Abstract
The nature of atmospheric aerosols is extremely complex and often requires advanced analytical tools for the determination of its physical and chemical properties. In particular, the interaction of particles with atmospheric water is a complex function of both particle size and composition. The ability of a particle to grow in a humid environment can be measured by humidity tandem differential mobility analyzing techniques (H-TDMA). In this article, we present a new development combining thermo-desorption and humidification aerosol conditioning in series that allows to measure changes in the hygroscopic behavior of aerosol at 90% relative humidity (RH) after conditioning of the particle by thermo-desorption to a temperature between 25°C and 300°C. The main feature of this system, named Volatility Hygroscopic—Tandem Differential Mobility Analyzer (VH-TDMA), is to allow for rapid (10 minutes) series of scans to control particle response to 1-thermal conditioning, 2- RH increase to 90% and 3—a combination of both thermal and RH conditioning. The VH-TDMA is, therefore, suited to investigate particle ageing through a simple coupling of H-TDMA and V-TDMA performances.
The aim of the present article is to describe the instrument design and to validate its performances by focusing on the measurement of hygroscopic behavior of pure inorganic particles such as sodium chloride or ammonium sulfate, as well as internally mixed organic-inorganic particles. Based on laboratory experiments and applications to natural aerosols, we show that the VH-TDMA system can be used to investigate the hygroscopic properties of the non-volatile fraction of ambient sub-micrometer aerosols in the range of 20 to 150 nm and the influence of the more volatile fraction of the particle on hygroscopic growth.
1. INTRODUCTION
The composition and structure of atmospheric particulate matter is not easily characterized by conventional methods although both are key parameters controlling the fate of particles in the atmospheric system. In particular, uptake of water vapor by atmospheric particles depends on size and chemical composition. Tandem Differential Mobility Analyzers (TDMA) provide indirect information on particle composition and size in almost real time and, hence, offer a powerful advantage over many other chemistry-based methods that require longer time-integration.
The hygroscopic properties of Aitken and accumulation mode particles have often been investigated using the Hygroscopic Tandem Differential Mobility Analyser (H-TDMA) (CitationRader and McMurry 1986; CitationStolzenburg 1988; CitationSvenningsson et al. 1992; CitationBerg et al. 1998). The basic principle of the H-TDMA technique is to monitor the growth of a particle of known diameter in a high RH environment (typically around 90% RH). In addition, for a given particle size, differential growth of particles is used to characterize the mixing state of the aerosol population. Internal mixing is characterized by homogeneous growth while externally mixed particle population is evidenced by the presence of multiple hygroscopic modes.
The hygroscopic properties of atmospheric particles containing organic and inorganic compounds have extensively been investigated by the TDMA technique in both laboratory and field experiments (CitationSaxena et al. 1996; CitationCruz and Pandis 1998; CitationHansson et al. 1998). Elevated hygroscopic growth factors (HGF) (i.e., >1.6) are typically found in the marine or coastal atmosphere due to the presence of sea-salt and sulphate aerosols (CitationBerg et al. 1998; CitationJohnson et al. 2004; CitationPutaud et al. 2004; CitationRaes et al. 2000; Swietlicki et al. 1999; CitationBower et al. 2000), while reduced HGF (i.e., <1.1) are found in the urban areas (Massling et al. 2005; Rissler et al. 2005). In most sites, however, H-TDMA studies indicate the simultaneous presence of both a less-hygroscopic and a more hygroscopic particle class, consisting of particles with less and more soluble material, respectively (CitationSvenningsson et al. 1992, Citation1994; CitationZhang et al. 1993; CitationCovert and Heintzenberg 1993; CitationPitchford and McMurry 1994; Kerminem 1997; CitationSwietlicki et al. 2000). Nevertheless, the proportion between more- and less-hygroscopic modes remains variable (CitationKandler and Shütz 2007).
The thermal properties of particles are also related to their chemical composition (CitationO'Dowd et al. 1992; CitationWahlin et al. 2000; CitationPaulsen et al. 2006; CitationPhilippin et al. 2003; Rose et al. 2006). Analyses of particle thermal properties are based on the fact that most components present in aerosols become volatile at a characteristic temperature (CitationOrsini et al. 1998; CitationBurtscher et al. 2001; CitationBrooks et al. 2002). When the temperature increases, certain chemical constituents in the aerosol volatilizes, resulting in a change in both number concentration (when a particle is totally volatilized) and size. The temperature at which complete or partial volatilization takes place allows estimating the chemical composition and the degree of mixing within the aerosol population (CitationClarke 1993; CitationJennings et al. 1997; CitationOrsini et al. 1998; CitationPhilippin et al., 2003). The response of atmospheric aerosols to thermal desorption has often been investigated using Volatility Tandem Differential Mobility Analyser (V-TDMA) techniques (CitationOrsini et al. 1998; CitationPhilippin et al. 2003; CitationVillani et al. 2007). Due to difficulties in relating volatilization temperatures to chemical composition and due to the complex nature of atmospheric particles, the use of V-TDMAs for investigating natural aerosols remains problematic.
While V-TDMA techniques are suited to quantify the non-volatile fraction of aerosol particles (often using a reference thermo-desorption temperature of 300°C), they can not distinguish between elemental carbon and low volatile organic species in the non-volatile residue. On the other hand, hygroscopic growth measurements alone can not be used for investigating the chemical structure of the particles which is particularly linked to their formation process (i.e., condensation onto refractory material or inorganic salt and direct homogeneous nucleation).
The aim of this work is to develop and validate a new TDMA system coupling features of both V- and H-TDMAs. The system, hence named Volatility Hygroscopic Tandem Differential Mobility Analyser (VH-TDMA), has primarily been used in this study to investigate the role of semi-volatile particle species condensed on their surface to limit or increase particle hygroscopic growth. A similar development has recently been presented by CitationJohnson et al. (2004) with different operating procedures but similar capacities. However, in this study, the VH-TDMA was applied to investigation of gentle thermodesorption effect on hygroscopic growth while CitationJohnson et al. (2004) rather focused on the relationship between thermal and hygroscopic properties for a large range of temperatures. In this article, we will describe how VH-TDMAs offer a cost-effective solution to H-TDMA and V-TDMA features in addition to the unique possibility to investigate hygroscopic properties of thermo-desorbed particles. In that respect, VH-TDMAs are more effective than parallel H-TDMA and V-TDMA instruments to investigate changing particle properties during aerosol ageing.
2. EXPERIMENTAL SECTION
2.1. Instrument Design
The VH-TDMA features the properties of both the V-TDMA and the H-TDMA system. The instrument is capable of rapid switching to investigate the behavior of particle under conditioning at constant temperature (i.e., V-TDMA), at constant humidity (i.e., H-TDMA) or at simultaneous constant temperature/humidity (i.e., VH-TDMA). This new system is especially designed to perform automatic measurements with minimal needs for maintenance, high reliability and stability of flow rates, as well as precise control of humidity and temperature. The design of the VH-TDMA system is shown in .
The system is based on a usual TDMA set-up: it consists of a bipolar diffusion charger, a fixed voltage long column DMA1 (i.e., custom-made DMA, inner radius = 0.937 cm, outer radius = 1.96 cm, length = 44 cm) that is used to select a narrow size range of ambient aerosol which is subsequently counted with a TSI-3010 condensation particle counter (CPC1). A second long column DMA2 (identical to DMA1) followed by a second TSI-3010 CPC (CPC2) operates as a mobility size spectrometer to measure the modified size distribution.
2.2. VH-TDMA Technical Specifications
2.2.1. Sheath Airflow Arrangement
A re-circulating sheath flow system is used in both DMAs. For the present instrument, two membrane pumps are used operating with Teflon membranes avoiding artifacts by outgassing material. DMA1 is operated at flow rates of 2.0/10 lmin–1 (sample flow/sheath air flow) and the same aerosol/sheath flow ratio (1.0/5 lmin–1) is used in DMA2. The sheath air flows are controlled by mass-flow controllers/meters located in the excess air outlet of the DMAs (Red path in ). Total particle filters are used in both sheath lines and excess-flow lines to ensure that clean, particle-free sheath air is injected at the bottom of the DMAs and to protect the mass-flow meters and needle valves. The volumetric sheath flow is calculated by measuring the absolute pressure and temperature of the sheath flow in each DMA loop. These pressures and temperatures are also used to calculate and correct the particle mobility in each DMA. It should be noted that, under standard operation of the DMAs, the diffusional broadening of the transfer function is limited. The operating voltage under which diffusional broadening may be significant when aerosol/sheath flow ratio of 1.0/5 lmin–1 is used in DMA2 is 20V (CitationFlagan 1999). This voltage corresponds to a size of 14 nm which is outside of the standard use of the VH-TDMA (20 nm).
The aerosol sample flow at the outlet of the DMAs is set by the CPC 3010 critical orifice with a nominal flow rate of 1.0 lmin–1. The aerosol flow at the inlet of the DMAs is also measured and recorded by measuring the pressure drop over calibrated capillaries. This feature increases reliability and stability of the system with an automatic flow regulation that does not require any assistance. Aerosol flow and excess air flow meters for both DMAs are calibrated against a reference volumetric flow meter (0–10 lmin–1 ±0.4% F.S.). During each measurement scan the flow standard deviation are less than 1%. The re-circulating sheath flow loop also contains a Silica Gel tube dryer capable of maintaining the RH below 20%. DMA1 is continuously operated at low RH (i.e., dry DMA) to select the monodisperse aerosol, while for DMA2 the sheath air can be switched from dry to humidified air. Additional technical details concerning the humidifying unit are provided in Section 2.2.3. In both loops, the sheath flow is also introduced into a heat exchanger placed at the pump outlet to maintain uniform temperature in the DMA.
Sampled aerosol particles flow through the conditioning units (i.e., the humidifier and/or thermo-desorber) by using several in-line electronically actuated three-way valves throughout the system (Green path in ). To reduce in-line losses, valves with an inner orifice diameter of 4 mm are used. The different three-way valve positions permits four possible TDMA modes:
1. | TDMA mode The TDMA mode is the basic operation procedure of the instrument. Once the solenoid valves (SV1 to SV6 in ) are turned on, both thermo-denuder and aerosol pre-humidifier are directed through bypass lines ByP-1 and ByP-2 (see black dashed lines in ), respectively. The DMA2 sheath flow flows through a silica gel tube dryer to keep RH < 20%. By operating both DMAs at a constant, low RH, it is possible to compare the offset of the two DMAs in a range of particle sizes immediately before and after field or laboratory campaigns to take into account the diameter shift measured by the second DMA. This “Dry scan” is also used to check the DMA transfer function. | ||||
2. | H-TDMA mode Investigation of particle hygroscopic growth is performed setting the VH-TDMA in H-TDMA operational mode. The “wet scan” is actuated when the solenoid valves SV1 and SV2 are turned on, while SV3 to SV6 are switched off. Prior to entering the pre-humidifier, the aerosol sample flow passes through a stainless-steel line (i.e., ByP-1), that has the same length and dimension of the volatilization tube. This feature ensures that the diffusion losses are the same, regardless of which flow path the particles follow. During normal operations, the RH of the second DMA is monitored by sensors RH4 and RH5, placed in the sheath and excess flow lines, respectively, while the aerosol flow RH is measured by sensor RH3. RH5 is considered to be most representative for the effective overall relative humidity in DMA2 and is used for both regulating the wet DMA RH (i.e., RH = 90%) and for all plots and calculations involving RH. Before entering the second DMA, the RH-conditioned monodisperse aerosol flow is introduced into a small reaction chamber (V = 125 cm3) with a residence time of 7 s at a flow rate of 1.0 lmin–1. This residence time is much higher than the estimated time to achieve water vapor equilibrium for pure submicrometer salts at 90% RH and T = 25°C (100 milliseconds) (CitationKerminen 1997). However, increasing the exposure time of particles to high RH may limit the influence of water inhibiting compounds in the particulate phase (e.g., organic films, CitationSjogren et al. 2007) that reduce the water uptake across the particle surface and possibly increase the equilibrium time. The mean residence time of the aerosol in the pre-humidifier and in the subsequent line leading to DMA2 is about 6 s. In the DMA-CPC, the particles stay for another 6 s before being finally counted, leading to an overall conditioning time of about 12 s. | ||||
3. | V-TDMA mode Investigation of particle volatile properties is performed setting the VH-TDMA in V-TDMA operational mode. When the solenoid valves SV1 and SV2 are switched off, while SV3 to SV6 are on, the sample aerosol flow is conditioned to a well-defined temperature in the volatilization tube (“OVEN” in ) and the residual mobility distribution is then measured with DMA2 scanning in dry conditions (i.e., RH < 20%). It is important to note that, for the specific conditions of a volatility scan, it is extremely useful to measure the particle concentration downstream of each of the DMAs using the two CPCs to determine the number fraction of particles that are completely volatilized. It should be noted that V-TDMA scans are performed at a fixed temperature (ranging from 90°C to 200°C for the experiments described in this article). | ||||
4. | VH-TDMA mode Coupling features of V and H-TDMA modes is obtained using the VH-TDMA operation mode. When all solenoid valves (i.e., SV1 to SV6) are switched off, the aerosol sample flow is heated to the same volatility scan temperature as for the V-TDMA subsystem before entering the pre-humidifier (“PPT” in ). DMA2 scans in wet conditions (i.e., the set point RH is identical to that of the humidity scan) and measures the hygroscopic behavior of the aerosol after thermal conditioning. |
2.2.2. RH/T Measurements
Five capacitance RH sensors (model Rotronic, HygroClip SC05) are used in-line throughout the instrument (see ) to monitor the temperature and RH and to allow fast RH regulation.
A first probe is placed at the instrument inlet to monitor the sample flow RH (RH1), a second one is used to measure the dry DMA sheath flow loop RH, placed on the analyzer excess flow (RH2). Three other sensors are employed to check the DMA2 RH: one controls the aerosol sample flow inlet (RH3) and two others control the DMA sheath (RH4) and excess flow (RH5), respectively. The sensors used for the DMA2 RH regulation are placed in the excess air line (RH5) and the aerosol inlet line (RH3), respectively.
During normal humid scan operations, the RH of the aerosol flow in the aerosol pre-humidifier is set to 88–89%, while the RH in the DMA excess flow is maintained at 90%. Up to a RH of 90%, the relative humidity of the sheath flow (RH4) and excess flow (RH5) of DMA2 agree to within 1.5%. For RH > 90%, RH5 is lower than RH4 by up to 3%, probably due to small temperature gradients in the system.
The RH probes are oriented parallel to the air flow and are located within a custom-made housing to reduce in-line aerosol particle losses due to turbulent diffusion. The probes have an accuracy of ±1.5% for RHs up to 90% and ±0.3°C for temperatures between –40°C and 80°C. Above 90% RH, the accuracy deteriorates to ±2%. The humidity probes are calibrated against a dew point meter (model DewMaster, EdgeTech). The relative humidity (in the range 10–97% RH) is calculated by measuring the flow temperature with a calibrated temperature probe (Pt100, precision <±0.06°C), keeping the humidity sensors in a thermostatically stable environment. During normal operations, the values of the five RH sensors of the VH-TDMA are corrected using a linear regression fitting through the calibration measurement points.
2.2.3. Humidity Control System
In our system, the RH of the DMA2 sheath flow and the aerosol flow are adjusted separately using independent humidifying circuits. In both cases, the flows are humidified using a vertically oriented Nafion membrane (i.e., PermaPure single-tube MD-110-12S-4 for the aerosol flow, 1 lmin–1, and PermaPure multi-tube PH-60T-12SS for the DMA2 sheath flow, 5 lmin–1). Regulation of the humidity is obtained using saturated air counter-flow in the Nafion tube. The moisture can diffuse from this secondary flow through the membrane into the flow stream. The secondary saturated airflow is provided by a system of controlled evaporation mixing (CEM), shown in , that allows to mix particle-free dry air (up to 16.0 lmin–1) with an Ultra-pure water flow (up to 50 gh–1) that evaporates when injected inside the three-way heating valve (up to 100°C). The CEM temperature and water flow set point are regulated as a function of the atmospheric dew point temperature and the desired saturated air flow rate.
The aerosol and sheath flows humidification is independently regulated using custom-made PID software which compares the set point RHs with those measured by the capacitive sensors (i.e., RH3 for the aerosol flow, and RH5 for the excess flow). Two electronically actuated solenoid three-way valves allow switching to the saturated flows injections in the Nafion humidifiers (about 2.0 lmin–1 in the multi-tube membrane and 4.0 lmin–1 in the single-tube membrane) with an adapted PID.
2.2.4. Temperature Control
The determination of RH in DMA2 is not trivial due to temperature gradients between inlet and outlet of the DMA. A temperature difference of only 0.5°C leads to a change in RH up to 3–4% at a RH close to 90%. Hence, it is essential that the wet DMA is kept at a constant temperature, independent of the ambient temperature fluctuations to minimize RH gradients. This stabilization is achieved by enclosing DMA2 and the humidity conditioning section within an insulated, temperature controlled volume (∼70 l). The temperature is measured in the center of the volume with a temperature probe Pt100. The thermostatically stable environment is maintained by two thermoelectric air coolers (TEC, Peltier units 50+50 W). An additional long copper heat exchanger is placed in the sheath line, inside the thermostatic volume to ensure the equilibrium between flow temperature and DMA temperature. All heat sources, such as pumps or power supplies are kept outside the thermostatic volume.
An example of the stability of the DMA2 temperatures over a 24-h time period is shown in . The temperature sensors are placed at the DMA2 aerosol inlet, in the sheath and in the excess flow. All measured temperatures are within 0.3°C of each other; we can, therefore, assume that the three temperatures are representatives of the “DMA2 temperature” and are, thus, independent of ambient temperature fluctuations (of typically <1.5°C). The calculated standard deviations of the sensor temperatures are <0.05°C. During measurements over a 24-h time period, a typical standard deviation was 0.01 for the aerosol flow RH, set to 89% (RH3) and 0.08 for the excess flow RH, set to 90% (RH5), respectively. The slight oscillation of the temperature measurements that can be seen in (less than ±0.1°C) is due to the aerosol flow RH regulation system.
2.2.5. Aerosol Thermo-desorber Conception
The monodisperse aerosol is heated to a predefined temperature in a custom-made thermo-denuder. The details of the thermo-desorbing unit concerning its design and performances are described in CitationVillani et al. (2007). Although the unit was developed to be used over a large temperature range (up to 1000°C), the conditioning temperatures are limited in this study to T < 120°C.
2.2.6. Measurement Control and Data Acquisition
The VH-TDMA is controlled by a program written in the graphical programming language LabVIEW (National Instruments). The main program consists of 100 subroutines of which 40 subroutines are created for the VH-TDMA application and the rest is provided by the LabVIEW standard libraries. The data acquisition is achieved via two National Instruments data acquisition (DAQ) boards (National Instrument Corporation) and a custom-designed electronic conditioning box that measures the signals arriving at the specified board channels.
2.2.7. Operating Procedures
A complete characterization of aerosol particles at a specific dry diameter (DRY Dp) selected by DMA1 consists in an alternate sequence of three scans: (1) H-TDMA, (2) VH-TDMA, and (3) V-TDMA. It should be noted that, prior to neutralization and initial size classification in DMA1, the aerosol is dried to a relative humidity <20% in a diffusion drier tube. Furthermore, the humidifying unit is kept in a stand-by mode when it is not being used during volatility scans as well as for the thermo-desorbing unit during humidity scans. This enables rapid switching from one scan type to the other, thus, limiting the delays due to equilibrium conditions. DMA2 operates a stepping-voltage method (i.e., similar to a DMPS).
Hygroscopic growth factors (HGF) are measured as follows:
Volatility “growth” factors (VGF) are defined as the ratio between the particle diameter at RH = 10%, T > T amb (D p 10,T °) and the dry particle diameter at RH = 10% and ambient temperature (D 10,Tamb p ), as follows:
Note that no shape factor correction is applied after thermal-desorption of the particle and we therefore do not account for variability of water penetration inside particles (Weingartner et al. 1996). The operating procedures differ from those described by CitationJohnson et al. (2004). The Johnson VH-TDMA consists of 3 DMAs: one (DMA1) produces a flow of charged, size-segregated aerosol particles in dry conditions (i.e., RH < 20%) which is split into two equal sub-flows. The first sub-flow is directed to the V-TDMA subsystem (i.e., DMA2 and CPC1) where particles are examined for changes in diameter after thermal treatment. The second one is directed towards the H-TDMA subsystem (i.e., DMA2 and CPC2), where particles are examined in terms of their hygroscopic behavior. A bypass valve from the thermo-desorbing unit to the H-TDMA subsystem allows for VH-TDMA scans. The main difference between both systems is that in our system the conditioning temperature is kept constant. In the Johnson system, on the other hand, the scans are repeated at successively higher conditioning temperatures. Their system is being used for analyzing the hygroscopic behavior of monodispersed particles as a function of temperature.
3. VALIDATION OF VH-TDMA PERFORMANCES
3.1. Aerosol Penetration Efficiency
The aerosol penetration efficiency through the instrument is determined experimentally in order to correct the measured mobility distributions for the size-dependent losses in the DMAs, the valves, and connection tubing. The VH-TDMA system, therefore, requires a quantification of particle transport efficiency as a function of size for the four flow patterns (i.e., TDMA, V-TDMA, H-TDMA, and VH-TDMA).
The evaluation of particle losses was performed using sodium chloride (NaCl) aerosol particles generated in the laboratory with an atomizer (TSI Model 3670) filled with a solution of Ultra Pure Water (ELGA) and NaCl salt. NaCl is well suited for calibrating because it is thermally stable for a wide range of particle diameters. After dispersion, polydispersed NaCl aerosol particles are further mixed with sheath air for drying and are diluted in a mixing chamber (100 l) to ensure a stable particle concentration. Since particle diffusion is size dependent, the penetration measurements are carried out for a range of particle diameters (10 < D p < 100 nm) at ambient temperatures and RH < 20% and for the four flow patterns of the VH-TDMA. The size-dependent transport efficiency η(Ti,Dp) is calculated by comparing the CPC1 and CPC2 concentrations. illustrates the results of the transport efficiency measurements. As particle losses at ambient temperatures in the TDMA and the V-TDMA path are similar, only one curve named “DRY path” is represented. Likewise, the curve named “WET path” represents the particle penetration through the H-TDMA and VH-TDMA tubes at ambient temperature and RH < 20%. We can see that for the dry scans η=100%; for D p = 90 nm and D50%; p = 21 nm, while for the wet scans η= 96% for D p = 90 nm and D50%; p = 25 nm. As shown in , the particle losses are greater in the “WET path” because of the utilized aerosol Nafion humidifier, a 30 cm tube membrane with inner diameter of 2.8 mm.
3.2. H-TDMA Calibration
The calculation of hygroscopic growth requires a relative calibration of DMA2 with respect to DMA1. This calibration is the standard procedure performed for TDMA measurements. Ammonium sulphate ((NH4)2SO4) particles are produced and five specific particle sizes are selected by DMA1 in the range of 15 to 100 nm. The selected size is then measured by DMA2 at RH < 20%. The results are presented in and show that DMA2 underestimates the selected diameter of DMA1 by 1 to 5%, depending on particle size.
TABLE 1 Results of the DMA2 shift calibration using Ammonium Sulphate particles
The shift deviation is higher at smaller diameters either due to an offset or an error in the lower voltage range of the DMA power supplies, or to operating the DMAs in the low-voltage, diffusion dominated regime for particles smaller than 50 nm. The DMA2 shift is constant as long as the same DMAs and aerosol tubing are used. It is nevertheless checked before and after each operation in the field. The relationship between the DMA1 and DMA2 diameters is then fitted by linear regression to correct all growth factors measured with the H-TDMA.
To verify the proper operation of the H-TDMA system, humidograms of various laboratory-generated aerosols are established and then compared with theoretical calculations according to the standardized method for H-TDMA calibration. and 5b show the humidograms of laboratory-generated (NH4)2SO4and NaCl particles. DMA1 was operated at a fixed voltage to select 100 nm particles (DRY D p ). The measurements were performed for RHs between 8 ± 1% and 90 ± 1.5%.
FIG. 5 (a) Humidogram of laboratory-generated (NH4)2SO4 particles compared with theory from CitationTopping (2005a). (b) Humidogram of laboratory-generated NaCl particles compared with theory from CitationTopping (2005a).
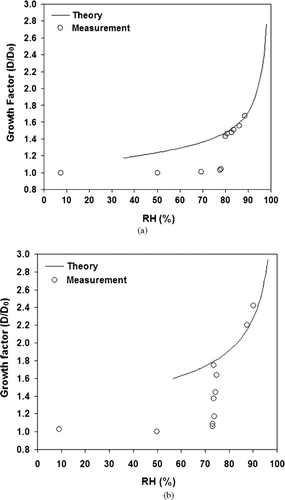
In general, there is good agreement between the observed hygroscopic growth factors and those found in literature. The RH uncertainty in the second DMA is due to the uncertainty of the dew point mirror used to calibrate the RH sensors and is less than ±1.5% at 90% RH. The uncertainty of the growth factor derived by the uncertainty in RH is less than ±0.05 at 90% RH. The measured and calculated growth factors agree within the uncertainty range of the system and is about ±0.05. The H-TDMA sub-system of the VH-TDMA is, therefore, well adapted to provide accurate measurements of particle hygroscopic growth.
3.3. VH-TDMA Calibration
The validation of the VH-TDMA performances was carried out following the procedure of CitationJohnson et al. (2004) by using internally mixed particles composed of NaCl and DEHS (Di-2-Ethylhexyl-Sebacat). These two compounds have different growth factors at 90% RH (2.35 and 1.05, respectively) and different volatilization temperatures (650°C and 120°C, respectively) for 100 nm particles. Internal mixing was produced by coating pure NaCl particles with DEHS with a PALAS MAG-2010 Monodisperse Aerosol Generator. Liquid DEHS evaporates in the generator at 70°C (i.e., saturator temperature) and condenses on the surface of the NaCl particle. The resulting particle size at the peak concentration, thus, shifted to larger diameters (i.e., from 50 nm for pure NaCl to 115 nm for NaCl with DEHS exposure), indicating a DEHS coating on the NaCl core. The large increase in size and the absence of small particles after DEHS exposure indicates that an external mixture of NaCl and DEHS is limited or even unlikely during such process. DEHS homogeneous nucleation does not take place as the low temperature gradient between saturator and reheater of the MAG generator prevents the formation of pure DEHS particles.
We performed a complete VH-TDMA scan of the NaCl internally mixed particles. The temperature dependence of the humid and dry particles as well as the corresponding VHGF for 100 nm particles is shown in .
FIG. 6 Particle diameter after volatilization (D v ), diameter after subsequent hygroscopic growth at 90% RH (D vh ) and diameter hygroscopic growth factor (VHGF = D vh /D v , black line) vs. thermo-desorber temperature for NaCl-seeded DEHS 00 nm aerosol particles.
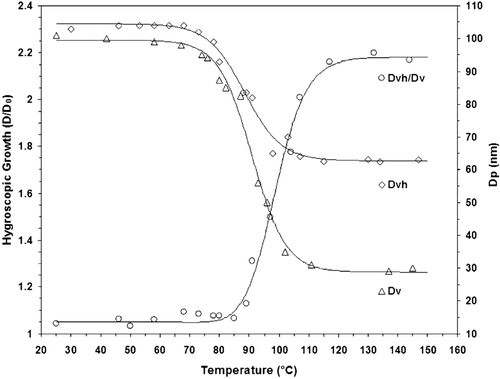
The DEHS component begins to evaporate at 70°C and is completely removed at 120°C. The diameter HGF begins to increase as the DEHS component evaporates and reaches a value of 2.18 when all of the DEHS is removed. The NaCl seeding particles diameter is, then, about 30 nm. The value of this growth factor agrees well with the theoretical value of 2.23 which was calculated using the model by Hameri et al. (2001) for 30 nm NaCl particles at 90% RH. The measured HGF agrees with that of the original NaCl particle once the DEHS coating is removed by thermo-desorption.
This finding is contradictory with previous studies (CitationKanakidou et al. 2005; CitationSvenningsonn et al. 2005) showing that the water uptake of an aerosol mixture can be predicted based on the knowledge of the hygroscopic growth of its single components—the so-called Zdanovskii-Stokes-Robinson (ZSR) theory (CitationStokes and Robinson 1966). In that case, the HGF of the original DEHS-NaCl mixture is very close to that of pure DEHS. One reason for the discrepancy with the ZSR theory might be the presence of DEHS on the surface of the NaCl core, which controls the interaction of the particle with water vapor.
4. FIRST RESULTS WITH NATURAL AEROSOL PARTICLES
The VH-TDMA was tested in the field during several research campaigns. In this paper, our investigation is limited to several selected case studies within the different campaigns. The objectives are to illustrate the potential of the VH-TDMA for better understanding the factors and mechanisms controlling hygroscopic growth.
The following interpretation of the VH-TDMA results are based on the hypothesis that any change in the HGF measured after thermo-desorption is due to preferential evaporation of one of the aerosol components, as for the NaCl/DEHS validation case. Nevertheless, it should be taken into consideration that other processes such as chemical alteration of the aerosol components might explain some of our results. We will present in the following representative examples of the different features found with VH-TDMA. It should be noted that each of the selected case studies have been observed regularly during the different field campaigns and do not result from single isolated cases.
4.1. Case Study 1: Thermo-Desorption has no Effect on HGF ()
The first case corresponds to measurements performed during a field campaign that took place at Castelporziano in May 2007, located on the western coast of Italy at 30 km SW of Rome (Italy). The measurement was done on 16/05 during the night. The selected particle size was 85 nm corresponding to one of the modes of the number size distribution. The V-TDMA scan at 110°C reduces the particle diameter down to 78 nm (8% reduction). The H-TDMA scan reveals 2 distinct hygroscopic modes with HGF of 1.03 ± 0.05 (less hygroscopic mode I) and 1.4 ± 0.05 (more hygroscopic mode II), respectively. The number fractions of the two modes are approximately 40% for mode I and 60% for mode II. The hygroscopicity and number fraction of both modes are not altered by the thermal treatment (mode I with HGF = 1.02 ± 0.05 and mode II with HGF = 1.41 ± 0.05). We are aware that a very accurate assessment of the HGF changes should also account for the Kelvin effect, that is, lower growth factor for smaller particle diameter. For (NH4)2SO4 particles, reducing particle diameter from 85 nm to 78 nm results in a HGF change of approximately 0.1 that cannot be resolved in our set-up.
FIG. 7 Representative case studies of VH-TDMA measurements. The “Dry” curve (dotted black line) represent the TDMA scan, the “V” curve (gray line) correspond to the V-TDMA scan at the indicated temperature. The “H” curve (black line) is the H-TDMA scan and the “VH” curve (dotted gray line) the VH-TDMA scan. For (a) Castelporziano, (b) Leipiz, (c) Cezeaux, and (d) Puy de Dome.
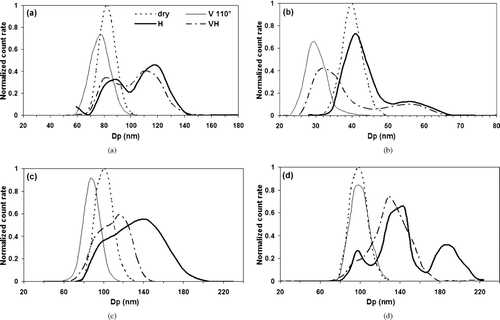
4.2. Case Study 2: Thermo-desorption Enhances HGF ()
Case study 2 is selected from a field campaign performed at the campus of the s done ine) the VH-TDMA scan. Institute for Tropospheric Research (IFT) in Leipzig (Germany). The site is located a few km NE of the downtown area and represents urban background aerosols. Aitken mode particle are transformed during transport from central Leipzig to the IFT. The present measurement has been done on 30/10/06 during the day-time. The selected size is 40 nm. The V-TDMA scan at 110°C produces a diameter reduction to 30 nm (25% reduction). The hygroscopic growth of 40 nm particles at 90% RH reveals the presence of two hygroscopic modes (a less-hygroscopic mode I at 1.1 ± 0.05 and a more-hygroscopic mode II at 1.4 ± 0.05). The proportion of the two modes is approximately 80% for mode I and 20% for mode II. Following thermal conditioning at 110°C, the less-hygroscopic mode does not appear to undergo any variation (HGF = 1.1) while the more hygroscopic mode continues to grow to 56 nm (from an original diameter of 30 nm), thus, resulting in a growth factor of 1.85 ± 0.05. This corresponds to a 30% increase with respect to the ambient HGF. Therefore, a removal of non-hygroscopic material, most likely anthropogenic VOCs, by evaporation at 110°C leads to a significant HGF increase.
4.3. Case Study 3: Thermo-desorption Limits HGF ()
Reduction of the hygroscopic growth is the least frequent of all cases although we have observed such examples during several campaigns. The measurement shown here was selected from a field campaign performed on the Cezeaux Campus, a suburban site located a few kilometers SE of the Clermont-Ferrand (France) city center. The hygroscopic growth of 100 nm particles at 90%RH reveals the presence of a less-hygroscopic mode at 1.0 ± 0.05 and a more-hygroscopic mode at 1.43 ± 0.05. The thermal treatment at 70°C reduces the particle diameter down to 91 nm (9% reduction). The more hygroscopic mode grows to 120 nm from its original diameter of 91 nm (i.e., HGF = 1.31 ± 0.05), corresponding to a 10% decrease with respect to the ambient HGF. On the contrary, the HGF of the less hygroscopic mode increases to 1.07 ± 0.05 for identical thermal treatment.
4.4. Case Study 4: Thermo-Desorption Suppresses one Hygroscopic Mode ()
The last case corresponds to measurements performed on the top of Puy de Dôme, Central France, in October 2005. At this period of year, the site lies in the free troposphere. Measurements were performed in an air mass originating from SW on 25/10. The size distribution shows 2 distinct modes around 30 and 100 nm, respectively. Here, we present a VH-TDMA analysis of 100 nm particles at a thermo-desorption temperature of 70°C. The V-TDMA scan reduces the particle diameter down to 97 nm. The H-TDMA scan reveals 3 distinct hygroscopic modes at 1.05 ± 0.05 (less hygroscopic mode I), 1.45 ± 0.05 (more hygroscopic mode II), and 1.9 ± 0.05 (very hygroscopic mode III). After thermal treatment, the hygroscopic properties of the particle are considerably modified resulting in a suppression of the very hygroscopic mode III and a more pronounced more-hygroscopic mode II at HGF = 1.43 ± 0.05. On the contrary, the less hygroscopic mode is not altered by thermal treatment. In that case, it appears that the hygroscopic properties of the mode III particles are determined by the presence of a very hygroscopic substance on the particle surface which is volatile at 70°C. A primary candidate is HNO3 that was found at elevated concentrations in both clouds and aerosols on the Puy de Dôme (CitationMarinoni et al. 2004; CitationSellegri et al. 2003). Nitric acid is very volatile and is often found attached to the particle surface. Thermo-desorption, therefore, evaporates the surface layer and reduces the HGF of the mode III particles down to the more hygroscopic mode II.
The four examples provided here show that gentle thermo-desorption potentially has a significant impact on the ability of a particle to grow at a given RH. It appears that under specific circumstances the loss of volatile material from the particle surface may significantly increase or decrease the hygroscopic growth, depending on the nature of the condensed material. Note that we cannot exclude at this stage that gentle thermo-desorption modifies the chemical nature of the organic fraction of the aerosol, thus leading to a change of hygroscopic properties. A more detailed analysis of the conditions during which hygroscopic properties are controlled by such surface effect is beyond the scope of the present article and is discussed in CitationSellegri et al. (2008) and CitationVillani et al. (2007).
5. SUMMARY AND CONCLUSIONS
This article presents and describes the original design of a unique VH-TDMA that has the capability of measuring the hygroscopic behavior of internally mixed particles and their non-volatile residues. The VH-TDMA comprises four subsystems (TDMA, H-TDMA, V-TDMA, VH-TDMA). The performance of each system was validated separately. Particle losses in the system have been quantified and accounted for in the retrieval procedures. We can show that the H-TDMA properly retrieves HGFs which are close to the theoretical expectations. Furthermore, with the VH-TDMA we have measured the change in hygroscopic growth of internally mixed particles during thermal removal of their more volatile components. Our results indicate that the thermo-desorption process is very efficient to remove adsorbed semi-volatile material from the particle surface. We can demonstrate that, once the volatilization temperature of the semi-volatile component is reached, the measured HGF is consistent with the HGF of the core-forming component of the internally mixed particle.
The results from field measurements show that the VH-TDMA technique is very useful for investigating particle formation processes and for differentiating seeded heterogeneous nucleation and homogenous nucleation. This distinction is possible since the VH-TDMA allows distinguishing in particles consisting of several species between the components that have similar volatility but differing hygroscopic behavior. The choice of the thermo-desorbing temperature during thermal treatment permits to investigate the role of the different chemical compounds in the process of particle hygroscopic growth. Therefore, the VH-TDMA is in investigations of the volume fraction of the semi-volatile particle constituents of the submicrometer aerosol and in the study of the hygroscopic behavior of the particulate residues for different volatilization temperatures.
Acknowledgments
P. Villani acknowledges financial support from CNRS and region Auvergne under the BDI program. The authors would like to acknowledge the financial support of the CNRS under the LEFE-CHAT program and ADEME and Ministère de l'Ecologie et du Développement Durable under the PRIMEQUAL program. Measurements in Castelporziano have been made possible through the EU-NoE-ACCENT program. P. Villani acknowledges the Marie Curie EU-Project AEROTOOLS (EVK2-CT-2002-57005) for the stay at the training site at the WMO WCC Leipzig.
This is a contribution from the EUSAAR FP6 Integrated Infrastructure Initiative.
REFERENCES
- Andrews , E. and Larson , S. M. 1993 . Effect of Surfactant Layers on the Size Changes of Aerosol Particles as a Function of Relative Humidity . Environ. Sci. Technol. , 27 : 857 – 865 .
- Berg , O. , Svietlicki , E. , Martinsson , B. , Wendish , M. , Yuskevic , B. , Heintzenberg , G. , Laj , P. , Ricci , L. and Berner , A. 1998 . Observed and Modelled Hygroscopic Behavior of Atmospheric Particles . Contr. Atmos. Phys./Beitr. Phys. Atmosph. , 71 ( 1 ) : 33 – 47 . (Vieweg)
- Bower , K. N. , Choularton , T. W. , Gallagher , M. W. , Beswick , K. M. , Flynn , M. , Allen , A. G. , Davison , B. M. , James , J. D. , Robertson , L. , Harrison , R. M. , Hewitt , C. N. , Cape , J. N. , McFadyen , G. G. , Milford , C. , Sutton , M. A. , Martinsson , B. G. , Frank , G. , Swietlicki , E. , Zhou , J. , Berg , O. H. , Mentes , B. , Papaspiropoulos , G. , Hansson , H.-C. , Leck , C. , Kulmala , M. , Aalto , P. , Väkevä , M. , Berner , A. , Bizjak , M. , Fuzzi , S. , Laj , P. , Facchini , M. C. , Orsi , G. , Ricci , L. , Nielsen , M. , Allan , B. J. , Coe , H. , McFiggans , G. , Plane , J. M. C. , Collett , Jr. J. L. , Moore , K. F. and Sherman , D. E. 2000 . ACE-2 HILLCLOUD: An Overview of the ACE-2 Ground Based Cloud Experiment Tellus B . 52 ( 2 ) : 749 – 777 . (Munksgaard)
- Brooks , B. J. , Smith , M. H. , Hill , M. K. and O'Dowd , C. D. 2002 . Size-Differentiated Volatility Analysis of Internally Mixed Laboratory-Generated Aerosol . J. Aerosol Sci. , 33 : 555 – 579 .
- Burtscher , H. , Baltensperger , U. , Bukowiecki , N. , Cohn , P. , Huglin , C. , Mohr , M. , Matter , U. , Nyeki , S. , Scmatloch , V. , Streit , N. and Weingartner , E. 2001 . Separation of Volatile and Non-volatile Aerosol Fractions by Thermodesorption: Instrumental Development and Applications . J. Aerosol Sci. , 32 : 427 – 442 .
- Chen , D. R. , Pui , Y. H. , Mulholland , W. and Fernandez , M. 1999 . Design and Testing of an Aerosol/sheath Inlet for High Resolution Measurements with a DMA . J. Aerosol Sci. , 30 ( 8 ) : 983 – 999 .
- Chen , Y. Y. and Lee , W. M. G. 1999 . Hygroscopic Properties of Inorganic-salt Aerosol with Surface-active Organic Compounds . Chemosphere , 38 ( 10 ) : 2431 – 2448 .
- Clarke , A. D. 1993 . Atmospheric Nuclei in the Pacific Atmosphere: Their Nature, Concentration and Evolution. . J. G. R.—Atmosphere , 98 ( D11 ) : 20633 – 47 .
- Covert , D. S. and Heintzenberg . 1993 . Size Distribution and Chemical Properties of Aerosol at Ny Alesund, Svalbard . Atmos. Environ , 27A : 2989 – 2997 .
- Cruz , C. N. and Pandis , S. N. 1998 . The Effect of Organic Coatings on the Cloud Condensation Nuclei Activation of Inorganic Atmospheric Aerosol . J. Geophysical Res , 103, D13 : 111 – 123 .
- Cruz , C. N. and Pandis , S. N. 2000 . Deliquescence and Hygroscopic Growth of Mixed Inorganic-Organic Atmospheric aerosol . Environ. Sci. Technol , 34 : 4313 – 4319 .
- Dua , S. K. , Hopke , P. K. and Raunemaa , T. 1998 . Hygroscopicity of Diesel Aerosols . Water Air and Soil Pollut. , 112 : 247 – 257 .
- Flagan , R. C. 1999 . On Differential Mobility Analyzer Resolution . Aerosol Sci. Technol , 30, 6 : 556 – 570 .
- Gysel , M. , Weingartner , E. and Baltensperger , U. 2002 . Hygroscopic of Aerosol Particles at Low Temperature. 2. Theoretical and Experimental Hygroscopic Properties of Laboratory Generated Aerosols . Environ. Sci. Technol , 36 : 63 – 68 .
- Hameri , K. , Rood , M. J. and Hansson , H. C. 1992 . Hygroscopic Properties of a NaCl Aerosol Coated with Organic Compounds . J. Aerosol Sci. , 23 : S437 – S440 .
- Hameri , K. , Charlson , R. J. , Hansson , H. C. and Jacobson , M. 1997 . Hygroscopic Properties of Ammonium Sulphate Aerosols Mixed with Slightly Soluble Organic Compound . J. Aerosol Sci. , 28 ( S1 ) : S153 – S154 .
- Hanssson , H. C. , Rood , M. J. , Koloutsou-Vakakis , S. , Hameri , K. , Orsini , D. and Wiedensohler , A. 1998 . NaCl Aerosol Particle Hygroscopicity Dependence on Mixing with Organic Compounds . J. Atmos. Chem , 31 : 321 – 346 .
- Hennig , T. , Massling , A. , Brechtel , F. J. and Wiedensohler , A. 2005 . A tandem DMA for Highly Temperature-stabilized Hygroscopic Particle Growth Measurement Between 90% and 95% Relative Humidity . Aerosol Sci. , 36 : 1210 – 1223 .
- Jennings , S. G. , Geever , M. , McGovern , F. M. , Francis , J. , Spain , T. G. and Donaghy , T. 1997 . Microphysical and Physico-chemical Characterization of Atmospheric Marine and Continental Aerosol at Mace Head . Atmos. Environ. , 31 ( 17 ) : 2795 – 2808 .
- Johnson , G. R. , Ristovski , Z. and Morawska , L. 2004 . Method for Measuringthe Hygroscopic Behavior of Lower Volatility Fractions in an Internally Mixed Aerosol . J. Aerosol Sci , : 443 – 455 .
- Kanakidou , M. , Seinfeld , J. H. , Pandis , S. N. , Barnes , I. , Dentener , F. J. , Facchini , M. C. , van Dingenen , R. , Ervens , B. , Nenes , A. N. C. J. S. E. , Putaud , J. P. , Balkanski , Y. , Fuzzi , S. , Horth , J. , Moortgat , G. K. , Winterhalter , R. , Myhre , C. E. L. , Tsigaridis , K. , Vignati , E. , Stephanou , E. G. and Wilson , J. 2005 . Organic Aerosol and Global Climate Modelling: A Review . Atmos. Chem. Phys , 5 : 1053 – 1123 .
- Kandler , K. and Shütz , L. 2007 . Climatology of the Average Water-soluble Volume Fraction of Atmospheric Aerosol . Atmos. Res , 83 : 77 – 92 .
- Kerminen , V. M. 1997 . The Effects of Particle Chemical Character and Atmospheric Processes on Particle Hygroscopic Properties . J. Aerosol Sci. , 28 ( 1 ) : 121 – 132 .
- Knutson , E. O. and Whitby , K. T. 1975 . Aerosol Classification by Electric Mobility: Apparatus, Theory, and Applications . J. Aerosol Sci , 6 : 443 – 451 .
- Kohler , H. 1936 . The Nucleus and Growth of Hygroscopic Droplets . Trans. Faraday Soc. , 32 : 1152 – 1161 .
- Kramer , L. , Poschl , U. and Niessner , R. 2000 . Microstructural Rearrangement of Sodium Chloride Condensation Aerosol Particles on Interaction with Vapor . J. Aerosol Sci , 6 : 673 – 685 .
- Kreidenweis , S. M. , Koehler , K. , DeMott , P. , Prenni , A. J. , Carrico , C. and Ervens , B. 2005 . Water Activity and Activation Diameters from Hygroscopicity Data—Part I: Theory and Application to Inorganic Salts . Atmos. Chem. Phys. Discuss. , 5 : 287 – 323 .
- Lehmann , K. , Massling , A. , Tilgner , A. , Mertes , S. , Galgon , D. and Wiedensohler , A. 2005 . Size-rsolved Soluble Volume Fractions of Submicrometer Particles in Air Masses of Different Character . Atmos. Environ. , 39 : 4257 – 4266 .
- Marinoni , A. , Laj , P. , Sellegri , K. and Mailhot , G. 2004 . Cloud Chemistry at the Puy de Dôme: Variability and Relationships with Environmental Factors . Atmos. Chem. Phys. , 4 ( 3 ) : 715 – 728 .
- Massling , A. , Wiedensohler , A. and Bush , B. 1999 . Concept of an Advanced Hygroscopic Tandem Differential Mobility Analyser with a Great Operation Stability . J. Aerosol Sci , 30 ( S1 ) : S395 – S396 .
- Mikhailov , E. , Vlasenko , S. , Niessner , R. and Poschl , U. 2003 . Interaction of Aerosol Particles Composed of Protein and Salts with Water Vapor: Hygroscopic Growth and Microstructural Rearrangement . Atmos. Chem. Phys. Discuss , 3 : 4755 – 4832 .
- O'Dowd , C. D. , Jennings , S. G. , Cooke , W. F. and Smith , M. H. 1992 . A High Temperature Volatility Technique for Determination of Atmospheric Aerosol Composition . J. Aerosol Sci. , 23 ( S1 ) : S905 – S908 .
- Orsini , D. A. , Wiedensohler , A. and Stratmann , F. 1998 . A New Tandem Differential Mobility Analyser to Measure the Volatile Sulfuric Acid Aerosol Fraction . Journal of Atmospheric and Oceanic Technology , 16 : 760 – 772 .
- Paulsen , D. , Weingartner , E. , Alfarra , M. R. and Baltensperger , U . 2006 . Volatility Measurements of Photochemically and Nebulizer-generated Organic Aerosol Particles . J. Aerosol Sci , 37 : 1025 – 1051 .
- Philippin , S. , Wiedensohler , A. and Stratmann , F. 2003 . Measurements of Non-volatile Fractions of Pollution Aerosols with an Eight-tube Volatility Differential Mobility Analyzer (VTDMA-8) . J. Aerosol Sci. , 35 : 185 – 203 .
- Pitchford , M. L. and McMurry , P. H. 1994 . Relationship Between Measured Water Vapor Growth and Chemistry of Atmospheric Aerosol for Grand Canyon, Arizona, in Winter 1990 . Atmos. Environ , 28 : 827 – 839 .
- Putaud , J. P. , Raes , F. , Van Dingenen , R. , Bruggemann , E. , Facchini , M. C. and Decesari , S . 2004 . European Aerosol Phenomenology—2: Chemical Characteristics of Particulate Matter at Kerbside, Urban, Rural and Background Sites in Europe . Atmos. Environ. , 38 ( 16 ) : 2579 – 2595 .
- Raes , F. , van Dingenen , R. , Vignati , E. , Wilson , J. , Putaud , J. P. , Seinfeld , J. H. and Adams , P. 2000 . Formation and Cycling of Aerosols in the Global Troposphere . Atmos. Environ , 34 : 4215 – 4240 .
- Rader , D. J. and McMurry , P. H. 1986 . Application of the Tandem Differential Mobility Analyser to Studies of Droplet Growth or Evaporation . J. Aerosol Sci. , 17 : 771 – 787 .
- Rissler , J. , Vestin , A. , Swietlicki , E. , Fisch , G. , Zhou , J. , Artaxo , P. and Andreae , M. O. 2006 . Size Distribution and Hygroscopic Properties of Aerosol Particles from Dry-season Biomass Burning in Amazonia . Atmos. Chem. Phys. , 6 : 471 – 491 .
- Saxena , P. and Hildemann , L. M. 1996 . Water-soluble Organics in Atmospheric Particles: A Critical Review of the Literature and Application of Thermodynamics to Identify Candidate Compounds . J. Atmos. Chem , 24 : 57 – 109 .
- Sellegri , K. , Laj , P. , Peron , F. , Dupuy , R. , Legrand , M. , Preunkert , S. , Putaud , J.-P. , Cachier , H. and Ghermandi , G. 2003 . Mass Balance of Free Tropospheric Aerosol at the Puy de Dôme (France) in Winter . J. Geophys. Res , 108 ( D11 ) : 4333
- Sellegri , K. , Villani , P. , Picard , D. , Dupuy , R. , O'Dowd , C. and Laj , P. 2008 . Role of the Volatile Fraction of Submicron Marine Aerosol on its Hygroscopic Properties . Atmos. Res , in press
- Sjogren , S. , Gysel , M. , Weingartner , E. , Baltensperger , U. , Cubison , M. J. , Coeb , H. , Zardini , A. A. , Marcolli , C. , Krieger , U. K. and Peter , T. 2007 . Hygroscopic Growth and Water Uptake Kinetics of Two-phase Aerosol Particles Consisting of Ammonium Sulfate, Adipic and Humic Acid Mixtures . Aerosol Sci. , 38 : 157 – 171 .
- Stokes , R. H. and Robinson , R. A. 1966 . Interactions in Aqueous Nonelectrolyte Solutions. I. Solute-solvent equilibria . J. Phys. Chem. , 70 : 2126 – 2130 .
- Stolzenburg , M. R. and McMurry , P. H. 1988 . TDMAFIT Users' Manual , Minneapolis : Particle Technology Laboratory Department of Mechanical Engineering University of Minnesota . PTL Publication No. 653
- Svenningsson , B. , Hansson , H.-C. , Wiedensohler , A. , Ogren , J. A. , Noone , K. J. and Hallberg , A. 1992 . Hygroscopic Growth of Aerosol Particles in the Po-Valley . TELLUS , 44B : 556 – 569 .
- Svenningsson , B. , Hansson , H.-C. , Wiedensohler , A. , Noone , K. , Ogren , J. , Hallberg , A. and Colvile , R. 1994 . Hygroscopic Growth of Aerosol Particles and its Influence on Nucleation Scavenging in Cloud: Experimental Results From Kleiner Feldberg . J. Atmos. Chem. , 19 : 129 – 152 .
- Svenningsson , B. , Rissler , J. , Swietlicki , E. , Mircea , M. , Bilde , M. , Facchini , M. C. , Decesari , S. , Fuzzi , S. , Zhou , J. , Monster , J. and Rosenorn , T. 2005 . Hygroscopic Growth and Critical Supersaturations for Mixed Aerosol Particles of Inorganic and Organic Compounds of Atmospheric Relevance . Atmos. Chem. Phys. Discuss. , 5 : 2833 – 2877 .
- Swietlicki , E. , Zhou , J. , Covert , D. S. , Hameri , K. , Busch , B. , Vakeva , M. , Dusek , U. , Berg , O. H. , Wiedensholer , A. , Aalto , P. , Makela , J. , Martinsson , B. , Papaspiropoulos , G. , Mentes , B. , Frank , G. and Stratmann , F. 2000 . Hygroscopic Properties of Aerosol Particles in the North-Eastern Atlantic during ACE-2 . Tellus , 52B : 201 – 227 .
- Topping , D. O. , McFiggans , G. B. and Coe , H. 2005a . A Curved Multi-component Aerosol Hygroscopicity Model Framework: Part 1—Inorganic Compounds . Atmos. Chem. Phys. , 5 : 1205 – 1222 .
- Villani , P. , Picard , D. , Marchand , N. and Laj , P. 2007 . Design and Validation of a 6-Volatility Tandem Differential Mobility Analyzer (VTDMA) . Aerosol Sci. Technol , 41 : 10,898 – 906 .
- Wang , J. 2002 . Diffusional Losses in Particle Sampling Systems Containing Bends and Elbows . J. Aerosol Sci. , 33 : 843 – 857 .
- Wahlin , P. , Palmgren , F. and Van Dingenen , R. 2000 . Experimental Studies of Ultrafine Particles in Streets and the Relationship to Traffic . Atmos. Environ , 35, S1 : S63 – S69 .
- Weingartner , E. , Gysel , M. and Baltensperger , U. 2002 . Hygroscopic of Aerosol Particles at Low Temperature. 1. New Low-temperature H-TDMA instrument: Setup and First Applications . Environ. Sci. Technol , 36 : 55 – 62 .
- Wiedensohler , A. 1988 . An Approximation of the Bipolar Charge Distribution for Particles in the Submicron Size Range . J. Aerosol Sci. , 19 : 387 – 389 .
- Zhang , X. Q. , McMurry , P. H. , Hering , S. V. and Casuccio , G. S. 1993 . Mixing Characteristics and Water Content of Submicron Aerosols Measured in Los Angeles and at the Grand Canyon . Atmos. Environ , 27A : 1593 – 1607 .