Abstract
Micro-Raman spectroscopy (micro-RS) and Temperature Programmed Oxidation (TPO) combined with FTIR gas analysis have been used to determine structural changes and oxidation behavior in samples of spark discharge (GfG) and heavy duty engine (EURO IV) soot upon oxidation by oxygen in a temperature range between 293 K and 873 K. Raman spectra of soot and FTIR spectra of oxidation products have been recorded before and during the oxidation process. For micro-RS analysis spectral parameters have been determined by a five band curve fitting procedure (G, D1–D4). For GfG soot the relative intensity of D3 band is decreasing and the two observed Raman peaks are getting more separated during the TPO. This suggests a rapid preferential oxidation of highly reactive amorphous carbon. The decrease of the D1 band width indicates a decrease of chemical heterogeneity and an increase of structural order upon oxidation. Changes in Raman spectroscopic parameters are in good agreement with the behavior of soot during oxidation determined by CO2 emission with FTIR. In contrast to GfG soot the spectral parameters of EURO IV soot remained mostly unchanged during the oxidation process, so that EURO IV soot shows just minor changes in structure upon oxidation. Overall Raman spectroscopic parameters provide information about changes in structural order of graphitic and amorphous carbon fractions during oxidation and can be used to analyze oxidation readiness of soot. Thus micro-Raman spectroscopy may become a rapid analytical tool for the determination of soot reactivity by analysis of the structure.
INTRODUCTION
The attention to the relationship between quality of air and human health has been increasing in the last years. Atmospheric aerosol particles generated from a wide range of natural and anthropogenic sources have been cited as a risk to human health and form a class of air pollutants of great concern to air quality (CitationBernstein et al. 2004). Soot particles emitted by diesel engines account for a major fraction of air pollutants in urban area (CitationSydbom et al. 2001). Therefore, present and future emission limits of diesel particulate matter (DPM) require that these particles are efficiently removed from diesel engine exhaust. A wide range of particle trapping and exhaust treatment technologies, like diesel particulate filters (DPF), continuously regenerating traps (CRT) or Selective Catalytic Reduction (SCR), have been proposed and are currently under development. The filters and traps have to be regenerated periodically by gasification and oxidation of the deposited soot (CitationHiggins et al. 2002; CitationMesserer et al. 2006, Citation2004; CitationMüller et al. 2006; CitationSaracco et al. 1999). The behavior of this regeneration step is strongly depending on the reactivity of the deposited soot particles (CitationMüller et al. 2006, Citation2005; CitationSu et al. 2004a; CitationSu et al. 2004b).
A new way of solving the regeneration problem would be to create highly reactive soot which makes it possible to regenerate diesel aftertreatment systems at relatively low temperatures (CitationSu et al. 2004b). Therefore it is necessary to establish a rapid analytical tool to determine the reactivity of different types of soot and to get a better knowledge about the relationship between reactivity and structure of soot.
Soot is defined as the black solid product of incomplete combustion or pyrolysis of fossil fuels and other organic materials. It consists mostly of carbon and is composed of agglomerated primary particles with diameters between 10–30 nm which build up nanocrystalline and amorphous domains. The graphite-like crystalline domains typically consist of graphene layers, with average lateral extensions of up to 3 nm and can be regarded as highly disordered graphitic lattices (CitationMüller et al. 2005; CitationSu et al. 2004a; CitationSadezky et al. 2005). The amorphous domains are composed of polycyclic aromatic compounds, which can be regarded as graphene layer precursors in irregular or onion-like arrangements and other organic and inorganic components (CitationSadezky et al. 2005; CitationFerrari and Robertson 2000; CitationNegri et al. 2002; CitationSze et al. 2001).
Experiments concerning the reactivity of soot are usually done by Temperature Programmed Oxidation (TPO), which allows a quantification of the oxidized soot and a calculation of the reactivity by detection of the emitted carbon-oxides with IR spectroscopy (CitationMesserer et al. 2006) or mass spectrometry (CitationMüller et al. 2005). For investigation of soot structure High Resolution Transmission Electron Microscopy (HRTEM) is usually applied. Recent electron microscopic investigations have shown that differences in the oxidation behavior of different types of soot are associated with the different microstructures (CitationMüller et al. 2006, Citation2005; CitationSu et al. 2004a). However, TPO and HRTEM measurements are very time and cost consuming. On the other hand Raman spectroscopy can be used to get detailed information about the reactivity of soot by measuring the structure. Raman spectroscopy in general provides fingerprint spectra and allows a characterization of a wide range of chemical substances (CitationSadezky et al. 2005; CitationIvleva et al. 2005; CitationMcCreery 2000).
A correlation between the structure of soot and Raman spectra/Raman spectroscopic parameters has been reported and discussed in several publications (CitationSadezky et al. 2005; CitationFerrari and Robertson 2000; CitationSze et al. 2001; CitationBeyssac et al. 2003; CitationDippel et al. 1999; CitationFang and Lance 2004; CitationIvleva et al. 2007a; CitationIvleva et al. 2007b; CitationJawhari et al. 1995; CitationRosen and Novakov 1977; CitationVander Wal et al. 2004).
Raman spectroscopy has already been applied to investigate the oxidation/graphitization of different carbonaceous materials. The analysis of a correlation between Raman spectroscopic parameters of coal and other carbonaceous materials and their reactivity in temperature programmed oxidation experiments showed, that the position of the G peak, in the spectra of untreated samples, characterizes the degree of structural order and is linearly correlated with the oxidation temperature at which the rate of CO2 production exhibits a maximum (CitationVan Doorn et al. 1990). Raman spectroscopy combined with lattice fringe analysis of HRTEM images has been tested for the characterization of structural changes in an amorphous carbon black upon thermal annealing (CitationVander Wal et al. 2004). Authors have reported that a narrowing of the observed G and D peaks indicates an increase of homogeneity of the sample as disordered carbon was reorganized and joined to growing lamella. The change in relative intensities of the peaks (decrease of D and increase of G peak) was associated with increasing graphitization induced by higher temperatures (2223, 2573, and 3273 K). Furthermore, Raman spectroscopy and thermal analysis has been applied to study the influence of soot tempering and transformation on DPF regeneration at 923–1223 K (CitationFang and Lance 2004). Thereby a narrowing of the two observed peaks (at 1580–1590 cm−1 (G) and 1310–1360 cm−1 (D)) and an increase of the G/D peak intensity ratio occurred, suggesting that the investigated diesel soot underwent gradual transformation from amorphous into more ordered polyaromatic structures, which exhibit higher resistance towards oxidation.
Recently we have demonstrated the potential of Raman Microspectroscopy (which combines the analytical capabilities of Raman spectroscopy with the spatial resolution of an optical microscope) for analysis of changes in chemical structure of soot undergoing oxidation by nitrogen dioxide at constant temperatures (523 K and 573 K) (CitationIvleva et al. 2007b). In this study we applied micro-Raman spectroscopy (micro-RS) and TPO to investigate changes in structure and reactivity of soot during oxidation and gasification by oxygen at increasing temperature, to get an overview on temperature dependency of soot oxidation. The use of oxygen instead of nitrogen dioxide, as it was done in previous work, is interesting because of the harmful influence of nitrogen dioxide on the environment. We analyzed the correlation between Raman spectroscopic parameters and reactivity of GfG soot and EURO IV soot, in a temperature range between 293 K and 873 K with a heating rate of 5 K/min, to get a better knowledge of the oxidation behavior of soot particles in diesel exhaust.
METHODS
Two different types of soot have been used during the oxidation experiments. As model soot we used spark discharge soot (GfG), artificially generated by a spark discharge generator (Palas GfG 1000) (CitationMesserer et al. 2006). Real diesel soot (EURO IV) samples were taken from the undiluted raw exhaust of a heavy duty engine (MAN D20, 10.6 l, 320 kW) under World Harmonized Transient Cycle conditions (WHTC). All soot samples were collected on Bekipor Fecralloy metal fiber filter material with a fiber diameter of ∼ 10 μ m and a filter diameter of 47 mm. Oxidation experiments have been performed in a diesel exhaust aftertreatment model system at temperatures varying from 293 K up to 873 K and a total gasflow of 3 l/min of nitrogen including 5% of oxygen. Heating rate during the experiments was 5 K/min and the temperature of the filter substrate was adjusted with a Proportional-Integral-Derivative (PID) temperature controller on the surface of the filter material, using a type K thermocouple. Quantification of oxidation products was done by FTIR spectroscopy using a Bruker IFS 66/s FTIR Spectrometer, including a 2 l gasflow cell with an optical path length of 6.4 m (CitationMesserer et al. 2006). Raman spectra have been taken by a Renishaw 2000 Raman microscope system (Renishaw, UK), using a laser wavelength of λ0 = 514 nm, 25 mW. Wavelength calibrations were performed with a silicon wafer (1 s integration time, × 50 objective) by utilizing the first order phonon band of Si at 520 cm−1. Spectra of the soot samples before and after oxidation were recorded over the range of 800–2000 cm− 1 (Stokes shift) with a spectral resolution of ∼ 6 cm− 1, 50× magnification objective, 10 s integration time, defocused laser beam (diameter ≈ 40 μ m) and 50% of the source power. Spectra were processed via WiRE 1.2 software (Renishaw, UK) running under GRAMS/32 (Thermo Galactic, USA), that was used to control the Raman microscope system and data acquisition, as well as the processing and analysis (curve fitting) of spectra. Spectra were fitted after the multi-point baseline correction by combination of four Lorentzian-shaped G, D1, D2, and D4 bands at about 1580, 1350, 1620, and 1200 cm−1, respectively, with Gaussian-shaped D3 band at about 1500 cm−1, (CitationSadezky et al. 2005; CitationIvleva et al. 2007a; CitationIvleva et al. 2007b). For each Raman spectrum, the fitting procedure was repeated three times to ensure the reproducibility of the fit. It was found that standard deviations of D1 band width and D3 band intensity for each spectrum did not exceed 2%. Mean values and standard deviations of the spectral parameters presented and discussed below were obtained from seven spectra recorded from different regions on the sample. The good agreement between the data sets obtained from repeated oxidation experiments for GfG soot indicates high reproducibility and reliability of the measurements and fitting procedures.
The first-order Raman spectra of soot and related carbonaceous materials are generally characterized by two broad and strongly overlapping peaks with intensity maxima near 1580 cm− 1 (G or “Graphite” peak) and 1350 cm− 1 (D or “Defect” peak). Based on experimental observations and theoretical calculations, up to five bands corresponding to different vibration modes of crystalline or molecular structures in the sample material have been suggested to account for the observed spectra (CitationSadezky et al. 2005; CitationSze et al. 2001; CitationDippel et al. 1999). The G band at ∼ 1580 cm− 1 has been attributed to ideal graphitic lattice (E2g symmetry) stretching mode (CitationSadezky et al. 2005; CitationFerrari and Robertson 2000). Other bands contributing to the observed spectrum, are typical for disordered graphitic lattices (D bands). The D1 band at ∼ 1350 cm− 1 has been associated with a vibration mode involving graphene layer edges (A1g symmetry) (CitationSadezky et al. 2005; CitationFerrari and Robertson 2000), and the D2 band at ∼ 1620 cm− 1 has been attributed to a vibration mode involving surface graphene layers (E2g symmetry) (CitationSadezky et al. 2005; CitationFerrari and Robertson 2000). High signal intensities between the two main peaks of the observed spectra have been assigned to a D3 band at ∼ 1500 cm− 1 that is associated with the amorphous carbon content of soot (CitationSadezky et al. 2005; CitationBeyssac et al. 2003; CitationDippel et al. 1999; CitationJawhari et al. 1995). Moreover, weak bands at ∼ 1200 cm− 1 (D4) have been assigned to vibrations of disordered graphite lattices (A1g symmetry), sp2- and sp3-hybridized carbon bonds, C–C and C = C stretching vibrations of polyenes and ionic impurities (CitationSadezky et al. 2005; CitationDippel et al. 1999). For structural characterization of soot and related carbonaceous materials, a range of different spectroscopic parameters and curve fitting procedures have been proposed (CitationSadezky et al. 2005; CitationSze et al. 2001; CitationBeyssac et al. 2003; CitationDippel et al. 1999; CitationJawhari et al. 1995). CitationSadezky et al. (2005) have tested and compared nine different band combinations and demonstrated that the best fit to the Raman spectra of a wide range of soot samples and related carbonaceous materials was obtained with four Lorentzian bands (G, D1, D2, and D4) and one Gaussian band (D3). Our recent studies demonstrated that D1 band width and D3 band intensity obtained by this five band fitting procedure can provide information about the relative abundance and structural order of graphite-like and amorphous carbon and give information about structure of different types of soot (CitationIvleva et al. 2007b).
RESULTS AND DISCUSSION
Mass Loss of GfG and EURO IV Soot Upon Oxidation
shows mass ratio (m/m0, the mass remaining after oxidation to a given temperature normalized by initial soot mass) and data of FTIR CO2 measurements for GfG and EURO IV soot samples oxidized at increasing temperature. The mass ratio was determined by gravimetry of the loaded filter material and by integration of the FTIR CO2 signal. and 1c illustrate a good agreement for the values of mass ratio determined by two different methods for GfG and EURO IV soot. Moreover, for GfG soot the gravimetric measurements were undertaken in two similar oxidation experiments, to prove the stability of the system ().
FIG. 1 Mass ratio (m/m0) (a, c) and normalized CO2 signals (b, d) of GfG soot and EURO IV soot during oxidation at increasing temperature (293 K–873 K), heating rate of 5 K/min.
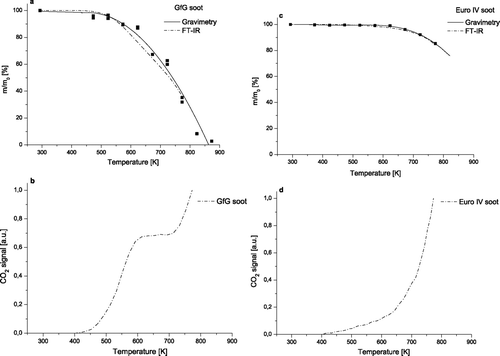
For GfG soot () the analysis of mass ratio revealed a rapid oxidation starting at about 450 K, which might be caused by oxidation of highly reactive amorphous structures. At high temperatures the amount of soot which is oxidized, is much higher in GfG soot than in EURO IV soot (ca. 65% versus ca. 10% at 773 K), suggesting a more reactive structure of GfG soot than EURO IV soot. The onset temperature of the GfG soot combustion is in good agreement with literature data (CitationMüller et al. 2005).
The CO2 signal for GfG soot oxidation normalized to the maximum is shown in . The signal was recorded during the TPO up to 773 K. Significant oxidation starts from 473 K and reaches a plateau at 573 K, where the emission of CO2 stays constant up to 723 K. Obviously, the highly reactive amorphous carbon fraction has been oxidized at this temperature. At 723 K a second combustion step can be observed until the oxidation has been stopped at 773 K. At that temperature 65% of the total amount of GfG soot was oxidized ().
For EURO IV soot () the combustion starts at higher temperatures (about 550 K) and seems to proceed much slower compared to GfG soot, suggesting that the EURO IV soot used in this study is characterized by a very unreactive structure. The onset temperature of the EURO IV soot combustion is in good correlation with literature data (CitationSu et al. 2004a). Overall, comparison of the two independent measuring techniques used to determine mass ratio demonstrates that the FTIR and the gravimetric measurements are both appropriate for analysis of soot combustion and show similar oxidation behavior for each type of soot.
The normalized CO2 signal for EURO IV soot oxidation is shown in . The onset combustion temperature for the EURO IV soot is observed at 573 K. The lower emission of CO2 in the low temperature range could be explained by a lower functionalization of the surface of EURO IV soot compared with GfG soot (CitationSu et al. 2004a). Overall, the oxidation of EURO IV soot occurs more slowly compared to GfG soot and shows no plateau between 473 K and 573 K. At 773 K the CO2 emission has just reached 15% of the total combustion ().
Changes in Raman Spectra of GfG and EURO IV Soot Upon Oxidation
shows the Raman spectra of untreated soot and the evolution of spectra after oxidation by 5% oxygen at increasing temperature. For GfG and EURO IV soot ten temperature steps have been analyzed. Spectra for each temperature plateau correspond to the average of seven spectra, thus they are highly representative for that temperature.
FIG. 2 Raman spectra (λ0 = 514 nm) of GfG soot (a) and EURO IV soot (b) after oxidation at different temperatures with a heating rate of 5 K/min.
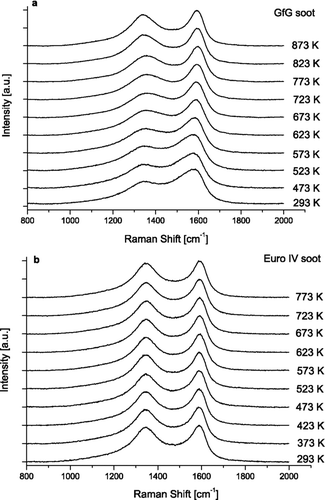
The untreated GfG spark discharge soot and EURO IV soot show two overlapping peaks around 1580 (G or “Graphite” peak) and 1350 cm–1 (D or “Defect” peak) that are typical for soot and related carbonaceous materials. The peaks in the spectra of GfG soot () became narrowed with increasing reaction time, whereas the spectra of EURO IV soot () remained largely unchanged. Different behavior of GfG and EURO IV soot during oxidation can be explained by differences in their structure. This assumption is in good agreement with recent HRTEM analysis of untreated GfG and Euro IV soot (CitationSu et al. 2004a). HRTEM images showed that GfG soot possesses a more heterogeneous and functionalized structure than EURO IV soot and therefore it can be oxidized much faster. It was shown that high amount of defects and deviation of the carbons from a perfect graphitic structure results in an increased surface functionalization. In our further work we are planning to combine micro-RS and HRTEM for the analysis of partially oxidized soot in order to validate the results of micro-RS and get more information about structural changes of soot during oxidation.
For quantitative spectral analysis the five band fitting procedure was applied (CitationSadezky et al. 2005; CitationIvleva et al. 2007a; CitationIvleva et al. 2007b). Curve fitting is based on combination of four Lorentzian-shaped G (ideal graphitic lattice), D1, D2, D4 (disordered graphitic lattice) bands at about 1580, 1350, 1620, and 1200 cm−1 respectively, with Gaussian-shaped D3 (amorphous carbon) band at about 1500 cm−1. Band position (Stokes Shift, cm−1), full width at half maximum (FWHM, cm−1) and integrated band intensity relative to the G band (I/I G ) were determined from the spectra of fresh and oxidized GfG and EURO IV soot samples. An exemplary spectrum of untreated EURO IV soot with five band fits is shown in .
Recently, we have demonstrated that the D1 FWHM and the D3 band intensity are the spectroscopic parameters which can provide information about the relative abundance and structural order of graphite-like and amorphous carbon and give most information about the chemical structure (CitationIvleva et al. 2007a) and reactivity of different types of soot (CitationIvleva et al. 2007b). Therefore, in present study we focused on discussions of changes in the D1 FWHM and the D3 band relative intensity during oxidation of GfG and EURO IV soot.
Changes of Spectral Parameters and Mass Conversion of GfG and EURO IV Soot
As shown in for untreated GfG soot the FWHM of D1 band lies at 225 ± 7 cm−1 and remains in the range of the standard deviations until about 673 K. Then it decreases rapidly down to 170 ± 6 cm−1. This progressive decrease of D1 FWHM indicates a decrease of chemical heterogeneity and an increase of structural order as it has already been reported in literature (CitationIvleva et al. 2007a).
FIG. 4 Changes in FWHM of D1 band (a, c) and relative intensity of D3 band (R3) (b, d) for GfG soot and EURO IV soot during oxidation versus temperature.
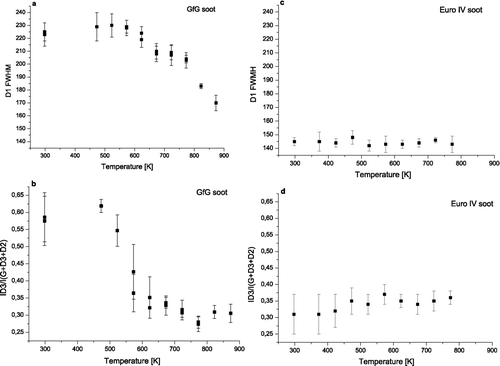
Also the relative intensity of D3 band, (R3 = I D3/(I D3 + I D2 + I G )) (CitationIvleva et al. 2007b) has been calculated and plotted against temperature. R3 is closely related to the amorphous carbon content. shows a rapid decrease of the D3 band relative intensity from 0.57 ± 0.07 at 293 K to 0.33 ± 0.05 at 623 K, which indicates a decrease of amorphous carbon content. From 623 K the R3 value remains constant in the range of standard deviations.
shows D1 FWHM for untreated and oxidized EURO IV soot. The lower starting value for EURO IV soot at 145 ± 3 cm−1 indicates a much lower degree of chemical heterogeneity and structural disorder compared to GfG soot (225 ± 7 cm−1). The changes in D1 FWHM during oxidation are within standard deviation values. The final value at 773 K lies at around 143 ± 5 cm−1.
For EURO IV soot the starting value of R3 parameter (0.32 ± 0.07), as shown in , is nearly two times lower than the value for GfG soot (0.57 ± 0.07). That indicates a lower content of amorphous carbon in EURO IV soot. During the oxidation, the changes in the mean values of D3 band relative intensity hardly exceeded the standard deviations. The variations for R3 parameter can be associated with structural differences between various measurement points.
In order to analyze the correlations between Raman spectroscopic parameters and soot reactivity, the D1 band width for GfG soot has been plotted against mass conversion (1 - m/m0) determined by gravimetry (). It was found that up to ca. 20% of mass conversion the changes in the mean values of D1 FWHM (225 ± 7 cm−1 for untreated soot) stay in the range of standard deviations. After that, D1 band width decreases slowly (down to 170 ± 7 cm−1) until almost complete mass conversion (98%) indicating overall increase of chemical homogeneity and structural order in the soot samples upon TPO.
FIG. 5 Changes in FWHM of D1 band (a) and relative intensity of D3 band (R3) (b) for GfG soot during oxidation versus mass conversion (1 - m/m0) determined by gravimetry.
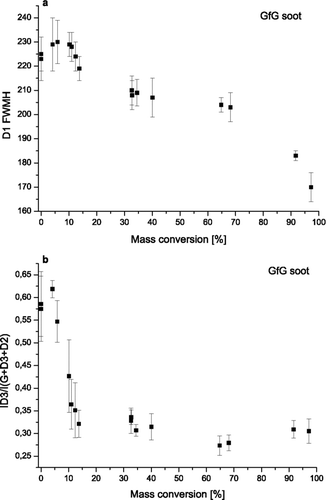
shows a steep decrease of R3 parameter of GfG soot from 0.57 ± 0.07 at the beginning of the oxidation to 0.32 ± 0.03 at 13% of mass conversion. From about 20% of mass conversion the R3 parameter remains constant until the end of the TPO. In fact 20% of mass conversion corresponds exactly to 623 K, the temperature where the decrease of R3 parameter ends as shown in . This steep decrease suggests preferential oxidation of the highly reactive amorphous carbon fraction. The amount of amorphous carbon in GfG can be estimated as 20%.
In conclusion D3 band relative intensity and D1 band width are well correlated with amorphous carbon content and with the structural order in GfG soot. Actually, these two parameters are significantly changing in two different temperature regions. EURO IV soot seems to be partially characterized by well distinguishable structures like amorphous carbon and graphitic lattices. Here the structure seems to be more homogeneous and more organized.
Reactivity-Structure Correlations for GfG and EURO IV Soot
As already mentioned FTIR CO2 spectra were constantly taken during the TPO, to get an overview about CO2 emission and thereby reactivity of the oxidized soot. and 1d show the normalized FTIR CO2 signals for GfG and EURO IV soot that have been recorded during the TPO up to 773 K with a heating rate of 5 K/min. In both cases no signal is registered below 373 K, but starting from 423 K a low signal due to the emission of CO2 can be observed by oxidation of the GfG soot. In this case surface functional groups and fractions of highly reactive carbon are decomposing. This has also been registered in experiments with gasification done only by nitrogen and it shows that the oxidation at this temperature seems to be carried out predominantly by oxygen atoms within the soot surface. A pronounced oxidation of GfG soot, that can be associated with the combustion of highly reactive amorphous carbon, starts from 473 K and reaches a plateau at 573 K. Up to 723 K the emission of CO2 stays constant. The amount of amorphous carbon seems to be much higher in GfG soot, so this type of soot can be oxidized much faster. At 723 K a second oxidation step can be observed until the oxidation has been stopped at 773 K. At that temperature 65% of the total amount of GfG soot was oxidized. This value is in good correlation with the results of mass conversion determined by gravimetry.
Next step is to compare the evolution of CO2 emission (), which gives information on reactivity, with Raman analysis of specific spectral parameters, which provide information on structure. The relative intensity of D3 band shows a strong decrease between 473 K and 573 K () and then remains constant in the range of standard deviation until the end of TPO. Since the decrease of R3 is associated with a decrease of amorphous carbon content, almost all amorphous carbon is oxidized before 573 K. Indeed, the first strong oxidation step ends at 573 K when the less organized and most reactive carbon is already oxidized. The plateau between 573 K and 723 K indicates that the reaction cannot continue with such a high oxidation rate. An explanation could be that the temperature is not high enough to oxidize the soot as fast as before, since the remaining soot is characterized by a more organized structure. This situation changes again at the beginning of the second oxidation step at 723 K. Here also remaining soot particles (disordered graphitic lattices) are going to become oxidized. Right from this temperature D1 FWHM () starts to decrease significantly, indicating an increase of structural order.
The onset combustion temperature for the EURO IV soot is observed around 473 K. The lower emission of CO2 in the low temperature range could be explained by a lower functionalization of the surface of EURO IV soot compared with GfG soot (CitationSu et al. 2004a). Overall, the oxidation of EURO IV soot occurs more slowly compared to GfG soot and shows no plateau between 573 K and 723 K. At 773 K the CO2 emission has just reached 15% of the total combustion (65% for GfG soot), hence the lower increase of CO2 emission rate at EURO IV soot suggests a lower amount of amorphous carbon and higher structural order in EURO IV soot than in GfG soot.
IMPLICATIONS AND OUTLOOK
The low amount of amorphous carbon and higher structural order seems to be responsible for the small changes in EURO IV soot Raman spectra at different TPO steps. Indeed, amorphous carbon fraction contributes substantially to the structure of untreated GfG soot. When this fraction is oxidized the Raman spectra and spectral parameters change. Also the graphitic fraction for GfG soot seems to be heterogeneous, leading to changes in spectral parameters also during high temperature oxidation. On the other hand EURO IV soot seems to have a more homogeneous structure dominated by disordered and small in-plane-dimensioned graphitic lattices, so that Raman spectra hardly change upon oxidation. This suggests that during the shrinking-core combustion of EURO IV soot the relative amount of an organized structure remains quite stable.
In this study Temperature Programmed Oxidation with FTIR CO2 analysis and micro-Raman spectroscopy have been combined to get information on structure (from micro-RS analysis) and reactivity (from TPO experiments) of GfG and EURO IV soot undergoing oxidation and gasification by oxygen. The aim of this research was to supply information on changes in structure and reactivity of different types of soot during the oxidation process. GfG and EURO IV samples soot have been gasified using oxygen instead of nitrogen dioxide as it was done in previous work. This could be one step further to help reducing the particle emissions in diesel engines by optimization of the regeneration process of diesel particulate filters (DPF) and thereby to help protecting the environment. A better knowledge of the structure reactivity correlations would help to establish micro-Raman spectroscopy as a rapid analytical tool to predict reactivity of soot by analyzing the structure. To get more detailed information, further measurements with other types of soot have to be performed. Therefore also HRTEM images of partially oxidized soot samples will be added to get direct information about structure and morphology of soot.
Acknowledgments
Financial support by DFG (Ni 261/21-1) is gratefully acknowledged.
REFERENCES
- Bernstein , J. A. , Alexis , N. , Barnes , C. , Bernstein , I. L. , Bernstein , J. A. , Nel , A. , Peden , D. , Diaz-Sanchez , D. , Tarlo Susan , M. and Williams , P. B. 2004 . Health Effects of Air Pollution . J. Allergy Clin. Immunol. , 114 : 1116 – 1123 .
- Beyssac , O. , Goffe , B. , Petitet , J.-P. , Froigneux , E. , Moreau , M. and Rouzaud , J.-N. 2003 . On the Characterization of Disordered and Heterogeneous Carbonaceous Materials by Raman Spectroscopy . Spectrochim. Acta, Part A , 59A : 2267 – 2276 .
- Dippel , B. , Jander , H. and Heintzenberg , J. 1999 . NIR FT Raman Spectroscopic Study of Flame Soot . Phys. Chem. Chem. Phys. , 1 : 4707 – 4712 .
- Fang , H. L. and Lance , M. J. 2004 . Influence of Soot Surface Changes on DPF Regeneration . Soc. Automot. Eng., SP , SP–1898 : 89 – 97 .
- Ferrari , A. C. and Robertson , J. 2000 . Interpretation of Raman Spectra of Disordered and Amorphous Carbon . Phys. Rev. B: Condens. Matter , 61 : 14095 – 14107 .
- Higgins , K. J. , Jung , H. , Kittelson , D. B. , Roberts , J. T. and Zachariah , M. R. 2002 . Size-Selected Nanoparticle Chemistry: Kinetics of Soot Oxidation . J. Phys. Chem. , 106 : 96 – 103 .
- Ivleva , N. P. , McKeon , U. , Niessner , R. and Pöschl , U. 2007a . Raman Microspectroscopic Analysis of Size-Resolved Atmospheric Aerosol Particle Samples Collected with an ELPI: Soot, Humic-Like Substances, and Inorganic Compounds . Aerosol Sci. Technol. , 41 : 655 – 671 .
- Ivleva , N. P. , Messerer , A. , Yang , X. , Niessner , R. and Pöschl , U. 2007b . Raman Microspectroscopic Analysis of Changes in the Chemical Structure and Reactivity of Soot in a Diesel Exhaust Aftertreatment Model System . Environ. Sci. Technol. , 41 : 3702 – 3707 .
- Ivleva , N. P. , Niessner , R. and Panne , U. 2005 . Characterization and Discrimination of Pollen by Raman Microscopy . Anal. Bioanal. Chem. , 381 : 261 – 267 .
- Jawhari , T. , Roid , A. and Casado , J. 1995 . Raman Spectroscopic Characterization of Some Commercially Available Carbon Black Materials . Carbon , 33 : 1561 – 1565 .
- McCreery , R. L. 2000 . Raman Spectroscopy for Chemical Analysis. , 15 – 35 . New York : John Wiley & Sons .
- Messerer , A. , Niessner , R. and Pöschl , U. 2006 . Comprehensive Kinetic Characterization of the Oxidation and Gasification of Model and Real Diesel Soot by Nitrogen Oxides and Oxygen Under Engine Exhaust Conditions: Measurement, Langmuir–Hinshelwood, and Arrhenius Parameters . Carbon , 44 : 307 – 324 .
- Messerer , A. , Rothe , D. , Pöschl , U. and Niessner , R. 2004 . Advances in the Development of Filterless Soot Deposition Systems for the Continuous Removal of Diesel Particulate matter . Top. Catal , 30/31 : 247 – 250 .
- Müller , J. O. , Su , D. S. , Jentoft , R. E. , Kröhnert , J. , Jentoft , F. C. and Schlögl , R. 2005 . Morphology-Controlled Reactivity of Carbonaceous Materials Towards Oxidation . Catal. Today , 102–103 : 259 – 265 .
- Müller , J. O. , Su , D. S. , Jentoft , R. E. , Wild , U. and Schlögl , R. 2006 . Diesel Engine Exhaust Emission: Oxidative Behavior and Microstructure of Black Smoke Soot Particulate . Environ. Sci. Technol. , 40 : 1231 – 1236 .
- Negri , F. , Castiglioni , C. , Tommasini , M. and Zerbi , G. 2002 . A Computational Study of the Raman Spectra of Large Polycyclic Aromatic Hydrocarbons: Toward Molecularly Defined Subunits of Graphite . J. Phys. Chem. , 106 : 3306 – 3317 .
- Rosen , N. and Novakov , T. 1977 . Raman Scattering and Characterisation of Atmospheric Aerosol Particles . Nature , 266 : 708 – 710 .
- Sadezky , A. , Muckenhuber , H. , Grothe , H. , Niessner , R. and Pöschl , U. 2005 . Raman Spectra of Soot and Related Carbonaceous Materials: Spectral Analysis and Structural Information . Carbon , 43 : 1731 – 1742 .
- Saracco , G. , Badini , C. and Specchia , V. 1999 . Catalytic Traps for Diesel Particulate Control . Chem. Eng. Sci. , 54 : 3035 – 3041 .
- Su , D. S. , Jentoft , R. E. , Müller , J. O. , Rothe , D. , Jacob , E. , Simpson , C. D. , Tomovic , Z. , Müllen , K. , Messerer , A. , Pöschl , U. , Niessner , R. and Schlögl , R. 2004a . Microstructure and Oxidation Behaviour of Euro IV Diesel Engine Soot: A Comparative Study with Synthetic Model Soot Substances . Catal. Today , 90 : 127 – 132 .
- Su , D. S. , Müller , J. O. , Jentoft , R. E. , Rothe , D. , Jacob , E. and Schlögl , R. 2004b . Fullerene-like Soot from EuroIV Diesel Engine: Consequences for Catalytic Automotive Pollution Control . Top. Catal. , 30/31 : 241 – 245 .
- Sydbom , A. , Blomberg , A. , Parnia , S. , Stenfors , N. , Sandstrom , T. and Dahlen , S. E. 2001 . Health Effects of Diesel Exhaust Emissions . Eur. Respir. J. , 17 : 733 – 746 .
- Sze , S.-K. , Siddique , N. , Sloan , J. J. and Escribano , R. 2001 . Raman Spectroscopic Characterization of Carbonaceous Aerosols . Atmos. Environ. , 35 : 561 – 568 .
- Van Doorn , J. , Vuurman , M. A. , Tromp , P. J. J. , Stufkens , D. J. and Moulijn , J. A. 1990 . Correlation between Raman Spectroscopic Data and the Temperature-Programmed Oxidation Reactivity of Coals and Carbons . Fuel Process. Technol. , 24 : 407 – 413 .
- Vander Wal , R. L. , Tomasek , A. J. , Street , K. , Hull , D. R. and Thompson , W. K. 2004 . Carbon Nanostructure Examinated by Lattice Fringe Analysis of High-Resolution Transmission Electron Microscopy . Appl. Spectrosc. , 58 : 230 – 237 .