J. Aerosol Sci. , 17 : 771 – 787 .
- Rood , M. J. , Larson , T. V. , Covert , D. S. and Ahlquist , N. C. 1985 . Measurement of Laboratory and Ambient Aerosols with Temperature and Humidity Controlled Nephelometry . Atmos. Environ , 19 : 1181 – 1190 .
- Saxena , P. , Hildemann , L. M. , Mcmurry , P. H. and Seinfeld , J. H. 1995 . Organics Alter Hygroscopic Behavior of Atmospheric Particles . J. Geophys. Res. , 100 ( D9 ) : 18755 – 18770 .
- Saxena , P. and Hildemann , L. M. 1996 . Water-Soluble Organics in Atmospheric Particles: A Critical Review of the Literature and Application of Thermodynamics to Identify Candidate Compounds . J. Atmos. Chem , 24 : 57 – 109 .
- Saxena , P. and Hildemann , L. M. 1997 . Water Absorption by Organics: Survey of Laboratory Evidence and Evaluation of UNIFAC for Estimating Water Activity . Environ. Sci. Technol. , 31 : 3318 – 3324 .
- Seinfeld , J. H. and Pandis , S. N. 2006 . Atmospheric Chemistry and Physics, , Second Edition , New York : Wiley-Interscience .
- Sekigawa , K. 1983 . Estimation of the Volume Fraction of Water-Soluble Material in Sub-Micron Aerosols in the Atmosphere . J. Meteorol. Soc. Jpn. , 61 : 359 – 367 .
- Sheridan , P. J. , Jefferson , A. and Ogren , J. A. 2002 . Spatial Variability of Submicrometer Aerosol Radiative Properties Over the Indian Ocean During INDOEX . J. Geophys. Res. , 107 ( D19 ) doi:10.1029/2000JD000166
- Snider , J. R. and Petters , M. D. 2007 . Optical Particle Counter Measurement of Marine Aerosol Hygroscopic Growth . Atmos. Chem. Phys. Disc. , 7 : 12381 – 12415 .
- Sorooshian , A. , Varutbangkul , V. , Brechtel , F. J. , Ervens , B. , Feingold , G. , Bahreini , R. , Murphy , S. M. , Holloway , J. S. , Atlas , E. L. , Buzorius , G. , Jonsson , H. , Flagan , R. C. and Seinfeld , J. H. 2006a . Oxalic Acid in Clear and Cloudy Atmospheres: Analysis of Data from International Consortium for Atmospheric Research on Transport and Transformation 2004 . J. Geophys. Res , 111 : D23S45 doi:10.1029/2005JD006880
- Sorooshian , A. , Brechtel , F. J. , Ma , Y. L. , Weber , R. J. , Corless , A. , Flagan , R. C. and Seinfeld , J. H. 2006b . Modeling and Characterization of a Particle-into-Liquid Sampler (PILS) . Aerosol Sci. Tech. , 40 : 396 – 409 .
- Sorooshian , A. , Ng , N. L. , Chan , A. W. H. , Feingold , G. , Flagan , R. C. and Seinfeld , J. H. 2007a . Particulate Organic Acids and Overall Water-Soluble Aerosol Composition Measurements from the 2006 Gulf of Mexico Atmospheric Composition and Climate Study (GoMACCS) . J. Geophys. Res , 112 : D13201 doi:10.1029/2007JD008537
- Sorooshian , A. , Lu , M.-L. , Brechtel , F. J. , Jonsson , H. , Feingold , G. , Flagan , R. C. and Seinfeld , J. H. 2007b . On the Source of Organic Acid Aerosol Layers Above Clouds . Environ. Sci. Technol. , 41 : 4647 – 4654 .
- Stolzenburg , M. , Kreisberg , N. and Hering , S. 1998 . Atmospheric Size Distributions Measured by Differential Mobility Optical Particle Size Spectrometry . Aerosol Sci. Tech. , 29 : 402 – 418 .
- Svenningsson , I. B. , Hansson , H. C. , Wiedensohler , A. , Ogren , J. A. , Noone , K. J. and Hallberg , A. 1992 . Hygroscopic Growth of Aerosol-Particles in the Po Valley . Tellus B , 44 : 556 – 569 .
- Svenningsson , B. , Hansson , H. C. , Wiedensohler , A. , Noone , K. , Ogren , J. , Hallberg , A. and Colvile , R. 1994 . Hygroscopic Growth of Aerosol-Particles and its Influence on Nucleation Scavenging In-Cloud Experimental Results from Kleiner–Feldberg . J. Atmos. Chem. , 19 : 129 – 152 .
- Svenningsson , B. , Hansson , H. C. , Martinsson , B. , Wiedensohler , A. , Swietlicki , E. , Cederfelt , S. I. , Wendisch , M. , Bower , K. N. , Choularton , T. W. and Colvile , R. N. 1997 . Cloud Droplet Nucleation Scavenging in Relation to the Size and Hygroscopic Behaviour of Aerosol Particles . Atmos. Environ. , 31 : 2463 – 2475 .
- Swietlicki , E. , Zhou , J. C. , Berg , O. H. , Martinsson , B. G. , Frank , G. , Cederfelt , S. I. , Dusek , U. , Berner , A. , Birmili , W. , Wiedensohler , A. , Yuskiewicz , B. and Bower , K. N. 1999 . A Closure Study of Sub-Micrometer Aerosol Particle Hygroscopic Behaviour . Atmos. Res. , 50 : 205 – 240 .
- Swietlicki , E. , Zhou , J. C. , Covert , D. S. , Hameri , K. , Busch , B. , Vakeva , M. , Dusek , U. , Berg , O. H. , Wiedensohler , A. , Aalto , P. , Makela , J. , Martinsson , B. G. , Papaspiropoulos , G. , Mentes , B. , Frank , G. and Stratmann , F. 2000 . Hygroscopic Properties of Aerosol Particles in the Northeastern Atlantic During ACE-2 . Tellus B , 52 : 201 – 227 .
- Tang , I. N. and Munkelwitz , H. R. 1993 . Composition and Temperature-Dependence of the Deliquescence Properties of Hygroscopic Aerosols . Atmos. Environ. , 27 : 467 – 473 .
- Tang , I. N. and Munkelwitz , H. R. 1994a . Water Activities, Densities, and Refractive-Indexes of Aqueous Sulfates and Sodium-Nitrate Droplets of Atmospheric Importance . J. Geophys. Res. , 99 : 18801 – 18808 .
- Tang , I. N. and Munkelwitz , H. R. 1994b . Aerosol Phase-Transformation and Growth in the Atmosphere . J. Appl. Meteorol. , 33 : 791 – 796 .
- Videen , G. , Pellegrino , P. , Ngo , D. , Videen , J. S. and Pinnick , R. G. 1997 . Light-Scattering Intensity Fluctuations in Microdroplets Containing Inclusions . Appl. Optics , 36 : 6115 – 6118 .
- Wang , J. , Flagan , R. C. and Seinfeld , J. H. 2003 . A Differential Mobility Analyzer (DMA) System for Submicron Aerosol Measurements at Ambient Relative Humidity . Aerosol Sci. Tech. , 37 : 46 – 52 .
- Weast , R. C. 1987 . CRC Handbook of Chemistry and Physics, , 68th Edition , Florida : CRC Press .
- Wise , M. E. , Surratt , J. D. , Curtis , D. B. , Shilling , J. E. and Tolbert , M. A. 2003 . Hygroscopic Growth of Ammonium Sulfate/Dicarboxylic Acids . J. Geophys. Res. , 108 ( D20 ) doi:10/1029/2003JD003775
- Yao , X. H. , Fang , M. , Chan , C. K. , Ho , K. F. and Lee , S. C. 2004 . Characterization of Dicarboxylic Acids in PM2.5 in Hong Kong . Atmos. Environ. , 38 : 963 – 970 .
- Yu , J. Z. , Huang , S. F. , Xu , J. H. and Hu , M. 2005 . When Aerosol Sulfate Goes Up, so Does Oxalate: Implication for the Formation Mechanisms of Oxalate . Environ. Sci. Technol. , 39 : 128 – 133 .
- Zhang , X. Q. , McMurry , P. H. , Hering , S. V. and Casuccio , G. S. 1993 . Mixing Characteristics and Water-Content of Submicron Aerosols Measured in Los Angeles and at the Grand Canyon . Atmos. Environ. , 27 : 1593 – 1607 .